Design, Synthesis, Chemical and Biochemical Insights Into Novel Hybrid Spirooxindole-Based p53-MDM2 Inhibitors With Potential Bcl2 Signaling Attenuation
- 1Pharmaceutical Organic Chemistry Department, Faculty of Pharmacy, Suez Canal University, Ismailia, Egypt
- 2Department of Chemistry, Faculty of Science, Alexandria University, Alexandria, Egypt
- 3Department of Chemistry, Faculty of Science, Suez Canal University, Ismailia, Egypt
- 4Medical Biotechnology Department, Genetic Engineering and Biotechnology Research Institute, City of Scientific Research and Technological Applications (SRTA-City), Alexandria, Egypt
- 5Department of Pharmaceutical Chemistry, Faculty of Pharmacy, Alexandria University, Alexandria, Egypt
- 6H.E.J. Research Institute of Chemistry, International Center for Chemical and Biological Sciences, University of Karachi, Karachi, Pakistan
- 7Department of Drug Design, Groningen Research Institute of Pharmacy, University of Groningen, Groningen, Netherlands
- 8Department of Organic Chemistry, University of Valencia, Valencia, Spain
- 9Department of Chemistry, College of Science, King Saud University, Riyadh, Saudi Arabia
The tumor resistance to p53 activators posed a clinical challenge. Combination studies disclosed that concomitant administration of Bcl2 inhibitors can sensitize the tumor cells and induce apoptosis. In this study, we utilized a rapid synthetic route to synthesize two novel hybrid spirooxindole-based p53-MDM2 inhibitors endowed with Bcl2 signaling attenuation. The adducts mimic the thematic features of the chemically stable potent spiro [3H-indole-3,2′-pyrrolidin]-2(1H)-ones p53-MDM2 inhibitors, while installing a pyrrole ring via a carbonyl spacer inspired by the natural marine or synthetic products that efficiently inhibit Bcl2 family functions. A chemical insight into the two synthesized spirooxindoles including single crystal x-ray diffraction analysis unambiguously confirmed their structures. The synthesized spirooxindoles 2a and 2b were preliminarily tested for cytotoxic activities against normal cells, MDA-MB 231, HepG-2, and Caco-2 via MTT assay. 2b was superior to 5-fluorouracil. Mechanistically, 2b induced apoptosis-dependent anticancer effect (43%) higher than that of 5-fluorouracil (34.95%) in three studied cancer cell lines, activated p53 (47%), downregulated the Bcl2 gene (1.25-fold), and upregulated p21 (2-fold) in the treated cancer cells. Docking simulations declared the possible binding modes of the synthesized compounds within MDM2.
Introduction
The World Health Organization declared in its 2020 report the burden posed by cancer worldwide. Two years ago, the estimated annual cancer cases were 18.1 million including 9.6 million deaths. These figures are expected to double by 2040 (WHO report on cancer). This is mirrored by the scientific community efforts to establish novel drug discovery protocols (Reed, 1999; Wong, 2011). Within this approach, evasion of apoptosis being a hallmark in carcinogenesis has attracted considerable interest (Pistritto et al., 2016). Given the pivotal regulatory role of the tumor suppressor protein p53 in apoptosis (Fridman and Lowe, 2003; Vazquez et al., 2008), drugging this pathway for harnessing its apoptosis-inducing functions is a rapidly growing efficient anticancer protocol (Khoo et al., 2014). p53, the so-called “guardian of the genome,” is a transcription factor that can regulate a plethora of genes controlling DNA repair, cell cycle arrest, and apoptosis (Fridman and Lowe, 2003; Vazquez et al., 2008). p53 normally induces the apoptotic cascade mainly via activating the pro-apoptotic proteins PMAIP1 and PUMA (Villunger et al., 2003), which are able to inhibit the mitochondrial antiapoptotic protein family such as Mcl1 and Bcl2 (Chen et al., 2005). Besides, the direct translocation of p53 to the mitochondria can induce Bcl2 family proteins and, consequently, trigger apoptosis (Mihara et al., 2003; Chipuk et al., 2004). Other findings implied that p53 can induce both caspase-dependent (Schuler et al., 2000) and -independent programmed cell death (Tovar et al., 2013) as well as cell senescence via the cell cycle inhibitor p21 (Lujambio et al., 2013). The transcription activity of p53 is tightly controlled by its endogenous negative regulator MDM2, which can directly conceal p53 N-terminal transactivation domain (Oliner et al., 1993) and induce its proteosomal degradation (Haupt et al., 1997). Consistent with its regulatory role, MDM2 is oncogenic when overexpressed (Ganguli et al., 2000).
In approximately 50% of human cancers, p53 is detected with mutation or deletion, whereas wild-type p53 cancers loss p53 functions due to overexpression (Eymin et al., 2002) or amplification (Oliner et al., 1992) of MDM2. Thus, targeting the interplay between p53 and MDM2 for harnessing p53 apoptosis-inducing functions has been adopted as attractive anticancer strategy. Over the last decades, active drug discovery programs have focused on validating p53–MDM2 pathway druggability. Various strategies, especially direct MDM2 inhibition, have been proposed by both academic research groups and industry (Anifowose et al., 2020; Konopleva et al., 2020). In the course of the initial hit finding, about 20 different classes of potent small-molecule MDM2 inhibitors have been introduced. Further optimization strategies disclosed about seven clinical-stage molecules (Popowicz et al., 2011; Beloglazkina et al., 2020). Of the most promising ones, for which both potency and pharmacokinetics could be optimized, are spirooxindoles.
Spirooxindoles were first identified via structure-based de novo design as MDM2–p53 interaction inhibitors by Wang et al. (Ding et al., 2006), where the p53 Trp23 indole ring that forms a key hydrogen bond with MDM2 Leu54 and fills its hydrophobic cleft was replaced by an oxindole ring. Then additional valency utilized the oxindole C3 to install a spiro-ring inspired by natural anticancer architectures such as spirotryprostatin B. The spiro [3H-indole-3,3′-pyrrolidin]-2(1H)-ones were then introduced with suitable vectors to address the two remaining lipophilic MDM2 cavities (Leu26 and Phe19) of the p53 binding site. Consequently, several clinical-stage spirooxindoles were developed such as SAR405838 by Sanofi-Aventis (Wang et al., 2014), RO2468 by Hoffmann-La Roche (Zhang et al., 2014), and DS-3032b by Daiichi Sankyo (Nakamaru et al., 2015). However, the chirality at C2 was with epimerization in solution by reversible ring-opening retro Mannich reaction (Popowicz et al., 2010). This associated chemical instability directed modifications of Wang’s scaffold to the more chemically stable spiro [3H-indole-3,2′-pyrrolidin]-2(1H)-ones that are not prone to epimerization. With more structure-based optimization studies inspired by natural products, a fused ring system that ideally suited MDM2 was incorporated. Promising derivatives were highly selective and orally active with in vivo efficacy even after single-dose administration as demonstrated by BI-0252 (Figure 1) (Gollner et al., 2016).
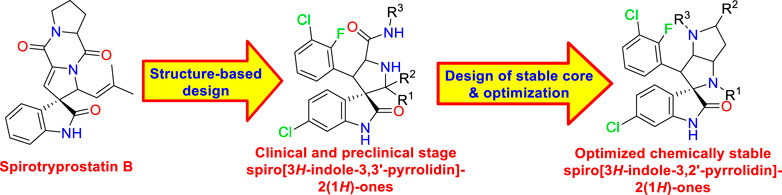
FIGURE 1. Design of spiro [3H-indole-3-3′-pyrrolidin]-2(1H)-one MDM2 inhibitors and optimization to the chemically stable spiro [3H-indole-3-2′-pyrrolidin]-2(1H)-ones series.
Despite the introduction of several efficient MDM2 inhibitors, various molecular mechanisms influencing cancer cell resistance to MDM2 inhibitors were reported (Long et al., 2010; Vogelstein et al., 2013; Chapeau et al., 2017). Such resistance posed an argument about the tumor cell response to p53 activation events. Accordingly, chemotherapeutic agents that can synergize with MDM2 inhibitors, reverse resistance, and induce stronger p53 response were extensively studied. Combination studies revealed that downregulating the antiapoptotic genes, especially the Bcl2 family members, sensitizes the cancer cells to apoptosis and promotes tumor elimination (Kojima et al., 2006; Kracikova et al., 2013).
Considering the major clinical challenge represented by the tumor resistance to MDM2 inhibitors, it is reasonable that the next frontier in MDM2 research is to develop efficient inhibitors endowed with potential to modulate p53 downstream signals involved in tumor sensitization beside its intrinsic p53-activation capability. Within this context, targeting Bcl2 family attracted our interest given the evidence that inhibition of MDM2 and Bcl2 protein function synergistically induce apoptosis in cancer cells (Kojima et al., 2006; Kracikova et al., 2013).
Accordingly, in continuation to our previous work (Barakat et al., 2019; Islam et al., 2019; Islam et al., 2020), we utilized the chemically stable spiro [3H-indole-3,2′-pyrrolidin]-2(1H)-one scaffold as core for installing a pyrrole ring on the spiro ring via carbonyl spacer inspired by marinopyrrole; a natural marine product that disrupt Bcl2 family functions by multiple mechanisms ranging from direct inhibition to downregulation (Figure 2) (Doi et al., 2012; Wan et al., 2018). Other representative examples for pan-Bcl2 family inhibitors such as obatoclax (GX15-070) and sunitinib are supportive for our rational design study (Li et al., 2008). The spiro ring was modified as pyrrolidine and hexahydropyrrolo[1.2-c]thiazoline, together with the terminal aryl ring substitutions, to afford two new spirooxindoles via [3 + 2] cycloaddition (32CA) reaction of olefin-based N-methyl pyrrole with the substituted isatin, and the secondary amines Scheme 1. Recent advances made in the theoretical understanding of 32CA reactions based on the molecular electron density theory (Domingo, 2016) (MEDT) have allowed establishing a very good correlation between the electronic structure of the simplest three-atom- component (TAC) and their reactivity toward ethylene (Ríos-Gutiérrez and Domingo, 2019). The simplest AY, CH2–NH–CH2, is a very reactive pseudodiradical TAC participating in pdr-type 32CA reactions without any appreciable barrier (Domingo et al., 2010). However, the substitution on the experimental TACs stabilize them, thus, changing its electronic structure and, consequently, its reactivity to that of zw-type 32CA reactions (Domingo et al., 2020a). Consequently, this type of 32CA reaction demands the adequate nucleophilic/electrophilic activation of the reagents to take place (Ríos-Gutiérrez and Domingo, 2019).
The synthesized spirooxindoles were subjected to cytotoxicity screening via MTT assay (Mosmann, 1983; Rizk et al., 2019; Abdelmoneem et al., 2021) on normal lung fibroblasts (Wi-38) to assess their safety profiles, followed by evaluating their anticancer potencies against three selected human cancers: breast (MDA-MB 231), liver (HepG-2) and colon (Caco-2), which are reported to be among the most common leading causes of cancer death globally (WHO 2020). Flow cytometric analysis of apoptosis was then performed to test their apoptotic induction potentials, followed by immunohistochemical analysis of p53 transactivation and qRT-PCR analysis of Bcl2 gene expression. Further investigation of p53 downstream signaling status was performed via qRT-PCR analysis of p21 expression (Lujambio et al., 2013; Seidel et al., 2013). Finally, docking simulations of the studied spirooxindoles were conducted into MDM2 to get more information about their possible binding modes and the essential structural features.
Results and discussion
Chemistry
Synthesis
The starting material required for the synthesis of the substituted spirooxindole scaffold is the N-methyl pyrrole-based chalcone. The later chalcone 1a, b is synthesized by aldol condensation of N-methyl-2-acetylpyrrole with the appropriate aldehydes (3-fluorobenzaldehyde/3-nitrobenzaldehyde) in the presence of aqueous NaOH, by employing a multicomponent one-pot reaction approach to furnish the requisite compound in high purity as well as regioselective and diastereoselective fashion as drawn in Schemes 2, 3. The chemical features of the substituted spirooxindole analogs were assigned based on the spectrophotometric techniques. 1HNMR and 13CNMR show all the features of the corrected protons and carbon, respectively.
Crystal structures of compounds 2a, b
The asymmetric unit of 2a is found to be comprised of two molecules (Figure 3A and Supplementary Table S1). Structurally, compound 2a consists of five-membered N-methyl pyrrole (N20/C21/C16–C19) ring linked with spiroindole (C1–C7/C12–C14/C24–C25/Cl1/N1–N2/O3) moiety via the carbonyl group at C6 atom. The fluoro-substituted benzene (F1/C8–C11/C22–C23) ring was also found to be attached with spiroindole moiety at the C32 atom. One of the molecules in asymmetric unit showed 50% fluoride occupation on two carbon atoms (C27 and C48) of the ring. In the crystal lattice of compound 2a, molecules are found to be connected via H2A … O3, H3A … N1, H3 … O4, H12 … O4, H15 … O4, H24 … F2, and H38 … N4 intermolecular interactions to form a three-dimensional (3D) network with donor acceptor distance of 2.9046, 3.0784, 3.2510, 3.3412, 3.4922, 3.4083, and 3.4292 Å, respectively (Figure 4 and Supplementary Table S2).
Compound 2b crystals out as methanol solvate and was found to consist of a five-membered N-methyl pyrrole (N2/C16–C20) ring linked with spiroindole (C5–C11/C23–C26/Cl1/N3–N4/O4) moiety via carbonyl group at C6 atom. The spiroindole moiety was found to be further linked with S1/C21–C22 atoms forming a five-membered (N3/S1/C21–C23) ring with boat conformation. The nitro-substituted benzene (N11/O1–O2/C2–C4/C12–C14) ring was also found to be attached with spiroindole moiety at the C5 atom. The asymmetric unit also contains one methanol solvent (C27/O5) molecule (Figure 3B and Supplementary Table S1). In the three-dimensional network of crystal lattice of compound 2b, molecules are found to be connected via H4A … O5, H1 … O5, H11 … O2, and H19 … O4 intermolecular interactions with donor acceptor distances of 2.8199, 3.4252, 3.047, and 3.2463 Å, respectively (Figure 4 and Supplementary Table S3).
Analysis of molecular packing
The different contacts observed in the crystal structure of 2b are shown in Supplementary Figure S9. The molecules are mainly packed by H … H (36.6%), O … H (23.7%), C … H (13.6%), Cl … H (9.1%), and S … H (5.6%) and N … H (3.1%) contacts. The Hirshfeld surfaces for the intermolecular interactions with contact distances shorter than van der Waals radii sum of the two elements sharing this contact are shown in Figure 5 and corresponding interaction distances are listed in Table 1. The strongest contacts are those that appeared as red spots, which correspond to O … H, Cl … H and S … H hydrogen bonds as well as C–H … π interactions.
For 2a, the asymmetric unit comprised two molecules of this compound. One of them showed substitutional disorder at the F2 and F3 atomic sites with H17 and H48, respectively, and with equal occupancies. As a result, we have the two possible relative orientations of the molecular units in the crystal as shown in Figure 6. Presentation of all possible contacts and their percentages for the four possible in this crystal structure are shown in Supplementary Figure S10.
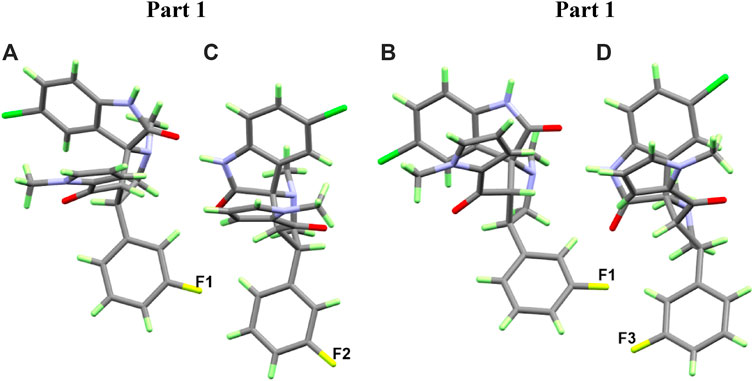
FIGURE 6. The possible orientation of the two molecular units as a consequence of the substitutional disorder in 2a.
In this regard, we have four sets of results for this compound as shown in Supplementary Figure S10. The most important contacts in 2a are H … H (42.2–46.4%), C … H (16.1–19.8%), F … H (6–10.9%), Cl … H (8.1–12.1%), and O … H (8.0–11.6%). The Hirshfeld analysis of the shortest contacts observed in case of 2a is collected in Figure 7 and a list of these interactions, along with the contact distances, is given in Table 2. It is worth noting that some short halogen-bonding interaction with contact distance of 3.042 Å for Cl1 … O5 contact was noted in this compound. It is important to mention that the rotation of the fluorophenyl ring around the C–C bond, which connects it with the spiro system leads to some weak F … F contacts only found in part 2 of the disordered system. The percentage of the F … F interactions does not exceed 1.1% from the whole intermolecular contacts.
DFT structural studies
Based on the x-ray structure model, compound 2a could exist in two possible conformers as shown in Supplementary Figure S13. The optimized molecular geometries of both conformers were calculated and presented in comparison with the x-ray structure model in Supplementary Figure S13. The optimized and calculated bond distances are in good agreement (Supplementary Table S4; Supplementary Data). There are good correlations between the calculated and experimental bond distances for both conformers (Supplementary Figure S14). The correlation coefficients are 0.9863 and 0.9916, respectively. It is worth to note that the total energies of 2a_F1 and 2a_F2 are −1,130,708.937 and −1,130,711.000 eV, respectively, with an energy difference of 2.063 eV. The energy difference is very small, which confirms the coexistence of both conformers as revealed from the x-ray structure analysis. Also, the dipole moments of the two conformers are close to each other. The calculated dipole moments values are 4.1294 and 4.6453 Debye for 2a_F1 and 2a_F2, respectively.
On the other hand, the optimized molecular geometries of 2b with and without the crystallized methanol molecule are given in Supplementary Figure S15. Also, a comparison between the calculated and optimized geometries is presented in the lower part of this figure together with the correlations between the calculated and experimental bond distance for both molecules. The correlations coefficients are high (0.9951–0.9955) indicating the good agreement between the calculated and x-ray structures (Supplementary Table S5; Supplementary Data). The calculated dipole moment is predicted to be higher in presence of the crystallized solvent (6.9043 Debye) compared with 5.8779 Debye for the compound without the crystallized methanol molecule.
The molecular electrostatic potential (MEP) map is a colored presentation that indicates the different charged regions in molecular systems. Red-colored regions are the most negative, while the most positive regions have a blue color (Figure 8). These regions represent the most proper regions for hydrogen-bonding interactions as hydrogen bond acceptor and hydrogen bond donor, respectively. The red regions are related to the oxygen atoms in both compounds which represent the most suitable regions for hydrogen bonding interactions as hydrogen bond acceptor sites. In contrast, the blue regions are related to the NH proton, which acts as a hydrogen bond donor. These results are in good agreement with the x-ray structure of the studied compounds.
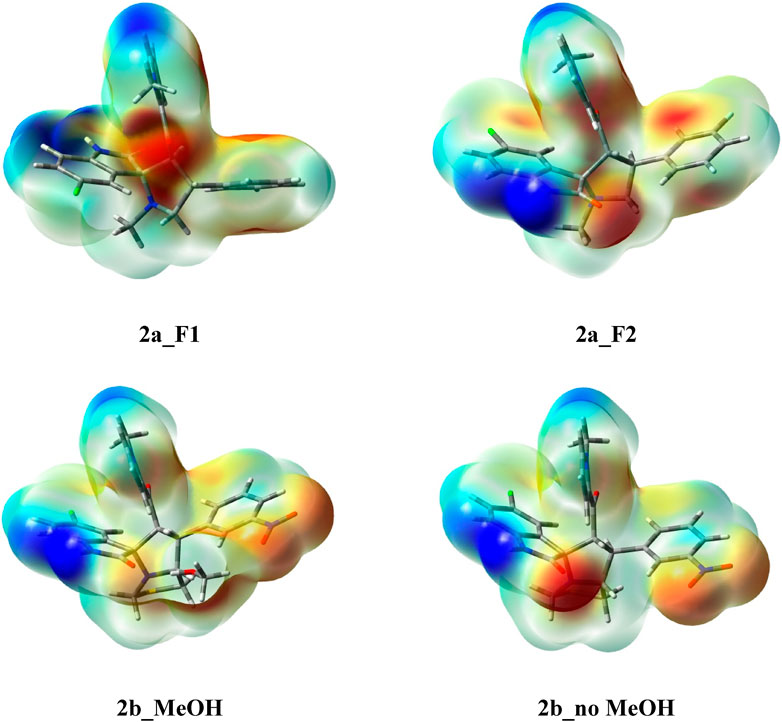
FIGURE 8. The molecular electrostatic potential (MEP) maps of the studied compounds. The color index from blue to turquoise to yellow to red indicat the more negative electron density.
The natural charges at the different atomic sites are listed in Supplementary Table S5; (Supplementary Data). In 2a, the most negative atomic sites are the oxygen atoms of the C=O groups and the nitrogen sites of the spiro-system. The calculated charges are in the range of −0.597 to −0.616 and −0.512 to −0.611, respectively. On other hand, the most positive atomic sites are the NH proton and the carbonyl carbon atoms with natural charges ranging from 0.408 to 0.410 e and 0.541 to 0.727 e, respectively. Similarly, for 2b, the two carbonyl oxygen atoms are the most negative with natural charges ranging from 0.598 to −0.641 e while the corresponding carbon atoms and the NH proton are the most positive sites with natural charges in the range of 0.536–0.742 and 0.410–0.412 e, respectively. In addition, the nitrogen atom of the nitro group has also a high positive charge of 0.517–0.519 e.
Conceptual DFT analysis of the 32CA reactions of AYs 3 and 4 with ethylene derivatives 1a and 1b
The reactivity indices defined within the conceptual DFT (CDFT) (Parr and Yang, 1989; Domingo et al., 2016) have shown to be powerful tools to understand the reactivity in polar reactions. The global reactivity indices, namely, the electronic chemical potential μ, chemical hardness η, global electrophilicity ω, and global nucleophilicity N, for the reagents involved in these 32CA reactions are gathered in Table 3.
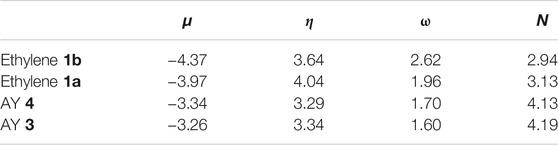
TABLE 3. B3LYP/6-31G(d) reactivity indices, in eV, of AYs 3 and 4, and ethylene derivatives 1a and 1b.
The electronic chemical potentials (Parr and Yang, 1989) μ of AYs, −3.26 (3) and −3.34 (4) eV, are higher than those of the ethylene derivatives, −3.97 (1a) and −4.37 (1b) eV, indicating that along a polar 32CA reaction, the global electron density transfer (GEDT) (Domingo, 2014) will take place from these AYs to the ethylene derivatives, the reactions being classified as the forward electron density flux (FEDF) (Domingo et al., 2020b).
AYs 3 and 4 have an electrophilicity ω index (Parr et al., 1999) of 1.60 (3) and 1.70 (4) eV, respectively, being classified as strong electrophiles within the electrophilicity scale (Domingo et al., 2016). On the other hand, they have a nucleophilicity N index (Domingo et al., 2008a) of 4.19 (3) and 4.13 (4) eV, being classified as strong nucleophiles within the electrophilicity scale (Domingo et al., 2016). The strong nucleophilic character of these AYs, higher than 4.0 eV, allows their classifications as a supernucleophile (Chamorro et al., 2020).
Ethylene derivatives 1a and 1b have an electrophilicity ω index of 1.96 (1a) and 2.62 (1b) eV, respectively, being classified as strong electrophiles. On the other hand, they have a nucleophilicity N index of 3.13 (1a) and 2.94 (1b) eV, being classified as a strong nucleophile and in the borderline of moderate nucleophiles, respectively. The presence of a strong electron-withdrawing NO2 group in ethylene 1b increases the electrophilicity and decreases the nucleophilicity of this species with respect to those of ethylene 1a. The supernucleophilic character of AYs 3 and 4 together with the strong electrophilic character of ethylenes 1a and 1b indicate that the corresponding 32CA reactions will have polar character, being classified as FEDF (Domingo et al., 2020b).
Study of the reaction mechanism
The mechanism of the 32CA reaction of AY 3 with ethylene 1a was theoretically studied. Due to the nonsymmetry of both reagents, two pairs of endo and exo stereoisomeric and two pairs of ortho and meta regioisomeric reaction paths are feasible. The four competitive reaction paths were studied (see Scheme 4). Analysis of the stationary points found in these reaction paths indicates that this 32CA reaction takes place though a one-step mechanism. The ωB97X-D/6-311G(d,p) relative energies in methanol are given in Scheme 4.
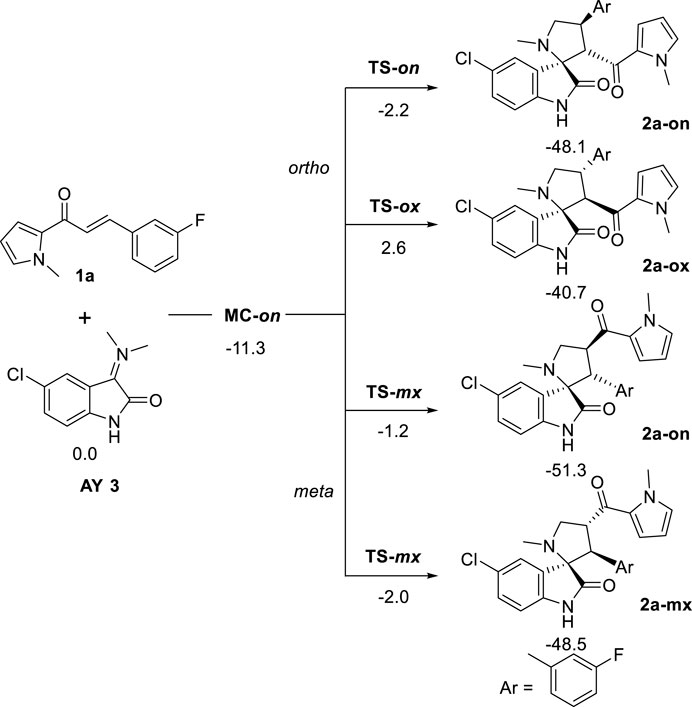
SCHEME 4. 32CA reaction of AY 3 with the ethylene derivative 1a. ωB97X-D/6-311G(d,p) relative energies in methanol, with respect to separated reagents, are given in kcal·mol−1
A series of molecular complexes (MCs) in which the two reagents are already joined by weak intermolecular interactions were also found. Only the most stable of them, MC-on, was selected as the energy reference. The distance between the two frameworks at this MC is ca. 3.2 Å; MC-on is found 11.3 kcal·mol−1 below the separated reagents (see Scheme 4). The most favorable TS-on is found 2.2 kcal·mol−1 below the separated reagents, the corresponding reaction path being strongly exothermic by 48.1 kcal·mol−1. Some appealing conclusions can be obtained from the relative energies given in Scheme 4: 1) TS-on is found below the separated reagents, but if the formation of MC-on is considered, the activation energy becomes positive by 9.1 kcal·mol−1. 2) The regioisomeric TS-mx is found only 0.2 kcal·mol−1 above TS-on. These energy results do not account for the only formation of 2-on via TS-on. 3) This 32CA reaction is totally endo stereoselective as TS-ox is found 4.8 kcal·mol−1 above TS-on. 4) The high exothermic character of the formation of 2a-on, −48.1 kcal mol−1, makes this 32CA reaction irreversible. Consequently, cycloadduct 2a-on is formed by kinetic control.
The geometries of the four TSs are given in Figure 9. The C−C distances between the four interacting carbons at the four TSs indicates that they correspond with asynchronous C−C single bond formation processes, in which the shorter C−C distance corresponds to that involving the methylene CH2 carbon of AY 3. At the most favorable TS-on, the C−C distances between the two pairs of interacting carbons, 2.110 and 2.685 Å, indicate that this TS is associated with a high asynchronous C−C single bond formation process, in which the shorter C−C distance corresponds to that involving also the most electrophilic ß-conjugated carbon of the ethylene derivative 1a. Analysis of the intrinsic reaction coordinates (Fukui, 1970) associated to high asynchronous TS-on indicates that this 32CA reaction takes place through a nonconcerted two-stage one-step mechanism (Domingo et al., 2008b) in which the formation of the second C−C single bond begins when the first C−C single bond is completely formed.
Finally, analysis of GEDT (Domingo, 2014) at TSs permits assessment of the polar character of this 32CA reaction. GEDT values lower than 0.05 e correspond with nonpolar processes, while values higher than 0.20 e correspond with polar processes. The GEDT values at the TSs are 0.19 e (TS-on), 0.13 e (TS-oxn), 0.21 e (TS-mn), and 0.22 e (TS-mx). These values, which are a consequence of the supernucleophilic character of AY 3 and the strong nucleophilic character of ethylene 1a, indicate that this 32CA reaction has a polar character. The flux of the electron density, which goes form AY 3 to ethylene 1a, classifies this 32CA reaction as FEDF, in clear agreement with the analysis of the CDFT indices.
Biological evaluation
Cytotoxicity screening
The studied spirooxindoles (2a and 2b) were screened for possible cytotoxic activities on normal lung fibroblasts (Wi-38) for assessment of their safety profiles. Then they were evaluated for their anticancer potential against three selected cancer cell lines (MDA-MB 231, HepG-2, and Caco-2) compared with 5-fluorouracil (5-FU) via MTT assay (Table 4) (Mosmann, 1983; Rizk et al., 2019; Abdelmoneem et al., 2021). Compound 2b was superior to 5-FU and 2a against the screened cells within its safe dose (EC100). Obviously, it recorded single-digit nanomolar IC50 values against MDA-MB 231 and Caco-2 cells. These pronounced anticancer activities were observed as severe shrinkage of the treated cancer cells (Figure 10) (Jerabek-Willemsen et al., 2011; Seidel et al., 2013). Compound 2a was also more potent than 5-FU against MDA-MB 231; however, it exhibited its anticancer effect beyond its respective EC100; hence, it lacked considerable selectivity. Unfortunately, it lacked potency HepG-2 and Caco-2 cells.
Flow cytometric annexin V/propidium iodide analysis of apoptosis
The studied derivatives were evaluated for their apoptotic induction potential by flow cytometric analysis (Seidel et al., 2013). As illustrated (Figure 11; Table 5), 2b demonstrated the highest apoptosis-dependent anticancer activity (>39%) in MDA-MB 231, HepG-2, and Caco-2 cells. The apoptotic effect of 2b was higher than that of the reference drug (13.02%–34.95%) in three studied cancer cell lines. Meanwhile, 2a recorded relatively lower apoptotic population percentages than 2b and 5-FU in HepG-2 and Caco-2 cells (Table 5). In MDA-MB 231 cells, 2a exhibited significantly higher apoptotic activity than 5-FU. The results were consistent with that of the MTT assay.
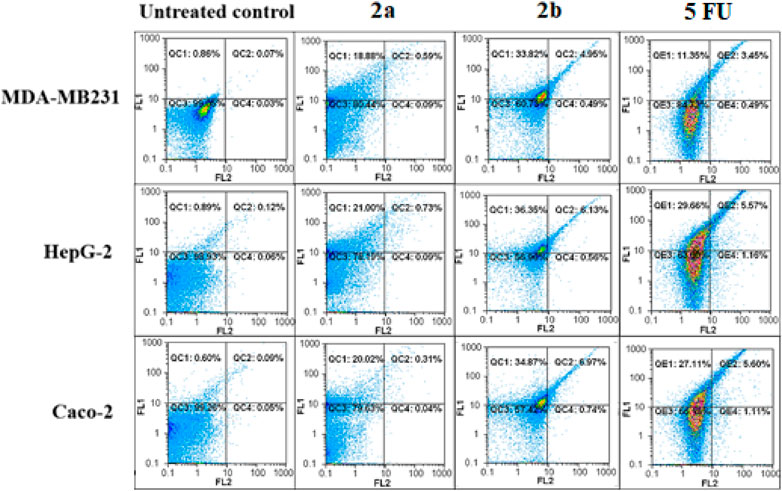
FIGURE 11. Flow charts of annexin-PI analysis of 2a- and 2b-treated MDA-MB 231, HepG-2, and Caco-2 cells compared with 5-fluorouracil (5-FU)-treated cells.
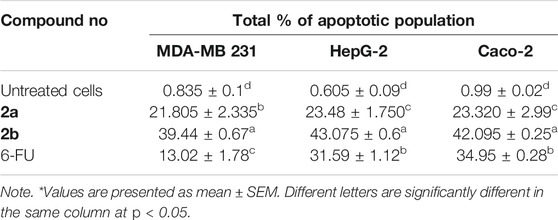
TABLE 5. Apoptotic cell population percentages in 2a- and 2b-treated MDA-MB 231, HepG-2, and Caco-2 cells.
Immunohistochemical analysis of p53 protein overexpression
We analyzed p53 immunoreactivity in HepG-2 cells treated with the two studied spirooxindoles, 2a and 2b. Interestingly, after exposure of HepG-2 cells to 2a and 2b for 72 h, a significant increase was detected in p53-positive cells. Figure 12 shows that p53 transactivation in the 2b-treated HepG-2 cells (47.24%) was significantly higher than that in the 2a-treated ones (9.12%).
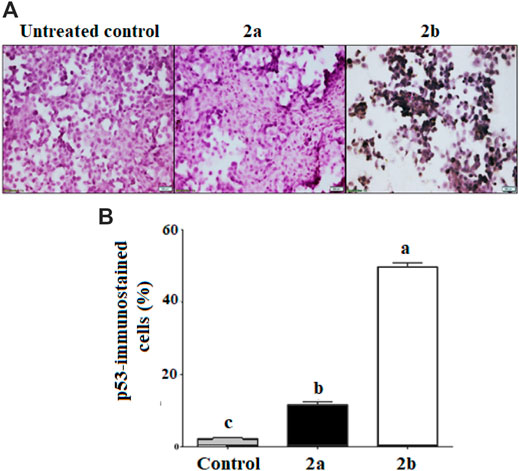
FIGURE 12. p53 immunohistochemical analysis of p53 expression in 2a- and 2b-treated HepG-2 cells. (A) Immunostaining images of p53-treated and untreated cells (magnification ×400) with (B) representative percentages of the positive immunostaining HepG-2 cells. Different letters are significantly different at p < 0.05.
qRT-PCR analysis of Bcl2 gene expression
RT-PCR analysis of Bcl2 gene in HepG-2 cells after treatment with the studied spirooxindoles revealed a 1.25-fold downregulation relative to the untreated cells (Figure 13). Both compounds were nearly equipotent.
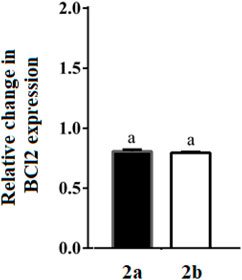
FIGURE 13. Change in gene expression of Bcl2 in the treated HepG-2 cells. Different letters are significantly different at p < 0.05.
qRT-PCR analysis of p21 gene expression
P53 mediates its DNA damage-induced checkpoint via transactivation of a plethora of apoptotic genes. Of these, p21 mediates G1 growth arrest (Alexander et al., 2014). Given its main p53-dependent antiproliferative role, p21 gene expression level was quantified by qRT-PCR in HepG-2 cells treated with the studied compounds (Figure 14). Herein, 2b upregulated p21 expression by two-folds, whereas, 2a recorded a 1.22-fold increase. This observation may be subsequent to p53-transactivation by the studied spirooxindoles.
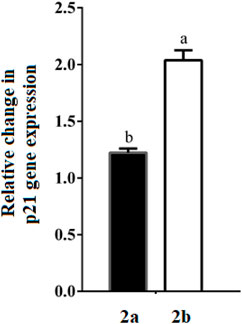
FIGURE 14. Change in gene expression of p21 in the treated HepG-2 cells. Different letters are significantly different at p < 0.05.
Molecular modeling
Docking simulations
The coordinates of MDM2 co-crystallized with the spiro [3H-indole-3,2′-pyrrolidin]-2(1H)-one inhibitor 6SJ were downloaded from the protein data bank (PBD ID: 5LAW) (Gollner et al., 2016) and prepared by MOE 2016.0802 “QuickPrep” module (Muegge et al., 2011). The studied spirooxindoles 2a and 2b were built, energy minimized, then docked into the binding site of the inhibitor. The best binding modes (Figure 15) showed that the indolinone ring of both compounds were buried into the Trp23(p53) pocket. However, only 2a formed the key hydrogen bond interactions with the backbone Leu54(MDM2) as the reference inhibitor 6SJ. Interestingly, the nitro group of 2b offered hydrogen bonding interactions with His96(MDM2) in the Leu26(p53) pocket and Lys94(MDM2). In light of these results, it may be assumed that the studied derivatives can share some key interactions with the reference MDM2 inhibitor.
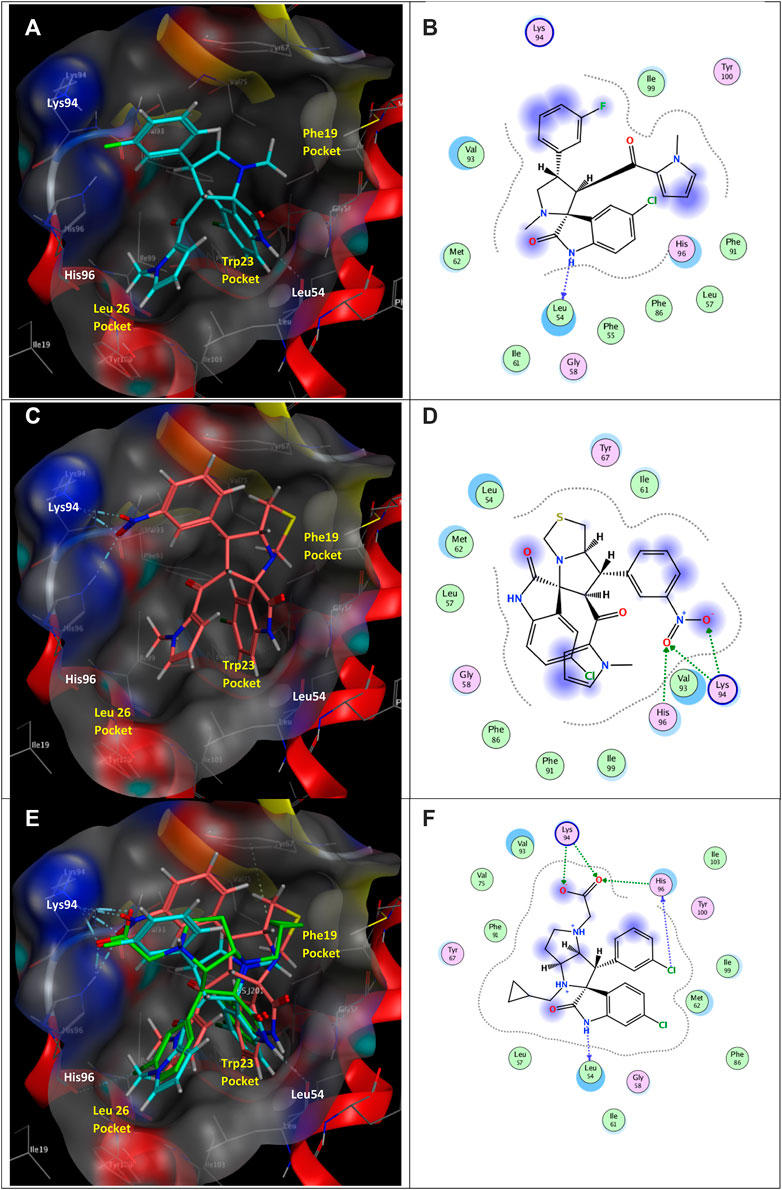
FIGURE 15. (A) Three-dimensional (3D) binding mode of 2a (cyan sticks), (B) 2D binding mode of 2a, (C) 3D binding mode of 2b (dark pink sticks), (D) 2D binding mode of 2b, (E) overlay of 2a and 2b with the co-crystalized human homolog of mouse double minute 2 (MDM2) inhibitor (green sticks), (F) 2D binding mode of the co-crystalized ligand 6SJ in MDM2 (PBD ID: 5LAW) (Gollner et al., 2016). The Leu26, Trp23, and Phe19 pockets of p53 are indicated as well as the key hydrogen bond interactions with MDM2 Leu54.
In silico Prediction of Physicochemical Properties, ADME and Drug-Likeness
Physicochemical properties, ADME, and drug-likeness parameters are extensively employed as lead identification tools. Herein, SwissADME (Daina et al., 2017) was utilized to predict the important physicochemical properties formulating the drug-likeness parameters of the studied spirooxindoles 2a and 2b (Table 6). Both compounds recorded drug-like bioavailability according to the parameters of Lipinski (Lipinski et al., 2017), Veber (Veber et al., 2002), and Muegge (Muegge et al., 2011). Compound 2a showed full accordance, whereas 2b exhibited only single Lipiniski violation regarding its molecular weight. PreADMET 2020 (https://preadmet.bmdrc.kr/adme/) (accessed August 14, 2020) was also employed for ADME prediction. The studied compounds displayed acceptable predicted aqueous solubility; however, 2a recorded relatively better solubility than 2b. Both were predicted to possess excellent intestinal absorption (>96%). Compound 2a displayed medium BBB penetration, whereas 2b was poorly absorbed by the CNS, thus predicted to be devoid of CNS-related side effects. Both recorded moderate predicted permeability through the Caco-2 cells model. Compound 2a was moderately absorbed by MDCK, unlike the relatively poorly absorbed 2b. The predicted plasma protein binding profile of 2b was almost 100%. Compound 2b recorded medium plasma protein binding (88%), thus it was expected to be more available for transport across various membranes than 2a. Both compounds were predicted to inhibit cytochromes CYP3A4 and CYP2D6. They showed no detected PAINs indicating genuine activities.
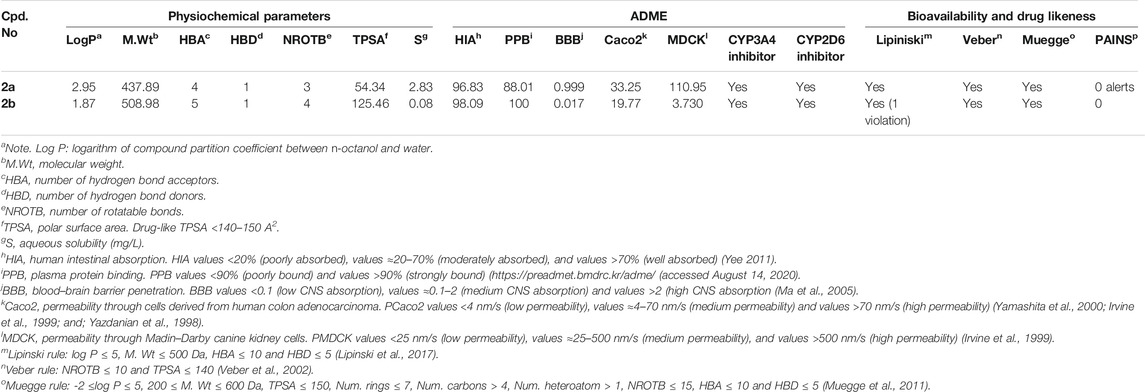
TABLE 6. In silico predicted physicochemical properties, ADMET, and drug likeness of the spirooxindoline derivatives 2a and 2b.
Conclusion
The current study portrays the design, synthesis, characterization, mechanistic study of 32CA reaction and biological evaluation of novel spirooxindole derivatives as dual MDM2 and Bcl2 inhibitors. The supramolecular structure of the studied compound is analyzed using Hirshfeld calculations. The calculated molecular geometry of the studied compound agrees well with the experimental x-ray structure. Also, calculated NMR spectra are in good agreement with the experimental data. Different electronic and reactivity descriptors were calculated and discussed. MTT assay revealed promising anticancer potencies, especially 2b that was superior to 5-FU against the screened cells with its safe dose. Compound 2b induced apoptosis-dependent anticancer activity up to 43.08% (superior to 5-FU), activated p53 by up to 47%, downregulated Bcl2 gene by 1.25 fold, and upregulated p21 by two folds in the treated cancer cells. Docking simulations of the synthesized compounds within MDM2 predicted common key interactions between the synthesized derivatives and the reference inhibitor. In light of the aforementioned data, we hope that further research in this area may allow introduction of the next-generation clinical-stage p53-MDM2 inhibitors in the near future.
Experimental
Materials and equipment
1H-NMR and 13C-NMR spectra of spiroxindole analogs 2a, b were recorded on CDCl3 using a JEOL 400 MHz spectrometer (JEOL Ltd., Tokyo, Japan) at room temperature. Mass spectra were recorded on JMS-600 H JEOL spectrometer (JEOL Ltd., Tokyo, Japan). X-Ray crystallographic analysis was collected by using a Bruker SMART APEX II D8 Venture diffractometer at Karachi University. Note for refractive index (specific rotation) measurement: The samples were prepared in 10 ml, then the concentration was calculated in g/100 ml, and a 100-mm polarimeter tube was used. The instrument used was A.KRÜSS Optronic P8000-PT digital polarimeter.
(E)-3-(3-Fluorophenyl)-1-(1-methyl-1H-pyrrol-2-yl)prop-2-en-1-one 1a and (E)-1-(1-methyl-1H-pyrrol-2-yl)-3-(3-nitrophenyl)prop-2-en-1-one 1b were synthesized according to the literature, and the spectrum is in good agreement with the reported (Yenupuri, et al., 2014).
Synthesis of the spiroxindole derivatives 2a, b
A mixture of N-methyl pyrrole-based chalcone 1a, b (0.5 mmol), 5-Clisatin (0.5 mmol), and the appropriate secondary amine sarcosine/thioproline (0.5 mmol) in methanol (10 ml) was refluxed for 3 h. After completion of the reaction as evident from tlc, the final product was precipitated, and the resulting solid was filtered and recrystallized from DCM/ethanol to afford a pure product.
(3S,3′R,4′S)-5-Chloro-4′-(3-fluorophenyl)-1′-methyl-3′-(1-methyl-1H-pyrrole-2-carbonyl)spiro [indoline-3,2′-pyrrolidin]-2-one 2a
Rf = 0.5; eluent: (50% ethyl acetate: hexane); m. p.: 203°C; 1H-NMR (CDCl3, 400 MHz): δ 7.83 (S, NH, 1H), 7.25–7.18 (m, aromatic-H, 4 H), 7.04 (dd, J = 8.08, 2.16 Hz, pyrrole-H), 6.91 (m, Ph-H, 1H), 6.60 (s, Ph-H, 1H), 6.54 (d, J = 8.28 Hz, pyrrole-H, 2H), 5.83 (t, J = 2.08 Hz, Pyrrole-H, 1H), 4.46–4.39 (m, pyrrolidine-H, 1H), 4.18 (d, J = 9.56 Hz, pyrrolidine-H, 1H), 3.60–3.55 (t overlaid s, pyrrolidine-H, and methyl, 4H), 3.44–3.40 (t, J = 7.28 Hz, pyrrolidine-H, 1H), 2.23 (s, methyl, 3H); 13C-NMR (CDCl3, 400 MHz): δ 186.0 (CO), 179.8(CO), 164.3, 161.8, 143.8, 139.2, 131.7, 129.6, 129.0, 127.9, 127.0, 123.9, 118.8, 115.0, 113.9, 11.7, 110.1, 107.9, 74.2, 63.1, 60.2, 43.7, 37.1, 35.1; HRMS (EI) calcd for C24H21ClFN3O2 (M+): 437.1300. Found: 437.1311;
(3S,6′R,7′S)-5-Chloro-6′-(1-methyl-1H-pyrrole-2-carbonyl)-7′-(3-nitrophenyl)-1′,6′,7′,7a′-tetrahydro-3′H-spiro [indoline-3,5′-pyrrolo [1,2-c]thiazol]-2-one 2b
Rf = 0.25; eluent: (50% ethyl acetate: hexane); m.p.: 130°C; 1H-NMR (CDCl3, 400 MHz): δ 8.52 (S, NH, 1H), 8.50 (s, Ph-H, 1H), 8.11 (d, J = 8.00 Hz, Ph-H, 1H), 7.86 (d, J = 8.00 Hz, Ph-H, 1H), 7.66 (d, 2.02 Hz, Pyrrole-H, 1H), 7.51 (t, J = 8.04 Hz, Ph-H, 1H), 7.19 (dd, J = 8.04, 2.02 Hz, pyrrole-H, 1H), 6.79 (dd, J = 4.36, 1.36 Hz, Ph-H, 1H), 6.68 (dd, J = 8.02, 1.88 Hz, 2.16 Hz, Ph-H, 1H), 6.56 (t, J = 2.02 Hz, Ph-H, 1H), 5.88 (t, J = 3.36 Hz, pyrrole-H, 1H), 4.46 (d, J = 11.64 Hz, pyrrolidine-H, 1H), 4.36–4.33 (m, pyrrolidine-H, 1H), 4.05 (d, J = 11.76 Hz, pyrrolidine-H, 1H), 3.90 (d, J = 10.32 Hz, pyrrolidine-H, 1H), 3.53 (d, J = 10.52 Hz, pyrrolidine-H, 1H), 3.53 (s, thiazolidine-CH2, 2H), 3.33 (s, methyl, 3H), 3.09 (dd, J = 11.72, 5.96 Hz, thiazolidine-H, 1H), 3.00 (dd, J = 11.76, 2.60 Hz, pyrrolidine-H, 1H), 13C-NMR (CDCl3, 400 MHz): δ 183.7, 180.1, 148.7, 141.3, 139.2, 135.0, 132.1, 130.4, 130.0, 129.0, 128.9, 127.8, 125.2, 123.2, 122.6, 120.0, 110.8, 108.2, 75.1, 74.4, 63.2, 54.5, 50.9, 50.3, 36.8, 36.4; (HRMS (EI) calcd for C25H21ClN4O4S (M+): 508.1018. Found: 508.1075;
Single-crystal x-ray diffraction analysis
Single-crystal x-Ray diffraction analysis has been provided in supplementary information.
Computational methods and Hirshfeld surface analysis
Hirshfeld surface analysis and computational methods have been provided in the supplementary information.
Biological evaluation assays
Biological evaluation assays including cytotoxicity evaluation, anticancer evaluation, flow cytometric analysis of apoptosis, immunohistochemical detection of tumor suppressor protein (p53), qRT-PCR analysis of p21 and Bcl2 gene expression, and statistical analysis, have been provided in the Supplementary Information.
Molecular docking protocol
Molecular docking protocol has been provided in the Supplementary Information.
Data Availability Statement
The datasets presented in this study can be found in online repositories. The names of the repository/repositories and accession number(s) can be found below: The Cambridge Crystallographic Data Centre (CCDC); CCDC numbers: 2012711 and 2012712.
Author Contributions
All authors listed have made a substantial, direct, and intellectual contribution to the work and approved it for publication.
Funding
The authors would like to extend their sincere appreciation to the Researchers Supporting Project Number (RSP-2021/64), King Saud University, Riyadh, Saudi Arabia, and project number PID2019-110776GB-I00 (AEI/FEDER, UE), Ministerio de Ciencias, Innovación y Universidades of the Spanish Government.
Conflict of Interest
The authors declare that the research was conducted in the absence of any commercial or financial relationships that could be construed as a potential conflict of interest.
Publisher’s Note
All claims expressed in this article are solely those of the authors and do not necessarily represent those of their affiliated organizations, or those of the publisher, the editors, and the reviewers. Any product that may be evaluated in this article, or claim that may be made by its manufacturer, is not guaranteed or endorsed by the publisher.
Supplementary Material
The Supplementary Material for this article can be found online at: https://www.frontiersin.org/articles/10.3389/fchem.2021.735236/full#supplementary-material
References
Abdelmoneem, M. A., Abd Elwakil, M. M., Khattab, S. N., Helmy, M. W., Bekhit, A. A., Abdulkader, M. A., et al. (2021). Lactoferrin-Dual Drug Nanoconjugate: Synergistic Anti-Tumor Efficacy of Docetaxel and the NF-κB Inhibitor Celastrol. Mater. Sci. Eng. C 118, 111422. doi:10.1016/j.msec.2020.111422
Alexander, C. G., Wanner, R., Johnson, C. M., Breitsprecher, D., Winter, G., Duhr, S., et al. (2014). Novel Microscale Approaches for Easy, Rapid Determination of Protein Stability in Academic and Commercial Settings. Biochim. Biophys. Acta (Bba) - Proteins Proteomics 1844 (12), 2241–2250. doi:10.1016/j.bbapap.2014.09.016
Anifowose, A., Agbowuro, A. A., Yang, X., and Wang, B. (2020). Anticancer Strategies by Upregulating P53 through Inhibition of its Ubiquitination by MDM2. Med. Chem. Res. 29, 1105–1121. doi:10.1007/s00044-020-02574-9
Barakat, A., Islam, M. S., Ghawas, H. M., Al-Majid, A. M., El-Senduny, F. F., Badria, F. A., et al. (2019). Design and Synthesis of New Substituted Spirooxindoles as Potential Inhibitors of the MDM2-P53 Interaction. Bioorg. Chem. 86, 598–608. doi:10.1016/j.bioorg.2019.01.053
Beloglazkina, A., Zyk, N., Majouga, A., and Beloglazkina, E. (2020). Recent Small-Molecule Inhibitors of the P53-MDM2 Protein-Protein Interaction. Molecules 25 (5), 1211. doi:10.3390/molecules25051211
Chamorro, E., Duque-Noreña, M., Gutierrez-Sánchez, N., Rincón, E., and Domingo, L. R. (2020). A Close Look to the Oxaphosphetane Formation along the Wittig Reaction: A [2+2] Cycloaddition?. J. Org. Chem. 85, 6675–6686. doi:10.1021/acs.joc.0c00697
Chapeau, E. A., Gembarska, A., Durand, E. Y., Mandon, E., Estadieu, C., Romanet, V., et al. (2017). Resistance Mechanisms to TP53-MDM2 Inhibition Identified by In Vivo piggyBac Transposon Mutagenesis Screen in an Arf−/−mouse Model. Proc. Natl. Acad. Sci. USA 114 (12), 3151–3156. doi:10.1073/pnas.1620262114
Chen, L., Willis, S. N., Wei, A., Smith, B. J., Fletcher, J. I., Hinds, M. G., et al. (2005). Differential Targeting of Prosurvival Bcl-2 Proteins by Their BH3-Only Ligands Allows Complementary Apoptotic Function. Mol. Cel 17 (3), 393–403. doi:10.1016/j.molcel.2004.12.030
Chipuk, J. E., Kuwana, T., Bouchier-Hayes, L., Droin, N. M., Newmeyer, D. D., Schuler, M., et al. (2004). Direct Activation of Bax by P53 Mediates Mitochondrial Membrane Permeabilization and Apoptosis. Science 303 (5660), 1010–1014. doi:10.1126/science.1092734
Daina, A., Michielin, O., and Zoete, V. (2017). SwissADME: a Free Web Tool to Evaluate Pharmacokinetics, Drug-Likeness and Medicinal Chemistry Friendliness of Small Molecules. Sci. Rep. 7, 42717. doi:10.1038/srep42717
Ding, K., Lu, Y., Nikolovska-Coleska, Z., Wang, G., Qiu, S., Shangary, S., et al. (2006). Structure-Based Design of Spiro-oxindoles as Potent, Specific Small-Molecule Inhibitors of the MDM2−p53 Interaction. J. Med. Chem. 49 (12), 3432–3435. doi:10.1021/jm051122a
Doi, K., Li, R., Sung, S.-S., Wu, H., Liu, Y., Manieri, W., et al. (2012). Discovery of Marinopyrrole A (Maritoclax) as a Selective Mcl-1 Antagonist that Overcomes ABT-737 Resistance by Binding to and Targeting Mcl-1 for Proteasomal Degradation. J. Biol. Chem. 287 (13), 10224–10235. doi:10.1074/jbc.m111.334532
Domingo, L. R. (2014). A New C-C Bond Formation Model Based on the Quantum Chemical Topology of Electron Density. RSC Adv. 4, 32415–32428. doi:10.1039/c4ra04280h
Domingo, L. R. (2016). Molecular Electron Density Theory: A Modern View of Reactivity in Organic Chemistry. Molecules 21, 1319. doi:10.3390/molecules21101319
Domingo, L. R., Chamorro, E., and Pérez, P. (2008a). Understanding the Reactivity of Captodative Ethylenes in Polar Cycloaddition Reactions. A Theoretical Study. J. Org. Chem. 73, 4615–4624. doi:10.1021/jo800572a
Domingo, L. R., Chamorro, E., and Pérez, P. (2010). Understanding the High Reactivity of the Azomethine Ylides in [3 + 2] Cycloaddition Reactions. Lett. Org. Chem. 7, 432–439. doi:10.2174/157017810791824900
Domingo, L., Ríos-Gutiérrez, M., and Pérez, P. (2016). Applications of the Conceptual Density Functional Theory Indices to Organic Chemistry Reactivity. Molecules 21, 748. doi:10.3390/molecules21060748
Domingo, L. R., Kula, K., and Ríos‐Gutiérrez, M. (2020a). Unveiling the Reactivity of Cyclic Azomethine Ylides in [3+2] Cycloaddition Reactions within the Molecular Electron Density Theory. Eur. J. Org. Chem., 5938–5948. doi:10.1002/ejoc.202000745
Domingo, L. R., Ríos-Gutiérrez, M., and Pérez, P. (2020b). A Molecular Electron Density Theory Study of the Participation of Tetrazines in Aza-Diels-Alder Reactions. RSC Adv. 10, 15394–15405. doi:10.1039/d0ra01548b
Domingo, L. R., Saéz, J. A., Zaragozá, R. J., and Arnó, M. (2008b). Understanding the Participation of Quadricyclane as Nucleophile in Polar [2σ + 2σ + 2π] Cycloadditions toward Electrophilic π Molecules. J. Org. Chem. 73, 8791–8799. doi:10.1021/jo801575g
Eymin, B., Gazzeri, S., Brambilla, C., and Brambilla, E. (2002). Mdm2 Overexpression and p14ARF Inactivation Are Two Mutually Exclusive Events in Primary Human Lung Tumors. Oncogene 21 (17), 2750–2761. doi:10.1038/sj.onc.1205359
Fridman, J. S., and Lowe, S. W. (2003). Control of Apoptosis by P53. Oncogene 22 (56), 9030–9040. doi:10.1038/sj.onc.1207116
Fukui, K. (1970). Formulation of the Reaction Coordinate. J. Phys. Chem. 74, 4161–4163. doi:10.1021/j100717a029
Ganguli, G., Abecassis, J., and Wasylyk, B. (2000). MDM2 Induces Hyperplasia and Premalignant Lesions when Expressed in the Basal Layer of the Epidermis. Embo J. 19 (19), 5135–5147. doi:10.1093/emboj/19.19.5135
Gollner, A., Rudolph, D., Arnhof, H., Bauer, M., Blake, S. M., Boehmelt, G., et al. (2016). Discovery of Novel Spiro[3H-indole-3,2′-pyrrolidin]-2(1H)-one Compounds as Chemically Stable and Orally Active Inhibitors of the MDM2-P53 Interaction. J. Med. Chem. 59 (22), 10147–10162. doi:10.1021/acs.jmedchem.6b00900
Haupt, Y., Maya, R., Kazaz, A., and Oren, M. (1997). Mdm2 Promotes the Rapid Degradation of P53. Nature 387 (6630), 296–299. doi:10.1038/387296a0
Irvine, J. D., Takahashi, L., Lockhart, K., Cheong, J., Tolan, J. W., Selick, H. E., et al. (1999). MDCK (Madin-Darby Canine Kidney) Cells: A Tool for Membrane Permeability Screening. J. Pharm. Sci. 88 (1), 28–33. doi:10.1021/js9803205
Islam, M. S., Al-Majid, A. M., El-Senduny, F. F., Badria, F. A., Rahman, A. F. M. M., Barakat, A., et al. (2020). Synthesis, Anticancer Activity, and Molecular Modeling of New Halogenated Spiro[pyrrolidine-thiazolo-oxindoles] Derivatives. Appl. Sci. 10 (6), 2170. doi:10.3390/app10062170
Islam, M. S., Ghawas, H. M., El-Senduny, F. F., Al-Majid, A. M., Elshaier, Y. A. M. M., Badria, F. A., et al. (2019). Synthesis of New Thiazolo-Pyrrolidine-(spirooxindole) Tethered to 3-acylindole as Anticancer Agents. Bioorg. Chem. 82, 423–430. doi:10.1016/j.bioorg.2018.10.036
Jerabek-Willemsen, M., Wienken, C. J., Braun, D., Baaske, P., and Duhr, S. (2011). Molecular Interaction Studies Using Microscale Thermophoresis. ASSAY Drug Develop. Tech. 9 (4), 342–353. doi:10.1089/adt.2011.0380
Khoo, K. H., Verma, C. S., and Lane, D. P. (2014). Drugging the P53 Pathway: Understanding the Route to Clinical Efficacy. Nat. Rev. Drug Discov. 13 (3), 217–236. doi:10.1038/nrd4236
Kojima, K., Konopleva, M., Samudio, I. J., Schober, W. D., Bornmann, W., and Andreeff, M. (2006). Concomitant Inhibition of MDM2 and Bcl-2 Protein Function Synergistically Induce Mitochondrial Apoptosis in AML. Cell cycle 5 (23), 2778–2786. doi:10.4161/cc.5.23.3520
Konopleva, M., Martinelli, G., Daver, N., Papayannidis, C., Wei, A., Higgins, B., et al. (2020). MDM2 Inhibition: an Important Step Forward in Cancer Therapy. Leukemia 34 (11), 2858–2874. doi:10.1038/s41375-020-0949-z
Kracikova, M., Akiri, G., George, A., Sachidanandam, R., and Aaronson, S. A. (2013). A Threshold Mechanism Mediates P53 Cell Fate Decision between Growth Arrest and Apoptosis. Cell Death Differ 20 (4), 576–588. doi:10.1038/cdd.2012.155
Li, J., Viallet, J., and Haura, E. B. (2008). A Small Molecule Pan-Bcl-2 Family Inhibitor, GX15-070, Induces Apoptosis and Enhances Cisplatin-Induced Apoptosis in Non-small Cell Lung Cancer Cells. Cancer Chemother. Pharmacol. 61 (3), 525–534. doi:10.1007/s00280-007-0499-3
Lipinski, C. A., Lombardo, F., Dominy, B. W., and Feeney, P. J. (2017). Experimental and Computational Approaches to Estimate Solubility and Permeability in Drug Discovery and Development Settings. Adv. Drug Deliv. Rev. 46 (1-3), 3–26. doi:10.1016/s0169-409x(00)00129-0
Long, J., Parkin, B., Ouillette, P., Bixby, D., Shedden, K., Erba, H., et al. (2010). Multiple Distinct Molecular Mechanisms Influence Sensitivity and Resistance to MDM2 Inhibitors in Adult Acute Myelogenous Leukemia. Blood Am. J. Hematol. 116 (1), 71–80. doi:10.1182/blood-2010-01-261628
Lujambio, A., Akkari, L., Simon, J., Grace, D., Tschaharganeh, D. F., Bolden, J. E., et al. (2013). Non-cell-autonomous Tumor Suppression by P53. Cell 153 (2), 449–460. doi:10.1016/j.cell.2013.03.020
Ma, X.-l., Chen, C., and Yang, J. (2005). Predictive Model of Blood-Brain Barrier Penetration of Organic Compounds1. Acta Pharmacologica Sinica 26 (4), 500–512. doi:10.1111/j.1745-7254.2005.00068.x
Mihara, M., Erster, S., Zaika, A., Petrenko, O., Chittenden, T., Pancoska, P., et al. (2003). p53 Has a Direct Apoptogenic Role at the Mitochondria. Mol. Cel 11 (3), 577–590. doi:10.1016/s1097-2765(03)00050-9
Mosmann, T. (1983). Use of MTT Colorimetric Assay to Measure Cell Activation. J. Immunol. Methods 65 (1), 55. doi:10.1016/0022-1759(83)90303-4
Muegge, I., Heald, S. L., and Brittelli, D. (2011). Simple Selection Criteria for Drug-like Chemical Matter. J. Med. Chem. 44 (12), 1841–1846. doi:10.1021/jm015507e
Nakamaru, K., Seki, T., Tazaki, K., and Tse, A. (2015). Abstract B5: Preclinical Characterization of a Novel Orally-Available MDM2 Inhibitor DS-3032b: Anti-tumor Profile and Predictive Biomarkers for Sensitivity. Mol. Cancer Ther. 2015, 14. doi:10.1158/1535-7163.targ-15-b5
Oliner, J. D., Kinzler, K. W., Meltzer, P. S., George, D. L., and Vogelstein, B. (1992). Amplification of a Gene Encoding a P53-Associated Protein in Human Sarcomas. Nature 358 (6381), 80–83. doi:10.1038/358080a0
Oliner, J. D., Pietenpol, J. A., Thiagalingam, S., Gyuris, J., Kinzler, K. W., and Vogelstein, B. (1993). Oncoprotein MDM2 Conceals the Activation Domain of Tumour Suppressor P53. Nature 362 (6423), 857–860. doi:10.1038/362857a0
Parr, R. G., Szentpaly, L. V., and Liu, S. (1999). Electrophilicity index. J. Am. Chem. Soc. 121, 1922–1924. doi:10.1021/ja983494x
Parr, R. G., and Yang, W. (1989). Density-Functional Theory of Atoms and Molecules. New York: Oxford University Press.
Pistritto, G., Trisciuoglio, D., Ceci, C., Garufi, A., and D'Orazi, G. (2016). Apoptosis as Anticancer Mechanism: Function and Dysfunction of its Modulators and Targeted Therapeutic Strategies. Aging 8 (4), 603–619. doi:10.18632/aging.100934
Popowicz, G. M., Czarna, A., Wolf, S., Wang, K., Wang, W., Dömling, A., et al. (2010). Structures of Low Molecular Weight Inhibitors Bound to MDMX and MDM2 Reveal New Approaches for P53-Mdmx/mdm2 Antagonist Drug Discovery. Cell cycle 9 (6), 1104–1111. doi:10.4161/cc.9.6.10956
Popowicz, G. M., Dömling, A., and Holak, T. A. (2011). The Structure‐Based Design of Mdm2/Mdmx-P53 Inhibitors Gets Serious. Angew. Chem. Int. Ed. 50 (12), 2680–2688. doi:10.1002/anie.201003863
PreADMET (2020). Available at: https://preadmet.bmdrc.kr/adme/(Accessed 8 14, 2020).
Reed, J. C. (1999). Dysregulation of Apoptosis in Cancer. Jco 17 (9), 2941. doi:10.1200/jco.1999.17.9.2941
Ríos-Gutiérrez, M., and Domingo, L. R. (2019). Unravelling the Mysteries of the [3+2] Cycloaddition Reactions. Eur. J. Org. Chem. 2019, 267–282. doi:10.1002/ejoc.201800916
Rizk, O. H., Teleb, M., Abu-Serie, M. M., and Shaaban, O. G. (2019). Dual VEGFR-2/PIM-1 Kinase Inhibition Towards Surmounting the Resistance to Antiangiogenic Agents via Hybrid Pyridine and Thienopyridine-Based Scaffolds: Design, Synthesis and Biological Evaluation. Bioorg. Chem. 92, 103189. doi:10.1016/j.bioorg.2019.103189
Schuler, M., Bossy-Wetzel, E., Goldstein, J. C., Fitzgerald, P., and Green, D. R. (2000). p53 Induces Apoptosis by Caspase Activation through Mitochondrial Cytochrome C Release. J. Biol. Chem. 275 (10), 7337–7342. doi:10.1074/jbc.275.10.7337
Seidel, S. A. I., Dijkman, P. M., Lea, W. A., van den Bogaart, G., Jerabek-Willemsen, M., Lazic, A., et al. (2013). Microscale Thermophoresis Quantifies Biomolecular Interactions under Previously Challenging Conditions. Methods 59 (3), 301–315. doi:10.1016/j.ymeth.2012.12.005
Tovar, C., Graves, B., Packman, K., Filipovic, Z., Xia, B. H. M., Tardell, C., et al. (2013). MDM2 Small-Molecule Antagonist RG7112 Activates P53 Signaling and Regresses Human Tumors in Preclinical Cancer Models. Cancer Res. 73 (8), 2587–2597. doi:10.1158/0008-5472.can-12-28072013
Vazquez, A., Bond, E. E., Levine, A. J., and Bond, G. L. (2008). The Genetics of the P53 Pathway, Apoptosis and Cancer Therapy. Nat. Rev. Drug Discov. 7 (12), 979–987. doi:10.1038/nrd2656
Veber, D. F., Johnson, S. R., Cheng, H.-Y., Smith, B. R., Ward, K. W., and Kopple, K. D. (2002). Molecular Properties that Influence the Oral Bioavailability of Drug Candidates. J. Med. Chem. 45 (12), 2615–2623. doi:10.1021/jm020017n
Villunger, A., Michalak, E. M., Coultas, L., Müllauer, F., Böck, G., Ausserlechner, M. J., et al. (2003). p53- and Drug-Induced Apoptotic Responses Mediated by BH3-Only Proteins Puma and Noxa. Science 302 (5647), 1036–1038. doi:10.1126/science.1090072
Vogelstein, B., Papadopoulos, N., Velculescu, V. E., Zhou, S., Diaz, L. A., and Kinzler, K. W. (2013). Cancer Genome Landscapes. Science 339 (6127), 1546–1558. doi:10.1126/science.1235122
Wan, Y., Dai, N., Tang, Z., and Fang, H. (2018). Small-molecule Mcl-1 Inhibitors: Emerging Anti-tumor Agents. Eur. J. Med. Chem. 146, 471–482. doi:10.1016/j.ejmech.2018.01.076
Wang, S., Sun, W., Zhao, Y., McEachern, D., Meaux, I., Barrière, C., et al. (2014). SAR405838: An Optimized Inhibitor of MDM2-P53 Interaction that Induces Complete and Durable Tumor Regression. Cancer Res. 74 (20), 5855–5865. doi:10.1158/0008-5472.can-14-0799
Wong, R. S. (2011). Apoptosis in Cancer: from Pathogenesis to Treatment. J. Exp. Clin. Cancer Res. 30 (1), 87. doi:10.1186/1756-9966-30-87
World Health Organization (2020). WHO Report on Cancer: Setting Priorities, Investing Wisely and Providing Care for All. Geneva, Switzerland: World Health Organization. License: CC BY-NC-SA 3.0 IGO. Available at: https://apps.who.int/iris/handle/10665/330745.
Yamashita, S., Furubayashi, T., Kataoka, M., Sakane, T., Sezaki, H., and Tokuda, H. (2000). Optimized Conditions for Prediction of Intestinal Drug Permeability Using Caco-2 Cells. Eur. J. Pharm. Sci. 10 (3), 195–204. doi:10.1016/s0928-0987(00)00076-2
Yazdanian, M., Glynn, S. L., Wright, J. L., and Hawi, A. (1998). Correlating Partitioning and Caco-2 Cell Permeability of Structurally Diverse Small Molecular Weight Compounds. Pharm. Res. 15 (9), 1490–1494. doi:10.1023/a:1011930411574
Yee, S. (2011). In Vitro permeability across Caco-2 Cells (Colonic) Can Predict In Vivo (Small Intestinal) Absorption in Man-Ffact or Myth. Pharm. Res. 14 (6), 763–766. doi:10.1023/a:1012102522787
Yenupuri, S., Hariharan, A. V. L. N. S., Bugata, B. K., and Nori, D. L. S. (2014). Microwave Assisted Synthesis and Biological Evaluation of a Series of 1,5-benzothiazepines as Potential Cytotoxic and Antimicrobial Agents. Eur. J. Chem. 5 (1), 138–143. doi:10.5155/eurjchem.5.1.138-143.924
Keywords: spirooxindole, protein–protein interaction (PPI), p53, human homolog of mouse double minute 2 (MDM2), Bcl 2
Citation: Aziz YMA, Lotfy G, Said MM, El Ashry ESH, El Tamany ESH, Soliman SM, Abu-Serie MM, Teleb M, Yousuf S, Dömling A, Domingo LR and Barakat A (2021) Design, Synthesis, Chemical and Biochemical Insights Into Novel Hybrid Spirooxindole-Based p53-MDM2 Inhibitors With Potential Bcl2 Signaling Attenuation. Front. Chem. 9:735236. doi: 10.3389/fchem.2021.735236
Received: 02 July 2021; Accepted: 07 October 2021;
Published: 14 December 2021.
Edited by:
Matthew A. Addicoat, Nottingham Trent University, United KingdomReviewed by:
Yuvaraj Arun, Hebrew University of Jerusalem, IsraelAmelia Pilar Rauter, University of Lisbon, Portugal
Copyright © 2021 Aziz, Lotfy, Said, El Ashry, El Tamany, Soliman, Abu-Serie, Teleb, Yousuf, Dömling, Domingo and Barakat. This is an open-access article distributed under the terms of the Creative Commons Attribution License (CC BY). The use, distribution or reproduction in other forums is permitted, provided the original author(s) and the copyright owner(s) are credited and that the original publication in this journal is cited, in accordance with accepted academic practice. No use, distribution or reproduction is permitted which does not comply with these terms.
*Correspondence: Assem Barakat, ambarakat@ksu.edu.sa
†Deceased