Molecular iodine-promoted oxidative cyclization for the synthesis of 1,3,4-thiadiazole-fused- [1,2,4]-thiadiazole incorporating 1,4-benzodioxine moiety as potent inhibitors of α-amylase and α-glucosidase: In vitro and in silico study
- 1Department of Chemistry, Hazara University, Mansehra, Khyber Pakhtunkhwa, Pakistan
- 2Department of Chemistry, Abbottabad University of Science and Technology (AUST), Abbottabad, Pakistan
- 3Department of Chemistry, School of Natural Sciences (SNS), National University of Science and Technology (NUST), Islamabad, Pakistan
- 4Department of Chemistry, College of Science, Princess Nourah bint Abdulrahman University, Riyadh, Saudi Arabia
- 5Department of Pharmaceutical Sciences, College of Pharmacy, AlMaarefa University, Riyadh, Saudi Arabia
- 6Department of Chemistry, Faculty of Applied Science, Umm Al-Qura University, Makkah, Saudi Arabia
- 7Department of Chemistry, College of Science, Taif University, Taif, Saudi Arabia
- 8Department of Biotechnology College of Science, Taif University, Taif, Saudi Arabia
Twenty-five analogs were synthesized based on 1,3,4-thiadiazole-fused-[1,2,4]-thiadiazole incorporating 1,4-benzodioxine moiety (1–25) and then tested for the antidiabetic profile. The entire afforded derivatives showed varied inhibition profiles ranging between 0.70 ± 0.01 and 30.80 ± 0.80 μM (against α-amylase) in comparison to standard acarbose (12.80 ± 0.10 μM). Similarly, synthetics analogs also displayed a varied range of α-glucosidase activity ranging from 0.80 ± 0.01 μM to IC50 = 29.70 ± 0.40 μM (against α-glucosidase) as compared to standard acarbose (IC50 = 12.90 ± 0.10 μM). Among synthesized analogs, compound 22 showed excellent potency due to the presence of di-hydroxy substitutions at the 2,3-position of the aryl ring. For all analogs, the structure–activity relationship was carried out based on the pattern of substitutions around the aryl ring, and further, the potent analogs were subjected to a molecular docking study to analyze how active residues of targeted enzymes interact with active parts of newly prepared analogs. The result obtained shows that these compounds furnish several key interactions with enzyme active sites and, hence, enhanced their enzymatic activities.
1 Introduction
A high concentration of glucose in plasma results in diabetes, which is a major metabolic problem (Mitrakou et al., 1992). Diabetes, which occurs in two types, namely, type-1 and type-2, is one of the primary problems. Inadequate insulin production is a defining feature of type-2 diabetes, commonly referred to as non-insulin-subordinate diabetes (Fall et al., 2006). Because type-2 diabetes is regarded as a preventable illness, it is more significant than type-1 diabetes. An imbalance between glucose consumption and insulin release is the cause of type-2 diabetes. The fundamental strategy for preventing type-2 diabetes is blood sugar control (Baron, 1998). Through advice and food management, it is reasonable to achieve this target with active insulin release (Goldberg, 1998; Kahn, 2001; Rabasa‐Lhoret and Chiasson, 2003). It was suggested by the United Kingdom Prospective Diabetes Study (UKPDS) that one of the major treatments for diabetes is diet control. Eating practice that depends on the blood sugar list of foodstuff is presently one of the largely suggested health remedies. It was concluded that nutritional treatment can be used at the same time as former clinical medicines to achieve a greater impact (Jenkins et al., 1981; Wolever et al., 1994; American College of Sports Medicine, 2000). In some cases, this is the inconvenience of restricting the types and quantity of food consumed. A further probable arrangement is to reduce the speed of glucose absorbance from the small digestive area by holding up the processing of nutritional starch, the important nutritional concern of glucose (Rabasa‐Lhoret and Chiasson, 2003). For practical considerations, accommodations, and avoidance of outcomes, this strategy is seen as being more effective than managing insulin discharge (Kahn, 2001). The disruption of enzymes that convert dietary starch into glucose, such as α-amylase and α-glucosidase, has received attention as a method to control blood sugar levels (Ahrén et al., 2004; Ali et al., 2006; Geng et al., 2008). α-Amylase catalyzes the hydrolysis of (1,4)-glycosidic bonds, releasing glucose and maltose from starch (Takkinen et al., 1991; Svensson and Søgaard, 1993), while α-glucosidase releases glucose from maltose or possibly sucrose (Anderson et al., 2003; Mohan et al., 2007). The two of them are discharged in the little digestive tract, while just α-amylase is found inside saliva. The retention of glucose in the circulatory system can be delayed to lower type-2 diabetes by reducing these two proteins (α-amylase and α-glucosidase). These efforts have been undertaken to differentiate between inhibitors of α-glucosidase and α-amylase that can be used as food or food additives. However, the evidence (Seo et al., 2005; Wakita et al., 2005) demonstrated that several sugar-like phenolic substances have the potential to block α-glucosidase. The majority of research on α-glucosidase and α-amylase inhibitors has been concentrated on phenolic substances (Hermeking, 2007; Bhandari et al., 2008).
Blood glucose levels over normal or hyperglycemia are indicated by impaired glucose tolerance (IGT) or impaired fasting blood glucose levels (IFG). In contrast to IFG, which occurs when blood glucose levels often exceed normal blood glucose concentrations above 7 mmol/L, IGT is characterized by blood glucose levels that are greater than 11 mmol/L 2 hours after a 75-g oral glucose load. In recent investigations of diabetes as a cause of male factor infertility, IGF and IGT were employed as diagnostic criteria. They are independent markers for diagnosis of diabetic mellitus (DM). These studies found that diabetes mellitus affects sperm motility, sperm counts, semen volume, and normal sperm morphology in addition to impairing erectile and ejaculatory function (Alves et al., 2013a; Alves et al., 2013b). There will be more men of reproductive age than ever before as the prevalence of diabetes is expected to reach 422 million people worldwide in 2014, and the number of children and adolescents with the condition is increasing (Wild et al., 2004; Agbaje et al., 2007). Furthermore, it is becoming more widely recognized that male infertility is a sign of declining male health (Eisenberg et al., 2015), especially when sexual dysfunction is prevalent (Lotti et al., 2016).
It was reported that numerous marketed drugs hold heteroarenes as the core center in their structure (Leeson and Springthorpe, 2007). Among heteroarene-bearing scaffolds, fused heteroarene is known as a prominent entity in numerous biologically active analogs for drug discovery and, hence, received much interest from medicinal chemists due to their presence in several biologically potent drugs. Furthermore, fused heterocyclic compounds displayed interesting biological activities such as benzothiazole fused with imidazole biologically reported as a potent antitumor agent for treatment of lung cancer in humans (Amino et al., 2006). Moreover, triazole fused with the benzoxazole moiety was reported to display an antibacterial profile (Shreedhara et al., 2017). Imidazole fused with benzo imidazole was reported to exhibit antianxiety activity for treatment of neuropsychiatric disorders (Han et al., 2005). Thiadiazole scaffolds as the core center of numerous sulfur-containing five-membered rings were found to have a broad spectrum of biological activities, such as antibacterial and neuroprotective profiles (Perlovich et al., 2012; Fang et al., 2017) (Figure 1).
One of the most difficult areas of drug development, particularly for oral-drug delivery systems, continues to be the enhancement of drug solubility and, consequently, its oral bioavailability. The solubility of poorly water-soluble medications can be improved using a variety of techniques that have been published in the literature. The methods are chosen based on a number of factors, including the qualities of the medicine under consideration, the kind of excipients to be chosen, and the kind of dosage form intended. Poor solubility and a sluggish rate of drug dissolution in aqueous gastrointestinal fluids are common causes of insufficient bioavailability for poorly water-soluble drugs. Especially for class II (low solubility and high permeability) drugs, increase in the drug’s solubility and rate of dissolution in gastrointestinal fluids may enhance bioavailability. Increasing solubility also enhances the bioavailability of Biopharmaceutical Classification System (BCS) class II medicines because, rather than absorption, the rate-limiting processes for BCS class II drugs are solubility in the stomach fluid and drug release from the dose form (Savjani et al., 2012).
Consequently, the broad range of biological activities possessed by these fused heterocyclic compounds, especially benzimidazole/benzoxazole/benzothiazole fused with thiadiazole/selenadiazole (Chen et al., 2020) (Figure 2) and further α-glucosidase and α-amylase inhibition properties possessed by thiadiazole analogs (Taha et al., 2017; Khan et al., 2022a), motivated us to design and synthesize a new class of heterocyclic compounds based on fused heteroarene such as 1,3,4-thiadiazole fused with 1,2,4-thiadiazole incorporating the benzodioxine moiety as an antidiabetic agent for treatment of the diabetic patient. Keeping in mind the biological importance of fused heterocyclic compounds, we deliberately carried out the synthesis of 1,4-benzodioxin-based 1,3,4-thiadiazole-fused-[1,2,4]-thiadiazole analogs and studied their α-amylase and α-glucosidase activities. Studies on the structure–activity connection and molecular docking were excluded to dilute the findings.
2 Results and discussion
2.1 Chemistry
Twenty-five analogs of 1,4-benzodioxin-based 1,3,4-thiadiazole-fused-[1,2,4]-thiadiazole were synthesized (Table 1). First of all, 2-formyl 1,4-benzodioxine (I, 1 equivalent) was reacted with thiosemicarbazide (1 equivalent) in methanol (10 ml) under the catalytic action of sodium acetate (0.8 mmol) to afford 1,4-benzodioxine-based thiosemicarbazone as the substrate (II). This intermediate was cleaned with hexane and deionized water to eliminate all impurities. Substrate (II, 1 equivalent) was subjected to an iodine-mediated (0.6 mmol) cyclization in 1,4-dioxane (10 ml) in the presence of potassium carbonate (0.8 mmol), and the resulting mixture was stirred for 12–14 h under reflux to obtain 1,4-benzodioxine-based 1,3,4-thiadiazole-2-amine as an intermediate (III). In the last step, substrate (III, 1 equivalent) further undergoes [3+2] iodine-mediated (0.6 mmol) oxidative cyclization with different phenylisothiocyanates (1 equivalent) in chloroform (10 ml) and potassium carbonate (0.8 mmol), and the resulting mixture was put on reflux for 16 h. It was reacted with a 5% sodium thiosulfate solution after being cooled to 25°C, followed by extraction with DCM (10 ml) to obtain targeted 1,4-benzodioxin-based 1,3,4-thiadiazole-fused-[1,2,4]-thiadiazole analogs (1–25) in an appropriate yield. The reaction progress was checked by employing a TLC plate. Different spectroscopic tools, including HREIMS and NMR, were employed to find the exact structure of the afforded analogs (Scheme 1).
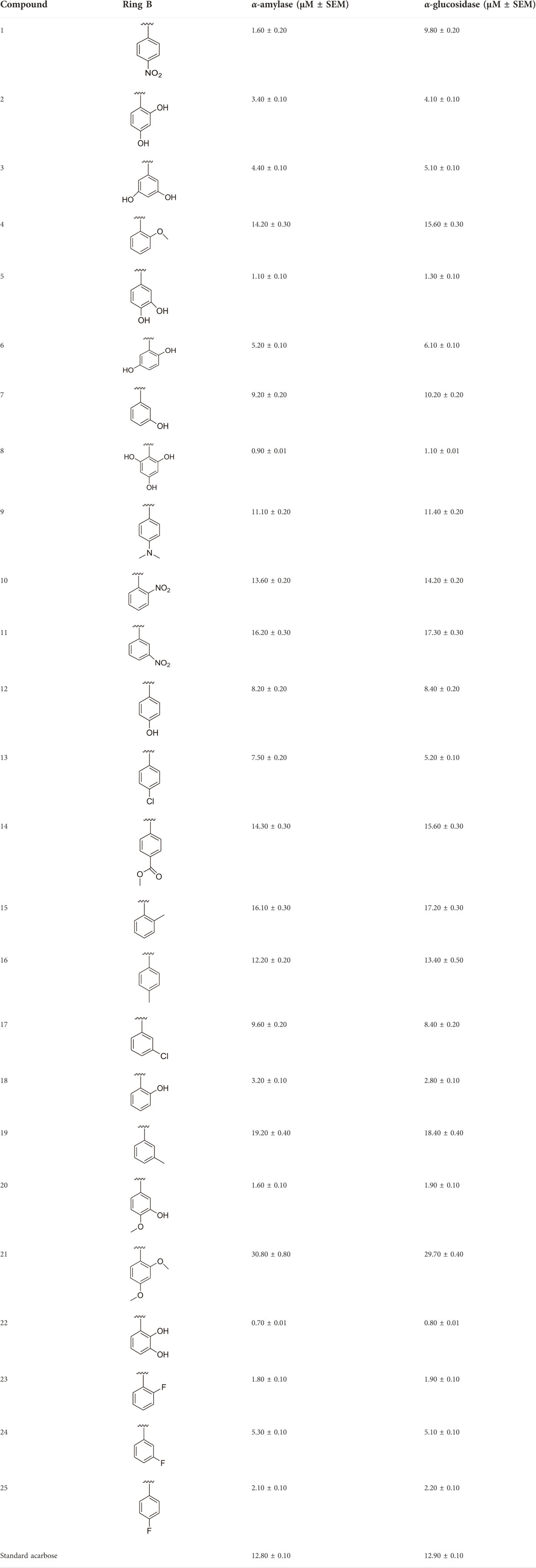
TABLE 1. Different substituents and inhibitory potentials (in vitro) of newly prepared analogs of 1,3,4-thiadiazole-fused-[1,2,4]-thiadiazole incorporating 1,4-benzodioxine skeleton against α-amylase and α-glucosidase enzymes.
2.2 In vitro α-amylase and α-glucosidase inhibitory activities (1–25)
All of the synthetically created hybrid analogs based on 1,3,4-thiadiazole-fused-1[1,2,4]-thiadiazole containing the 1,4-benzodioxine ring were generated and then evaluated for their (in vitro) inhibitory profiles for α-amylase and α-glucosidase. The entire analogs illustrated remarkable potency against α-amylase and α-glucosidase having IC50 values ranging from 0.70 ± 0.01 to 30.80 ± 0.80 μM (α-amylase) and 0.80 ± 0.01 to 29.70 ± 0.40 μM (α-glucosidase) when compared to standard acarbose drugs. It was suggested by SAR studies that each part of the synthesized analogs such as benzodioxine ring A, 1,3,4-thiadiazole-fused-[1,2,4]-thiadiazole and aryl ring B actively participated in the activity, and any variation around ring B resulted to different activity (Figure 3).
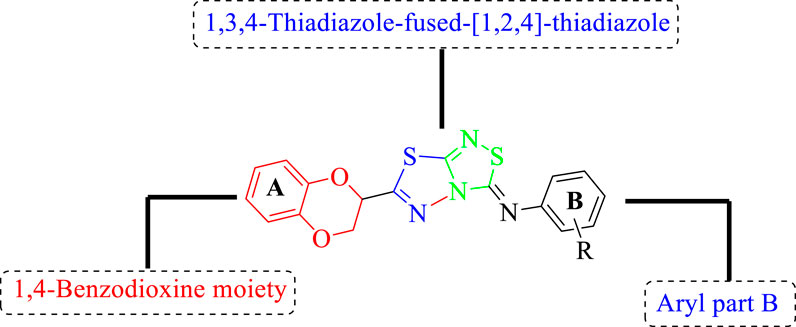
FIGURE 3. General structure of 1,4-benzodioxin-based 1,3,4-thiadiazole-fused-[1,2,4]-thiadiazole analogs (1–25).
2.2.1 Structure–activity relationship for α-amylase activity (1–25)
Among the synthesized analogs, the analogs bearing tri-hydroxy and di-hydroxy substitutions around ring B have emerged as potent inhibitors of α-amylase among the series. By comparing analog 22 (IC50 = 0.70 ± 0.01 μM) bearing di-hydroxy substitutions at the 2,3-position of ring B showed excellent α-amylase activity when compared to other analogs 2 (IC50 = 3.40 ± 0.10 μM), 3 (IC50 = 4.40 ± 0.10 μM), 5 (IC50 = 1.10 ± 0.10 μM), 6 (IC50 = 5.20 ± 0.10 μM),7 (IC50 = 9.20 ± 0.20 μM), 12 (IC50 = 8.20 ± 0.20 μM), 18 (IC50 = 3.20 ± 0.10 μM), and analog 8 (IC50 = 0.90 ± 0.01 μM bearing tri-hydroxy substitutions at 2,4,6-position of phenyl ring) under the positive control of standard acarbose (IC50 = 12.80 ± 0.10 μM). This enhanced activity of analog 22 showed the presence of attached di-hydroxy groups at the 3,4-position, which could enhance the inhibitory potentials through active participation in conventional hydrogen bonding with enzyme active residues. Moreover, analog 8 bearing tri-hydroxy substitutions at the 2,4,6-position of ring B was found to be second most potent and displayed enhanced activity than di-hydroxy substitutions bearing analogs 2, 3, 5, and 6, indicating that any variation in the position of substitution around ring B may result to a different activity. In addition, analogs 2, 3, and 5 bearing di-hydroxy substitution at phenyl ring B displayed superior activity than analogs 7 and 12 bearing only mono-hydroxy substitution. This enhanced activity of analogs 2, 3, and 5 was due to greater numbers of the attached hydroxy groups that might have more tendencies to interact with the active site of the enzyme. However, by comparing structurally similar analogs 7, 18, and 12 having a mono-hydroxy substitution at meta-, ortho-, and para-position of the phenyl ring, among these analogs, 18 was more potent than its counterparts 7 and 12. This difference in activity shows that alteration in the position of substituted around phenyl ring B greatly affects the activity (Figure 4).
In the same way, by comparing para-nitro moiety-bearing analog 1 with analogs 10 and 11 that hold the nitro moiety at ortho- and meta-position of the phenyl ring, compound 1 displayed better activity than its structurally similar compounds 10 and 11. This indicates that inhibitory potentials were greatly affected by altering the position of the electron-withdrawing nitro group around the phenyl ring attached to the thiadiazole-fused-thiadiazole skeleton (Figure 5).
The fluoro-substituted analogs show remarkable potency when compared to the –Cl moiety-bearing analogs. This was due to the strong EW nature of the –F moiety than the –Cl group which has a lower tendency of electron-withdrawing effect. Among fluoro-substituted analogs such as 23 (IC50 = 1.80 ± 0.10 μM), 24 (IC50 = 5.30 ± 0.10 μM), and 25 (IC50 = 2.10 ± 0.10 μM), the analogs 23 and 25 bearing the –F moiety at the ortho- and para-position of the phenyl ring showed superior potency when compared to its counterpart 24 that bears the –F moiety at meta-position. The diverse potency of these analogs was due to different attachment of the –F moiety around aryl ring B (Figure 6).
It was shown from SAR studies that attachment of the substituent of either weak electron-withdrawing or weak electron-donating nature such as –OCH3 and –CH3 groups resulted in decreased inhibitory potentials. Moreover, it was also noted that analogs bearing either substituent of the strong electron-withdrawing nature such as –NO2 and –F or substituents capable of forming hydrogen bonding such as –OH groups enhanced the inhibitory potentials. In addition, alteration in position, number/s, and nature of the substituent may result in diverse inhibitory potentials.
2.2.2 Structure–activity relationship for α-glucosidase activity (1–25)
It was noteworthy that the attachment of substituents that are capable of interactions in a better way with the active site of α-glucosidase such as –OH groups at appropriate positions enhanced the inhibitory potentials against α-glucosidase. Therefore, analog 22 bearing di-hydroxy substitutions at the 2, 3-position of the phenyl ring emerged as most active against α-glucosidase and showed ten-fold more potency than standard acarbose drug. This analog 22 having di-hydroxy substitutions shows better activity than other analogs 2, 3, and 5 bearing di-hydroxy substitutions at various positions of ring B, indicating that the hydroxyl group in greater numbers enhanced the inhibition profile. Compound 8 having tri-hydroxy moieties at 2,4,6-positions of the phenyl ring shows superior inhibitory activity when compared to other di-hydroxy group-bearing analogs such as 2 (bearing di-hydroxy at 2,4-position), 3 (bearing di-hydroxy at 3,5-position), and 5 (bearing di-hydroxy at 3,4-position). This superior activity of analog 8 was due to the greater number/s of attached hydroxyl moieties at the 2,4,6-position of phenyl ring B (Figure 7).
Among halogen-substituted analogs, the compounds bearing the –F moiety such as 23, 24, and 25 at ortho-, meta-, and para-position of the phenyl ring showed better activity than analogs such as 13 and 17 bearing the –Cl moiety at para- and meta-position of ring B, indicating that stronger electron-withdrawing effect shows that the moiety has more tendency of interactions with the active site of the enzyme and, therefore, enhanced the activity. Furthermore, comparing compound 23 bearing the –F moiety at the ortho-position of ring B showed superior potency in comparison to analogs 24 and 25 which also hold similar substituent like the –F group at the meta- and para-position of phenyl ring B. This difference in potency of these analogs was due to the different positions of the attached electron withdrawing –F group around ring B (Figure 8).
Analog 16 (IC50 = 13.40 ± 0.50 μM) holding methyl substitution at the para-position of ring B displayed superior potency in comparison to analogs 15 (IC50 = 17.20 ± 0.30 μM) and 19 (IC50 = 18.40 ± 0.40 μM) that also hold methyl groups at the ortho- and meta-position of phenyl ring B. This difference in potency of these analogs was due to the different positions of the attached electron donating –CH3 groups around ring B (Figure 9).
Overall, it was concluded that shifting the positions of substituents around aryl ring B had a significant impact on the inhibitory potentials against the α-glucosidase enzyme. It was also noted that alteration in inhibitory potentials was observed by attachment of substituents of different nature in different number/s around the phenyl ring linked to 1,3,4-thiadiazole-fused-[1,2,4]-thiadiazole incorporating the 1,4-benzodioxine skeleton.
2.3 Molecular docking study
The molecular docking had been conducted on α-amylase and α-glucosidase enzymes for most active compounds 22, 8, and 5 to explore the binding sites of newly prepared analogs and how they interact with the active site of both these targeted enzymes. From the in vitro study, it was concluded that compound 22 was found as the most potent inhibitor of both these targeted enzymes due to active participation of both attached hydroxyl groups in strong interactions such as hydrogen bonding. Therefore, keeping in mind the best inhibitory potentials of active analog 22, it is further subjected to a molecular docking study to explore its binding site, and the result obtained shows that compound 22 established several key interactions, such as Glu233 (H-bonding & pi–anion), Ile235 (pi–sigma and H-bonding), Leu162 and Ala198 (pi–pi T-shaped), His201 and Trp59 (pi–pi stacking), and numerous van der Waals interactions, when evaluated against α-amylase as shown in Figure 10A. While against α-glucosidase, it also furnished numerous key interactions, including Asp440 (CHB), Asp443 (CHB), His514 (amide–pi stacking), Lys421 (pi–alkyl), Met513 (pi–alkyl), and Asp423 (pi–anion), and several other residues interact through van der Waals interactions as shown in Figure 10B.
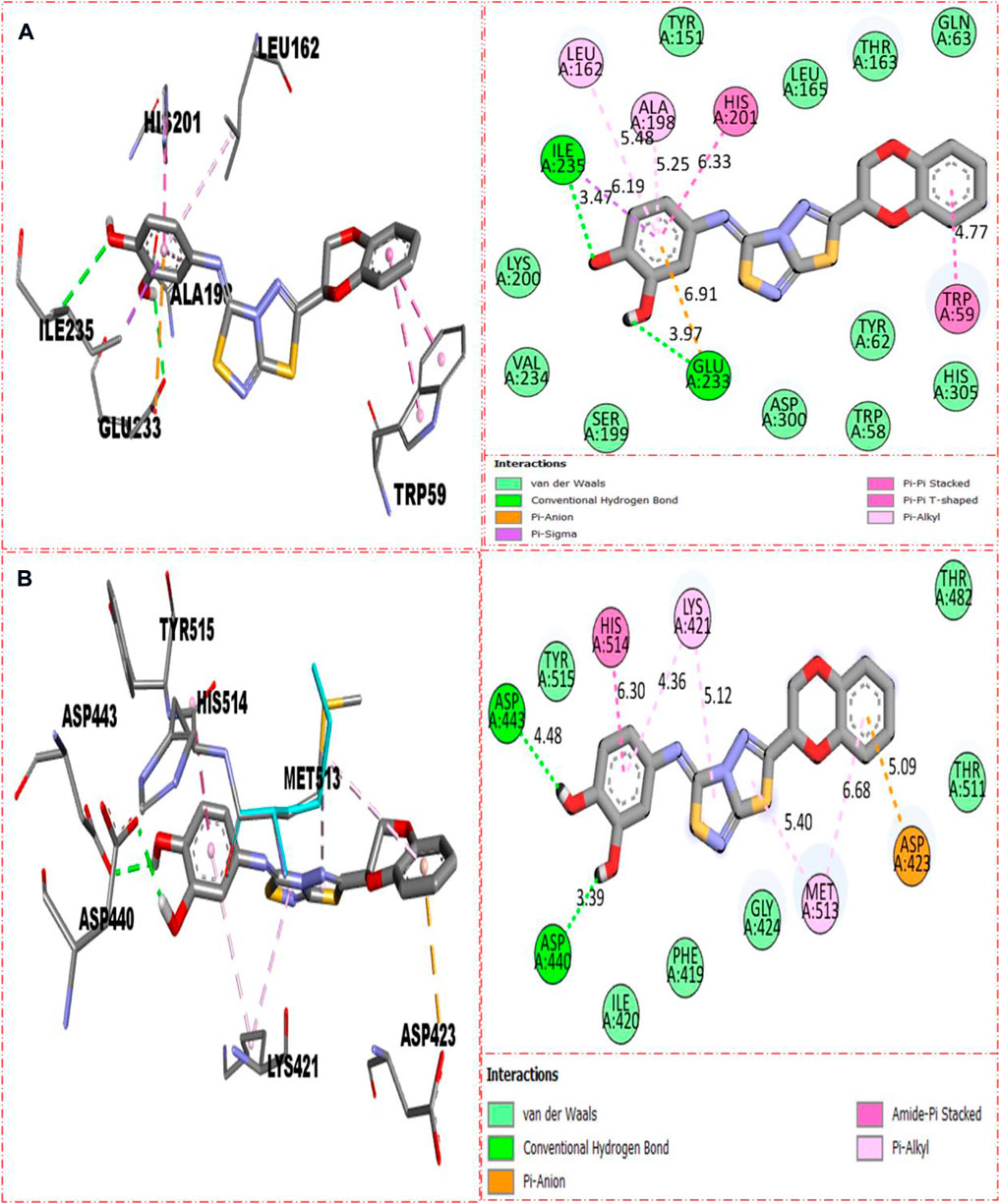
FIGURE 10. Represent the binding interactions of the most active compound 22. (A) represents compound 22 against α-amylase, while (B) denotes the same compound 22 against α-glucosidase enzyme and its 3D and 2D diagram.
For second-most active compound 8 bearing tri-hydroxyl groups at the 2,4,6-position of the aryl ring which can enhance its inhibitory potentials, this compound 8 when evaluated against α-amylase enzyme showed somewhat fewer interactions than compound 22, and, hence, its inhibitory potentials were found less than those of compound 22, which showed better binding interactions. Against α-amylase, this compound 8 revealed several interactions such as Lys200 (CHB), Tyr151 (pi–pi stacking), His201 (pi–sulfur & pi–cation), Ile235 (pi–alkyl), Ala198 (pi–pi T-shaped), Leu162 (pi–sigma & pi–alkyl), and van der Waals interactions (Figure 11A), while against α-glucosidase, it furnished numerous key interactions involving Asp423 (pi–anion), Met513 (pi–sulfur), Asp440 (CHB), His514 (amide–pi stacking), Lys421 (pi–alkyl), and numerous van der Waals interactions as shown in Figure 11B.
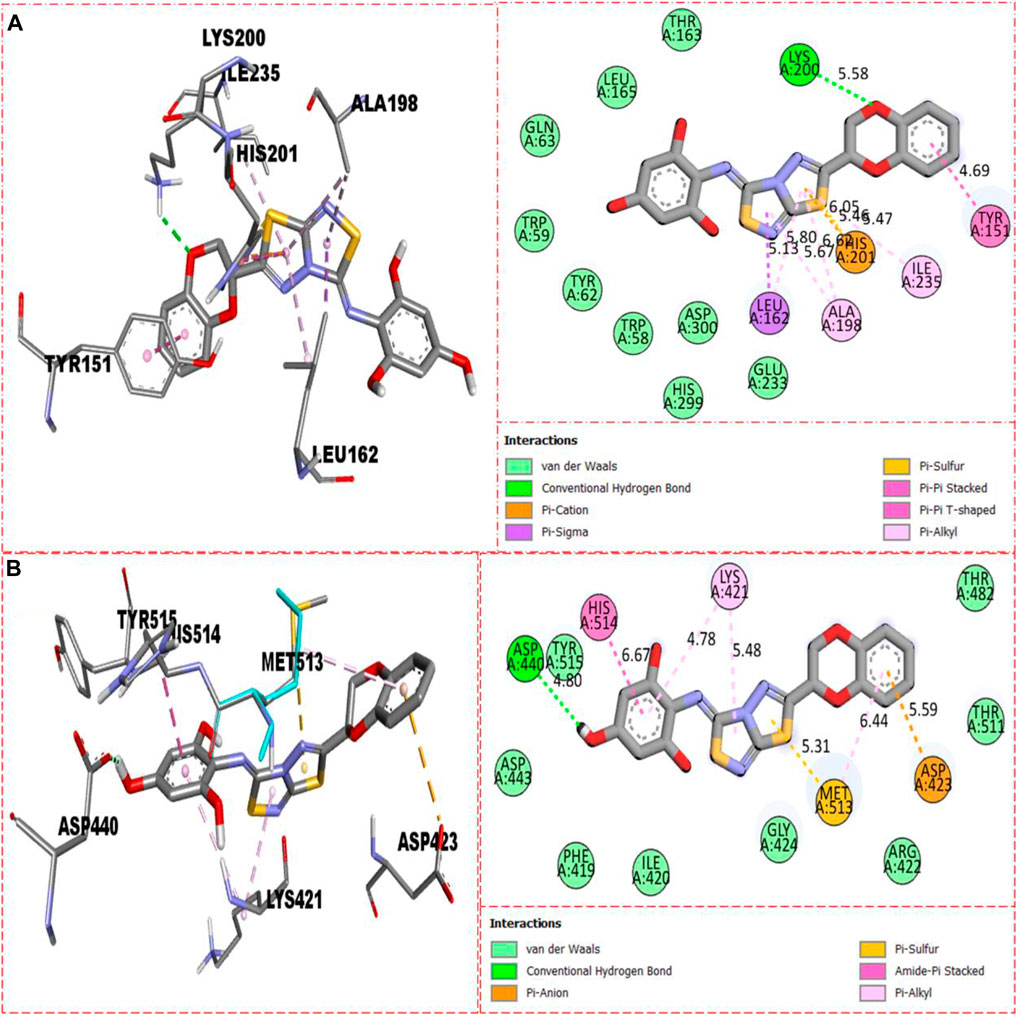
FIGURE 11. Represents the binding interactions of the second most active compound 8. (A) for compound 8 against α-amylase, while (B) for the same compound 5 against α-glucosidase enzyme and its 3D and 2D diagram.
Compound 5 was recognized as the third most active compound bearing di-hydroxy moieties at the 3,4-position of the aryl ring attached to fused thiadiazole rings. However, this compound 5 was found less potent than analog 22, which is structurally equivalent to it. It might be owing to the different positions of attached di-hydroxy groups around the aryl ring. The result obtained from the docking study shows that compound 5 exhibits various key interactions with the active residue of α-amylase, including Glu233 (CHB), Asp300 and Asp197 (pi–anion), Gly104 (carbon–hydrogen bond), Trp59 (pi–sulfur & pi–pi stacking), and van der Waals interactions (Figure 12A), while against α-glucosidase, it also displayed numerous interactions such as Asp440 (CHB), Asp423 (pi–anion), Met513 and Lys421 (pi–alkyl), and van der Waals interactions (Figure 12B).
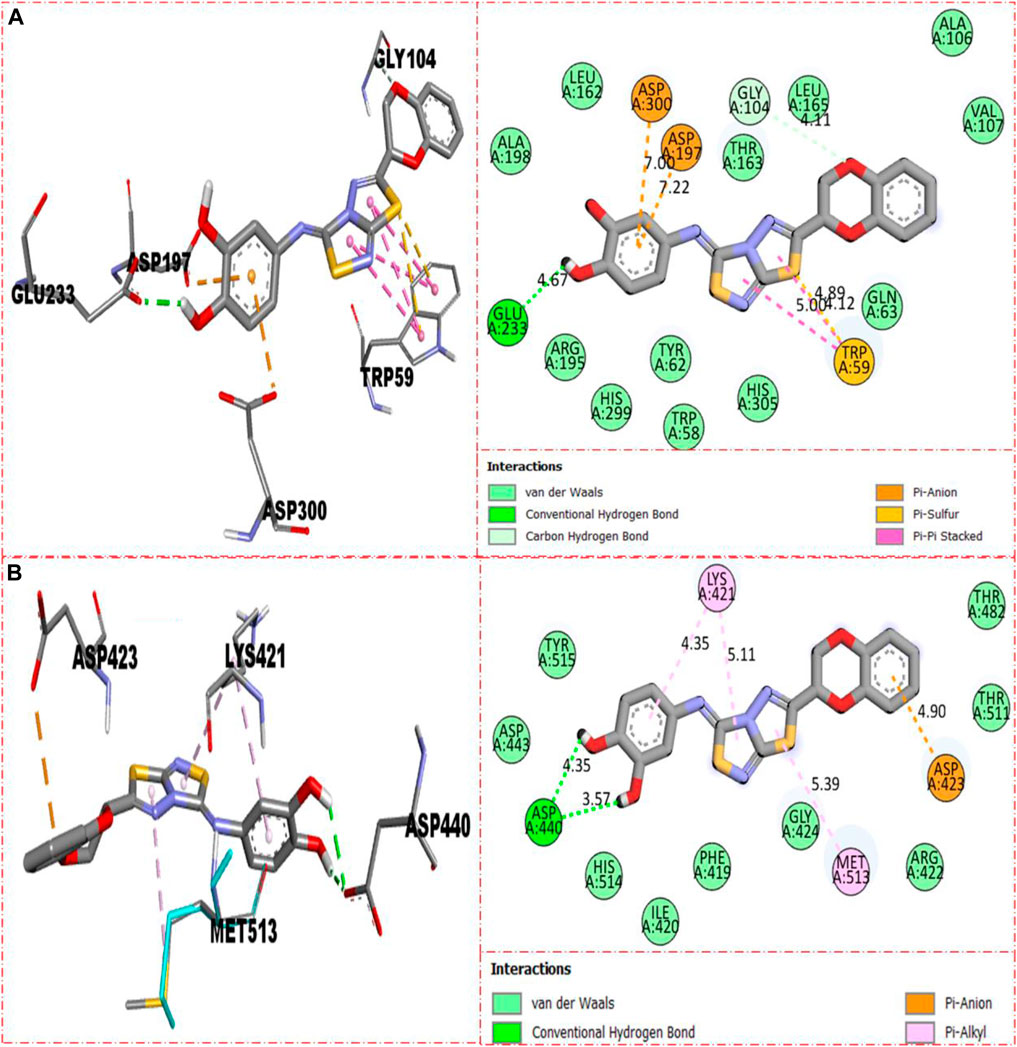
FIGURE 12. Represent the binding interactions of the third most active compound 5. (A) for compound 5 against α-amylase, while (B) for the same compound 5 against α-glucosidase enzyme and its 3D and 2D diagram.
3 Materials and methods
3.1 General information
All biological and chemical components were bought from Merck KGaA and Sigma-Aldrich Chemical Corp., both of St. Louis, Missouri, United States (Merck, Darmstadt, Germany). Using a Silica Gel 60 F254 TLC plate, thin-layer chromatography (TLC) was used to assess the purity of all intermediate and necessary chemicals (Merck KGaA, Darmstadt, Germany). A Stuart Melting Point Apparatus SMP30 was used to measure melting points (Staffordshire, United Kingdom). The 1H-NMR and 13C-NMR spectra were captured using a 500-MHz NMR instrument in units relative to a deuterated solvent serving as an internal reference. The electron impact high-resolution mass spectra (60 eV) were conducted on a Finnigan MAT-311A instrument (Germany). The chromatogram was shown using UV light from Schimazdu, Germany, with a wavelength of 254/365.
3.2 General procedure for the synthesis of 1,4-benzodioxine-based thiadiazole-fused-thiadiazole derivatives (1–25)
3.2.1 Synthesis of (E)-2-((2,3-dihydrobenzo[b][1,4]dioxin-2-yl)methylene)hydrazine-1-carbothioamide (II)
Thiosemicarbazide (1 equivalent), sodium acetate (0.8 mmol), and benzodioxine I (1 equivalent) were stirred together in 10 ml of methanol followed by addition of a formyl group containing benzodioxine I to it. A rotary evaporator was used to evaporate the solvent after the reaction mixture was agitated at 25°C for 3 h. The desired benzodioxine-based thiosemicarbazone II was produced in excellent yield after the residue was triturated with ethyl acetate/diethyl ether (5:95).
3.2.2 Synthesis of 5-(2,3-dihydrobenzo[b][1,4]dioxin-2-yl)-1,3,4-thiadiazol-2-amine (III)
Further dissolving the intermediate II (1 equivalent) in 1,4-dioxane (10 ml), potassium carbonate (0.8 mmol) and iodine (0.6 mmol) were successively added. In a nitrogen environment, the reaction mixture was heated to a reflux temperature until the formation of a new entity was complete (TLC was used to monitor the progress, 12–14 h). It was then brought to room temperature, treated with 20 ml of 5% Na2S2O3, and extracted using EtOAc (3 × 15 ml). Over anhydrous sodium sulfate, the mixed organic layer was dried and concentrated. In order to produce 2-amino-1,3,4-thiadiazole substrate III in a high yield, the supplied residue was purified using silica gel column chromatography with petroleum ether and EtOAc combination as the eluent.
3.2.3 Synthesis of targeted benzodioxine-based thiadiazole-fused-thiadiazole analogs (1–25)
Finally, 2-amino-1,3,4-thiadiazole-based substrate (III, 1 equivalent) further undergoes [3+2] oxidative cyclization on stirring overnight with different substituted phenyl isothiocyanates (1 equivalent) in the presence of molecular iodine (0.6 mmol) and potassium carbonates (0.8 mmol) in chloroform (10 ml) as a solvent to access the targeted 1,3,4-thiadiazole-fused-[1,2,4]-thiadiazole-based benzodioxine analogs (1–25) in an appropriate yield. The structures of all the newly discovered analogs were confirmed using spectroscopic techniques, including NMR and HREI-MS.
3.3 Assay protocol for the docking study
Utilizing Discovery Studio Visualizer’s (DSV) MGL tool 1.5.7 and AutoDock Vina, a molecular docking study was carried out (Li et al., 2015; Mrabti, 2018; Rao et al., 2021). In this study, the synthetic chemicals were tested against the enzymes glucosidase and amylase. These enzymes’ structures were found in the Protein Data Bank (PDB) by using the search criteria 1b2y & 3w37. The target protein and the prepared ligand were both saved in PDB format after the first step of protein preparation, which involved utilizing DSV to remove water molecules and existing ligands. The process was then continued in AutoDock, where the protein was charged with polar hydrogen, Kollman, and Gasteiger charges. Additionally, the chosen ligand was constructed using a torsion tree to find the root. Also, a configuration file was created and saved in the same docking folder along with the X, Y, and Z axes for both the ligand and protein in PDBQT format. The ligand was then generated in various postures using a command line, yielding a total of nine distinct poses in the PDBQT format. The dock protein and ligand were then opened in DSV to determine the ligand–enzyme active site interaction. The given study summarizes the additional information (Khan et al., 2022b).
4 Conclusion
In conclusion, we have designed and then synthesized twenty-five analogs (1–25) of thiadiazole-fused-[1,2,4]-thiadiazole-incorporating benzodioxine moiety. The literature-recognized procedure was used to evaluate the synthesized analogs’ ability to inhibit α-amylase and α-glucosidase. When compared to standard acarbose (IC50 = 12.80 ± 0.10 μM), (IC50 = 12.90 ± 0.10 μM), all analogs demonstrated a diverse range of efficacy with IC50 values ranging from 0.70 ± 0.01 to 30.80 ± 0.80 μM (against α-amylase) and 0.80 ± 0.01 to 29.70 ± 0.40 μM (α-glucosidase). The structure–activity relationship was carried out for all analogs based on substitution pattern around the aryl ring, and it was concluded from SAR studies that analogs holding hydroxyl moieties around the aryl ring enhanced the enzymatic activities against both these targeted enzymes via the formation of a strong conventional hydrogen bond with enzyme active sites. Additionally, a molecular docking study was performed on the potent analogs to determine the best way for them to interact with the active residues of the intended enzymes. The results revealed that these compounds provide a number of important interactions with the enzyme active site, increasing the enzymes’ enzymatic activities. Additionally, a number of spectroscopic techniques, including HREI-MS, 13C-NMR, and 1H-NMR, were employed to investigate the precise structure of the synthesized analogs.
Data availability statement
The original contributions presented in the study are included in the article/Supplementary Material; further inquiries can be directed to the corresponding authors.
Author contributions
All authors listed have made a substantial, direct, and intellectual contribution to the work and approved it for publication.
Funding
The authors would like to thank the Deanship of Scientific Research at Umm Al-Qura University for supporting this work by Grant Code: (22UQU4320141DSR45). Email: rapasha@uqu.edu.sa. This research was funded by Princess Nourah bint Abdulrahman University Researchers Supporting Project number (PNURSP2022R95), Princess Nourah bint Abdulrahman University, Riyadh, Saudi Arabia.
Acknowledgments
The authors would like to thank the Deanship of Scientific Research at Umm Al-Qura University for supporting this work by Grant Code: (22UQU4320141DSR45). Email: rapasha@uqu.edu.sa. This research was funded by Princess Nourah bint Abdulrahman University Researchers Supporting Project number (PNURSP2022R95), Princess Nourah bint Abdulrahman University, Riyadh, Saudi Arabia.
Conflict of interest
The authors declare that the research was conducted in the absence of any commercial or financial relationships that could be construed as a potential conflict of interest.
Publisher’s note
All claims expressed in this article are solely those of the authors and do not necessarily represent those of their affiliated organizations, or those of the publisher, the editors, and the reviewers. Any product that may be evaluated in this article, or claim that may be made by its manufacturer, is not guaranteed or endorsed by the publisher.
Supplementary material
The Supplementary Material for this article can be found online at: https://www.frontiersin.org/articles/10.3389/fchem.2022.1023316/full#supplementary-material
References
Mitrakou, A., Kelley, D., Mokan, M., Veneman, T., Pangburn, T., Reilly, J., et al. (1992). Role of reduced suppression of glucose production and diminished early insulin release in impaired glucose tolerance. N. Engl. J. Med. 326 (1), 22–29. doi:10.1056/nejm199201023260104
Fall, J. A., Holen, D. L., Davis, B., Krieg, T., and Koster, D. (2006). Subsistence harvests and uses of wild resources in iliamna, newhalen, nondalton, pedro bay, and port alsworth, Alaska: Alaska Department of Fish and Game Division of Subsistence.
Baron, A. D. (1998). Postprandial hyperglycaemia and α-glucosidase inhibitors. Diabetes Res. Clin. Pract. 40, S51–S55. doi:10.1016/s0168-8227(98)00043-6
Goldberg, A. L. (1998). Proteasome inhibitors: Valuable new tools for cell biologists. Trends Cell Biol. 8 (10), 397–403.
Kahn, S. E. (2001). [Beta]-Cell dysfunction and failure in type 2 diabetes. Diabetes 50 (2), S160. doi:10.2337/diabetes.50.2007.s160
Rabasa‐Lhoret, R., and Chiasson, J. L. (2003). α‐Glucosidase inhibitors. Int. Textb. diabetes mellitus. doi:10.1002/0470862092.d0612
American College of Sports Medicine, (2000). Position stand on exercise and type 2 diabetes. Med. Sci. Sports Exerc. 32, 1345–1360.
Jenkins, D. J., Wolever, T. M., Taylor, R. H., Barker, H., Fielden, H., Baldwin, J. M., et al. (1981). Glycemic index of foods: A physiological basis for carbohydrate exchange. Am. J. Clin. Nutr. 34 (3), 362–366. doi:10.1093/ajcn/34.3.362
Wolever, T. M., Katzman-Relle, L., Jenkins, A. L., Vuksan, V., Josse, R. G., and Jenkins, D. J. (1994). Glycaemic index of 102 complex carbohydrate foods in patients with diabetes. Nutr. Res. 14 (5), 651–669. doi:10.1016/s0271-5317(05)80201-5
Ali, S., Stone, M. A., Peters, J. L., Davies, M. J., and Khunti, K. (2006). The prevalence of co-morbid depression in adults with type 2 diabetes: A systematic review and meta-analysis. Diabet. Med. 23 (11), 1165–1173. doi:10.1111/j.1464-5491.2006.01943.x
Geng, P., Qiu, F., Zhu, Y., and Bai, G. (2008). Four acarviosin-containing oligosaccharides identified from Streptomyces coelicoflavus ZG0656 are potent inhibitors of α-amylase. Carbohydr. Res. 343 (5), 882–892. doi:10.1016/j.carres.2008.01.020
Ahrén, B. O., Landin-Olsson, M., Jansson, P. A., Svensson, M., Holmes, D., and Schweizer, A. (2004). Inhibition of dipeptidyl peptidase-4 reduces glycemia, sustains insulin levels, and reduces glucagon levels in type 2 diabetes. J. Clin. Endocrinol. Metab. 89 (5), 2078–2084. doi:10.1210/jc.2003-031907
Svensson, B., and Søgaard, M. (1993). Mutational analysis of glycosylase function. J. Biotechnol. 29 (1-2), 1–37. doi:10.1016/0168-1656(93)90038-o
Takkinen, K., Laukkanen, M. L., Sizmann, D., Alfthan, K., Immonen, T., Vanne, L., et al. (1991). An active single-chain antibody containing a cellulase linker domain is secreted by Escherichia coli. Protein Eng. Des. Sel. 4 (7), 837–841. doi:10.1093/protein/4.7.837
Mohan, S., Sim, L., Rose, D. R., and Pinto, B. M. (2007). Synthesis of S-alkylated sulfonium-ions and their glucosidase inhibitory activities against recombinant human maltase glucoamylase. Carbohydr. Res. 342 (7), 901–912. doi:10.1016/j.carres.2007.01.018
Anderson, G. G., Palermo, J. J., Schilling, J. D., Roth, R., Heuser, J., and Hultgren, S. J. (2003). Intracellular bacterial biofilm-like pods in urinary tract infections. Science 301 (5629), 105–107. doi:10.1126/science.1084550
Seo, M. J., Suh, S. Y., Bae, Y. C., and Jung, J. S. (2005). Differentiation of human adipose stromal cells into hepatic lineage in vitro and in vivo. Biochem. biophysical Res. Commun. 328 (1), 258–264. doi:10.1016/j.bbrc.2004.12.158
Wakita, T., Pietschmann, T., Kato, T., Date, M., Miyamoto, Z., Zhao, K., et al. (2005). Production of infectious hepatitis C virus in tissue culture from a cloned viral genome. Nat. Med. 11 (7), 791–796. doi:10.1038/nm1268
Bhandari, M. R., Jong-Anurakkun, N., Hong, G., and Kawabata, J. (2008). α-Glucosidase and α-amylase inhibitory activities of Nepalese medicinal herb Pakhanbhed (Bergenia ciliata, Haw.). Food Chem. 106 (1), 247–252. doi:10.1016/j.foodchem.2007.05.077
Hermeking, H. (2007). p53 enters the microRNA world. Cancer Cell 12 (5), 414–418. doi:10.1016/j.ccr.2007.10.028
Alves, M. G., Martins, A. D., Cavaco, J. E., Socorro, S., and Oliveira, P. F. (2013). Diabetes, insulin-mediated glucose metabolism and Sertoli/blood-testis barrier function. Tissue barriers. 1 (2), e23992. doi:10.4161/tisb.23992
Alves, M. G., Martins, A. D., Rato, L., Moreira, P. I., Socorro, S., and Oliveira, P. F. (2013). Molecular mechanisms beyond glucose transport in diabetes-related male infertility. Biochimica Biophysica Acta (BBA) - Mol. Basis Dis. 1832 (5), 626–635. doi:10.1016/j.bbadis.2013.01.011
Wild, S., Roglic, G., Green, A., Sicree, R., and King, H. (2004). Global prevalence of diabetes. Diabetes care 27 (5), 1047–1053. doi:10.2337/diacare.27.5.1047
Agbaje, I., Rogers, D. A., McVicar, C. M., McClure, N., Atkinson, A. B., Mallidis, C., et al. (2007). Insulin dependant diabetes mellitus: Implications for male reproductive function. Hum. Reprod. 22 (7), 1871–1877. doi:10.1093/humrep/dem077
Eisenberg, M. L., Chen, Z., Ye, A., and Buck Louis, G. M. B. (2015). Relationship between physical occupational exposures and health on semen quality: Data from the longitudinal investigation of fertility and the environment (LIFE) study. Fertil. Steril. 103 (5), 1271–1277. doi:10.1016/j.fertnstert.2015.02.010
Lotti, F., Corona, G., Castellini, G., Maseroli, E., Fino, M. G., Cozzolino, M., et al. (2016). Semen quality impairment is associated with sexual dysfunction according to its severity. Hum. Reprod. 31 (12), 2668–2680. doi:10.1093/humrep/dew246
Leeson, P. D., and Springthorpe, B. (2007). The influence of drug-like concepts on decision-making in medicinal chemistry. Nat. Rev. Drug Discov. 6 (11), 881–890. doi:10.1038/nrd2445
Amino, N., Ideyama, Y., Yamano, M., Kuromitsu, S., Tajinda, K., Samizu, K., et al. (2006). YM-201627: An orally active antitumor agent with selective inhibition of vascular endothelial cell proliferation. Cancer Lett. 238 (1), 119–127. doi:10.1016/j.canlet.2005.06.037
Shreedhara, S. H., Vagdevi, H. M., Jayanna, N. D., Raghavendra, R., Kiranmayee, P., Das, P., et al. (2017). The in vitro cytotoxic and molecular docking studies of newly synthesized fused benzoxazole-triazole derivatives. R. J Chem. Pharm. Res. 9 (5), 108–119.
Han, X., Pin, S. S., Burris, K., Fung, L. K., Huang, S., Taber, M. T., et al. (2005). Synthesis and structure-activity relationship of imidazo[1,2-a]benzimidazoles as corticotropin-releasing factor 1 receptor antagonists. Bioorg. Med. Chem. Lett. 15 (18), 4029–4032. doi:10.1016/j.bmcl.2005.06.028
Perlovich, G. L., Proshin, A. N., Volkova, T. V., Petrova, L. N., and Bachurin, S. O. (2012). Novel 1,2,4-thiadiazole derivatives as potent neuroprotectors: Approach to creation of bioavailable drugs. Mol. Pharm. 9 (8), 2156–2167. doi:10.1021/mp300011r
Fang, Y., Zhu, Z. L., Xu, P., Wang, S. Y., and Ji, S. J. (2017). Aerobic radical-cascade cycloaddition of isocyanides, selenium and imidamides: Facile access to 1,2,4-selenadiazoles under metal-free conditions. Green Chem. 19 (7), 1613–1618. doi:10.1039/c6gc03521c
Savjani, K. T., Gajjar, A. K., and Savjani, J. K. (2012). Drug solubility: Importance and enhancement techniques. Int. Sch. Res. Notices 2012, 195727. doi:10.5402/2012/195727
Chen, J. Y., Selvaraju, M., Lin, Y. T., Dhole, S., Lin, C. Y., and Sun, C. M. (2020). Molecular iodine-promoted [3 + 2] oxidative cyclization for the synthesis of heteroarene-fused [1,2,4] thiadiazoles/selenadiazoles. J. Org. Chem. 85 (8), 5570–5579. doi:10.1021/acs.joc.0c00421
Khan, A. A., Rahim, F., Taha, M., Rehman, W., Iqbal, N., Wadood, A., et al. (2022). New biologically dynamic hybrid pharmacophore triazinoindole-based-thiadiazole as potent α-glucosidase inhibitors: In vitro and in silico study. Int. J. Biol. Macromol. 199, 77–85. doi:10.1016/j.ijbiomac.2021.12.147
Taha, M., Tariq Javid, M. T., Imran, S., Selvaraj, M., Chigurupati, S., Ullah, H., et al. (2017). Synthesis and study of the α-amylase inhibitory potential of thiadiazole quinoline derivatives. Bioorg. Chem. 74, 179–186. doi:10.1016/j.bioorg.2017.08.003
Mrabti, N. N. (2018). QSAR study and molecular docking of benzimidazole derivatives inhibitors of p38 kinase. Moroc. J. Chem. 6 (3), 6–3.
Li, Z., Gu, J., Zhuang, H., Kang, L., Zhao, X., and Guo, Q. (2015). Adaptive molecular docking method based on information entropy genetic algorithm. Appl. Soft Comput. 26, 299–302. doi:10.1016/j.asoc.2014.10.008
Rao, C. M. M. P., Naidu, N., Priya, J., Rao, K. P. C., Ranjith, K., Shobha, S., et al. (2021). Molecular docking and dynamic simulations of benzimidazoles with beta-tubulins. Bioinformation 17 (3), 404–412. doi:10.6026/97320630017404
Khan, S., Ullah, H., Rahim, F., Nawaz, M., Hussain, R., and Rasheed, L. (2022). Synthesis, in vitro α-amylase, α-glucosidase activities and molecular docking study of new benzimidazole bearing thiazolidinone derivatives. J. Mol. Struct. 1269, 133812. doi:10.1016/j.molstruc.2022.133812
Rahim, F., Zaman, K., Taha, M., Ullah, H., Ghufran, M., Wadood, A., et al. (2020). Synthesis, in vitro alpha-glucosidase inhibitory potential of benzimidazole bearing bis-Schiff bases and their molecular docking study. Bioorg. Chem. 94, 103394. doi:10.1016/j.bioorg.2019.103394
Ullah, H., Uddin, I., Misbah, F., Khan, M., Taha, F., Rahim, M., et al. (2021). Synthesis of substituted benzohydrazide derivatives: In vitro urease activities and their molecular docking studies. Chem. Data Collect. 36, 100778. doi:10.1016/j.cdc.2021.100778
Keywords: synthesis, 4-benzodioxin, thiadiazole-fused-[1,4]-thiadiazole, α-amylase, α-glucosidase, molecular docking
Citation: Hussain R, Shah M, Iqbal S, Rehman W, Khan S, Rasheed L, Naz H, Al-ghulikah HA, Elkaeed EB, Pashameah RA, Alzahrani E and Farouk A-E (2022) Molecular iodine-promoted oxidative cyclization for the synthesis of 1,3,4-thiadiazole-fused- [1,2,4]-thiadiazole incorporating 1,4-benzodioxine moiety as potent inhibitors of α-amylase and α-glucosidase: In vitro and in silico study. Front. Chem. 10:1023316. doi: 10.3389/fchem.2022.1023316
Received: 19 August 2022; Accepted: 12 September 2022;
Published: 06 October 2022.
Edited by:
Kaushik Chanda, VIT University, IndiaReviewed by:
Ching-Feng Weng, National Dong Hwa University, TaiwanXuben Hou, Shandong University, China
A. Ganesan, University of East Anglia, United Kingdom
Copyright © 2022 Hussain, Shah, Iqbal, Rehman, Khan, Rasheed, Naz, Al-ghulikah, Elkaeed, Pashameah, Alzahrani and Farouk. This is an open-access article distributed under the terms of the Creative Commons Attribution License (CC BY). The use, distribution or reproduction in other forums is permitted, provided the original author(s) and the copyright owner(s) are credited and that the original publication in this journal is cited, in accordance with accepted academic practice. No use, distribution or reproduction is permitted which does not comply with these terms.
*Correspondence: Shahid Iqbal, shahidgcs10@yahoo.com; Wajid Rehman, sono_waj@yahoo.com