Multifunctional Nanomaterials for Ferroptotic Cancer Therapy
- 1Department of Urology, Xiang’an Hospital of Xiamen University, School of Medicine, Xiamen University, Xiamen, China
- 2School of Public Health, Xiamen Univerisity, Xiamen, China
- 3Central Laboratory, Xiang’an Hospital of Xiamen University, School of Medicine, Xiamen University, Xiamen, China
Patient outcomes from the current clinical cancer therapy remain still far from satisfactory. However, in recent years, several biomedical discoveries and nanotechnological innovations have been made, so there is an impetus to combine these with conventional treatments to improve patient experience and disease prognosis. Ferroptosis, a term first coined in 2012, is an iron-dependent regulated cell death (RCD) based on the production of reactive oxygen species (ROS) and the consequent oxidization of polyunsaturated fatty acids (PUFAs). Many nanomaterials that can induce ferroptosis have been explored for applications in cancer therapy. In this review, we summarize the recent developments in ferroptosis-based nanomaterials for cancer therapy and discuss the future of ferroptosis, nanomedicine, and cancer therapy.
1 Introduction
Cancer is one of the leading causes of death and one of the most important diseases affecting human health worldwide (Bray et al., 2021). According to the recent global cancer statistics, there were over 19 million new cancer cases and 10.0 million cancer deaths in 2020, and the cancer burden might be expected to reach 28.4 million cases by 2040 (Sung et al., 2021). Therefore, the development of cancer prevention and treatment strategies is crucial to achieve global cancer control. Conventional approaches, such as surgery, radiotherapy, and chemotherapy, have been utilized; however, the prognosis does not achieve both the doctor’s and patient’s satisfaction due to the hallmarks of cancer, such as sustaining proliferative signaling, evading growth suppressors, nonmutational epigenetic reprograming, avoiding immune destruction, activating invasion and metastasis, inducing vasculature, genome instability and mutation, resisting cell death, and deregulating cellular metabolism (Hanahan 2022). Accompanied with the significant scientific and technological advancements in recent times, several new technologies are currently under research in clinical trials, and some of them have already been approved for clinical application, including stem cell therapy, targeted therapy, ablation therapy, photodynamic therapy (PDT), photothermal therapy (PTT), chemodynamic therapy (CDT), sonodynamic therapy (SDT), and ferroptosis-based therapy (FBT) (Debela et al., 2021).
Ferroptosis is a novel type of regulated cell death (RCD) that is iron-dependent and characterized by the oxidization of polyunsaturated fatty acids (PUFAs) and subsequently accumulation of lipid peroxides (LPOs) (Figure 1) (Shi et al., 2021). During the past two decades, Brent R. Stockwell et al. focused on ferroptosis, and their research studies have attracted global attention. Specifically, in 2003, they identified a novel compound and named it “erastin” (Dolma et al., 2003). Intriguingly, they found that although cell death occurred, characteristics of apoptosis were not observed when erastin was present in several tumor cells. In 2007, they found that erastin-induced cell death could be suppressed by α-tocopherol (Yagoda et al., 2007). In 2008, they found another small compound, Ras selective lethal 3 (RSL3) that induced analogous iron-dependent nonapoptotic cell death in RAS-enriched cancer cells, and this could also be restrained by the presence of α-tocopherol or desferrioxamine mesylate (DFOM) (Yang and Stockwell, 2008). In 2011, they confirmed that erastin- and RSL3-induced cell death differed from the mechanism of other cell deaths (Wolpaw et al., 2011). Therefore, in 2012, they named this phenomenon ‘ferroptosis’ (Dixon et al., 2012). Ferroptosis is distinct from apoptosis, autophagy, and necroptosis in terms of morphology, biochemistry, and genetics (Cao and Dixon, 2016). Cells experiencing ferroptosis show special hallmarks, including the fracture of the cell membrane, smaller mitochondria, increased density of the mitochondrial membrane, reduced/vanished mitochondrial cristae, and outer mitochondrial membrane breakup; however, the nuclei remain normal (Li, J. et al., 2020).
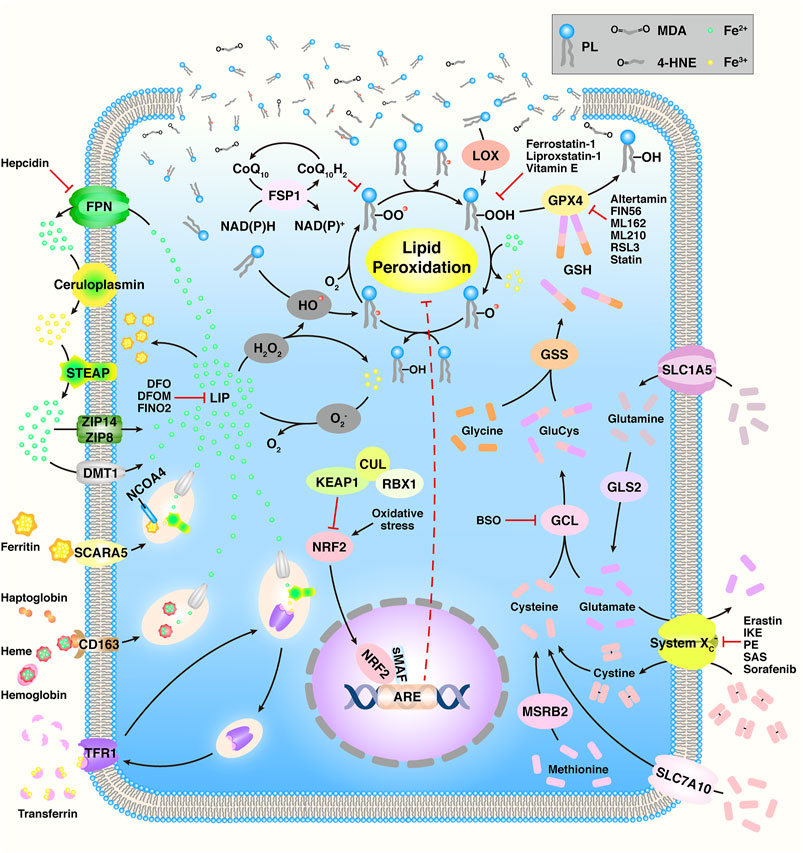
FIGURE 1. Overview of the ferroptosis mechanism. Adapted from Shi et al., (2021) Copyright @ 2021 (Frontiers).
Many nanotechnologists have focused on cancer therapy in recent years because of the promising physicochemical properties of nanomaterials (Liang et al., 2019), and an increasing number of biomedical nanomaterials have been widely investigated as nanomaterial-based drug delivery systems for anticancer therapeutics, with enhanced drug availability, improved efficacy, targeted delivery, and reduced treatment-related toxicity, which could better solve the disadvantages of anticancer drugs with low solubility and poor membrane permeability (Luo et al., 2014; Asghari et al., 2019; Fei et al., 2020a). Nanomaterials possess an exceptional advantage of the enhanced permeability and retention (EPR) effect, which is related to anatomical and pathophysiological differences between the tumor tissue and normal tissue. Crucially, the solid tumors are highly vascularized and have large gaps between the endothelial cells in tumor vessels; as a result, macromolecular drugs are selectively extravasated and retained by the tumor tissues (Fang et al., 2011). Nevertheless, the EPR effect can be influenced by the systolic blood pressure and is negligible in advanced cancers (Maeda 2015; Golombek et al., 2018; Shan et al., 2020). In addition to the EPR effect, several strategies were taken into advantage by the researchers, such as modifying targeting molecules, remodeling the tumor microenvironment (TME), and enabling tumor stimuli–responsive properties (Shi et al., 2017; Youn and Bae, 2018; Park et al., 2019). Thus, nanomaterials can be used as delivery agents for anticancer drugs, but they can also be used to convert energy to kill cancer cells, such as light, ultrasound (US), electricity, and magnetothermal energy (Fei et al., 2020a). As a result, in the last few years, the Food and Drug Administration (FDA) has approved a large number of anticancer nanomaterials, demonstrating the huge potential of nanomaterials for the precision and personalized cancer therapies in future.
Ferroptosis, as a novel RCD, has become a popular area of cancer research, and, thus, numerous nanomaterials that induce ferroptosis have been developed for cancer therapy because of their potential antitumor properties. In this review, we try to summarize and discuss the multifunctional ferroptosis-based nanomaterials on the recent advances and breakthroughs in cancer therapies.
2 FERROPTOSIS INDUCERS (FINs)
The FINs can be classified into four types (Figure 2). Class I FINs could block the biosynthesis of glutathione (GSH) or deplete it. Many small compounds (such as erastin, imidazole ketone erastin, piperazine erastin, sulfasalazine, and sorafenib) can inhibit system Xc−, which could transfer cystine into the cells and export glutamate out of the cells (Feng and Stockwell, 2018). The system Xc− consists of solute carrier family 7 member A11 (SLC7A11, also known as xCT) and SLC3A2 (4F2hc) (Shi et al., 2021). GSH is a cofactor of GSH peroxidase 4 (GPX4), which is a crucial regulator for the conversion of LPOs to the corresponding hydroxyl compounds (LOH). Therefore, the depletion of GSH can inactivate GPX4, subsequently resulting in the accumulation of LPOs to induce ferroptosis. Class II FINs directly inhibit GPX4. Small compounds (such as RSL3, ML162, ML210, and altretamine) can interact with the active selenocyteine site of GPX4 and inhibit its enzymatic activity, resulting in the accumulation of LPOs (Cao and Dixon 2016). Class III FINs deplete the GPX4 protein. For example, the compounds FIN56 and caspase-independent lethal 56 (CIL56) could induce ferroptosis by directly degrading the GPX4 and synchronously depleting the mevalonate-derived coenzyme Q10 (CoQ10) (Shimada et al., 2016). Class IV FINs could induce lipid peroxidation, but there is only one small compound, FIN endoperoxide (FINO2), that can directly oxidize iron, indirectly inactivate GPX4, and ultimately cause lipid peroxidation (Gaschler et al., 2018). This compound is suitable in vitro; however, it might not be effective in vivo.
3 Nanomaterials as FINs
The design of the multifunctional nanomaterials with precisely tuned physicochemical properties could enhance the drug delivery for cancer therapy. The first ferroptosis-inducing nanomaterial was reported by Kim et al. in 2016 (Figure 3) (Kim et al., 2016). They coated the near-infrared (NIR) fluorescent silica nanoparticles (NPs) with ploy (ethylene glycol)-coated (PEGylated) and Cornell dots (C′ dots) and surface-modified with the melanoma-targeting peptide and alpha-melanocyte stimulating hormone (αMSH). The C′ dots could absorb and incorporate extracellular iron into their structure to increase the intracellular iron level with the subsequent production of reactive oxygen species (ROS) and depletion of GSH, ultimately leading to ferroptosis in different cancer models. The research laid the groundwork for investigating nanomaterials as effective FINs in sensitive tumors.
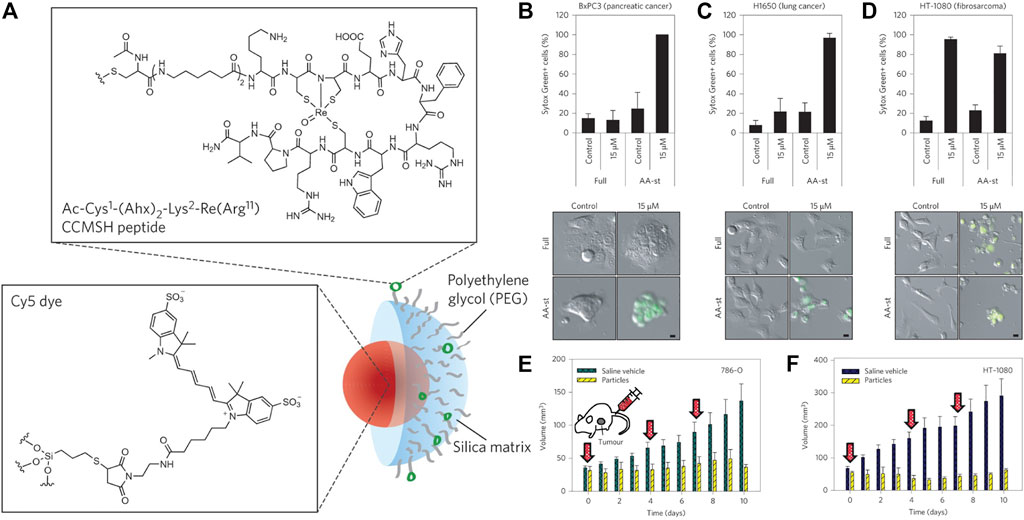
FIGURE 3. (A) Synthesis scheme of αMSH-PEG-C′ dots. (B–D) Cell death about BxPC3 cells with different treatments (B), H1650 cells (C), and HT-1080 cells (D). (E) 786-O and (F) HT-1080 tumor volume with different treatments. Reproduced with permission from Kim et al., (2016) Copyright @ 2016 (Springer Nature).
3.1 Iron-Contained Nanomaterials
Owing to the crucial role of iron in ferroptosis, iron-contained nanomaterials have become the most popular nanomaterials for FBT. In recent years, several types of iron-contained nanomaterials have been explored to induce ferroptosis.
3.1.1 Iron Ion–Based Nanomaterials
The different valence states of iron have been exploited to develop nanomaterials that act as FINs to kill cancer cells. In the zero-valent iron NP (ZVI NPs)–sensitive cancer cells, Fe0 could be oxidized to Fe2+ (Huang et al., 2019). The increased iron induced intracellular ROS surge via Fenton reaction, followed by the accumulation of mitochondrial LPOs. The monodispersed ferrihydrite NPs were synthesized and demonstrated the light-triggered Fe2+ generation at the tumor sites (Yang, Y. et al., 2021). Ferrihydrite NPs, 20∼30 nm, could release a large amount of Fe2+ to promote the iron/ROS-related irreversible DNA fragmentation and GPX4 inhibition under a common blue light illumination. In addition, it simultaneously induced polarization of the tumor-associated macrophage (TAM) from the tumor-promoting M2 phenotype to the tumor-killing M1 type, which concomitantly inhibited the tumor growth and prevented lung metastasis under illumination in vivo. The sulfasalazine and disulfide-bridged levodopa (DSSD) assembled as sulfasalazine@DSSD that could chelate Fe2+ to form the prodrug nanosystem sulfasalazine–Fe2+@DSSD (Xin et al., 2021). The disulfide bonds could consume GSH in tumor cells, triggering the leakage of sulfasalazine and Fe2+. Fe2+ induced ferroptosis via the Fenton reaction, and this was enhanced by the presence of sulfasalazine to improve the therapeutic efficacy. In another system, doxorubicin (DOX) was chelated with Fe2+, yielding Fe2+-DOX, which was condensed with calcium-containing precursors to form amorphous calcium carbonate (ACC)–encapsulated Fe2+-DOX cores via a one-step synthetic approach (Figure 4) (Xue et al., 2020). The nanoplatform was simultaneously modified with matrix metalloproteinase-2 (MMP-2)–sheddable PEG and folic acid (FA) to achieve circulation stability and targeting capability. The therapeutic nanomaterial could release Fe2+ that produced H2O2 and DOX that amplified the effect of Fe2+ under a pH-triggered and self-regulated manner.
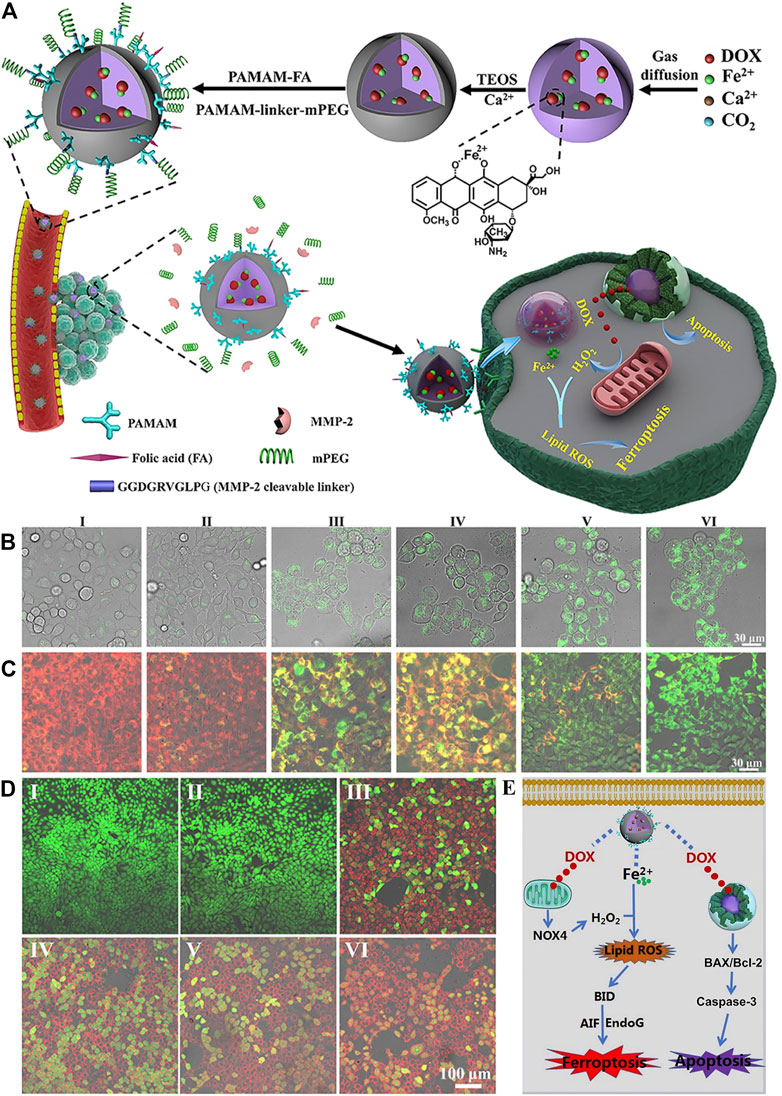
FIGURE 4. (A) Synthesis scheme of ACC@DOX-Fe2+-Ca-FA/mPEG and its therapeutic action. (B–D) Confocal laser scanning microscopy observation in 4T1 tumor cells about the intracellular distribution of the LPOs (B), the changes in the mitochondrial membrane potential (C) and apoptosis levels (D) after treatment with (I) PBS, (II) ACC-Ca-FA/mPEG, (III) DOX, (IV) ACC@DOX-Ca-FA/mPEG, (V) ACC@DOX-Fe2+-Ca-FA/mPEG, and (VI) MMP-2–treated ACC@DOX-Fe2+-Ca-FA/mPEG. (E) Molecular mechanism for ACC@DOX-Fe2+-Ca-FA/mPEG-induced ferroptosis/apoptosis. Reproduced with permission from Xue et al., (2020) Copyright @ 2020 (AAAS).
Recently, Cui et al. prepared a nanoenzyme in which Fe3+ was substantially reserved in the polypyrrole NPs (FePPy NPs) (Cui et al., 2021). The FePPy NPs generated hydroxyl radicals (•OH) by the degradation of H2O2 to induce ferroptosis. Upon irradiation, the FePPy NPs induced low-temperature PTT, which accelerated the Fenton reaction and enhanced ferroptosis and photoacoustic imaging (PAI) in vitro and in vivo. In addition, nanomaterials based on Fe3+ and tannic acid (TA) with the DOX-loaded dendrimers could combat multidrug resistance (MDR) in cancer cells via the apoptosis/ferroptosis pathway (Guo et al., 2019). The DOX-induced apoptosis elevated the intracellular ROS levels, thus sensitizing cancer cells to ferroptosis. The efficiency of CDT, in which H2O2 is decomposed into toxic •OH in tumor cells, was improved. Chen H. et al. reported a nanodrug delivery system based on TA, Pluronic F-68, and Fe3+ (Chen, H. et al., 2021). DOX, Pluronic F-68, and TA were first assembled to form DOX@Pluronic F-68/TA by π–π interactions and hydrophobic bonds. Subsequently, Fe3+ was introduced onto the surface of DOX@Pluronic F-68/TA to form DOX@Pluronic F-68/TA/Fe3+ via elaborate coordination interactions. The nanodrug DOX@Pluronic F-68/TA/Fe3+ efficiently targeted the tumor and primely suppressed tumor growth. In addition, the hollow mesoporous silica NP (MSNP)–loaded ferrate and DOX were followed by incorporating n-heneicosane (Fu et al., 2021). The mild hyperthermia induced by US initiated the phase change of n-heneicosane and enabled the co-release of ferrate and DOX. The ferrate then reacted with H2O to form H2O2, which released O2, thus causing TME-independent reoxygenation and downregulation of the expression of hypoxia-inducible factor 1α and MDR gene/transporter P-glycoprotein in tumor cells. Overall, the cooperation of chemotherapy and FBT resulted in enhanced suppression of hypoxic osteosarcoma growth in vivo.
Dopamine can be used to load hydrophobic drugs and metal ions due to its aromatic rings and catechol groups (Cheng et al., 2019). Furthermore, polydopamine (PDA) shows strong absorption in the NIR region at approximately 808 nm and can efficiently convert the light energy into thermal energy (Rahim et al., 2019;Yamagishi et al., 2019). PDA nanosubstrates could efficiently load Fe2+ and β-lapachone, which initiated ferroptosis in the tumor cells subjected to NIR illumination (Xue et al., 2021). Crucially, cellular hyperthermia was generated by PDA nanostructures under NIR irradiation and triggered the release of Fe2+. The NIR-enabled PTT also activated heat shock response and upregulated NADPH: quinone oxidoreductase protein (NQO1) via the heat shock protein 70 (HSP70)/NQO1 axis to promote bioreduction of β-lapachone and boost the intracellular H2O2 levels to facilitate the Fe2+-dependent lipid peroxidation, while minimizing the potential damage to normal tissues (Figure 5) (Xue et al., 2021). The Fe2+ and Fe3+ also could be loaded into the core of the ultrasmall PEG-modified PDA NPs (UPDA-PEG NPs, 8.4 nm), thus yielding a novel ferroptosis agent (Chen et al., 2019). Seventy percent of the iron ions could be released at pH 5.0, suggesting applications in the acidic TME. The ferroptosis induced by UPDA-PEG@Fe2+ NPs was dependent on ROS, whereas UPDA-PEG@Fe3+ NPs induced LPO-dependent ferroptosis. In another research, DOX was loaded onto the PDA NPs to achieve a synergistic effect (Nieto et al., 2021). The pH determined the Fe3+ state and the selectivity and therapeutic activity of the resultant Fe3+-loaded PDA NPs. Furthermore, the antitumor activity could be enhanced by DOX, which also increased ROS production in the cancer cells, and PDA NPs with different concentrations of Fe3+ and drugs could be tailor-synthesized and administered depending on the therapeutic need. The Fe3+ and the catechol of dopamine molecules could self-polymerize Fe3+-PDA cores with an Fe loading efficiency of 5.3% (Chen et al., 2020). Subsequently, the hyaluronic acid (HA) cross-linked cisplatin shells were constructed onto the Fe3+-PDA cores, and the core-shell nanomaterial was highly sensitive to an acidic or reductive TME, while its disassembly led to the continuous release of cisplatin and Fe3+. The cisplatin could enhance ferroptosis via activating the NADPH oxidases (NOXs) and suppressing GPX4. Thus, the core-shell nanomaterial provided a new antitumor strategy for synergistic chemotherapy/FBT/PTT with a low dosage.
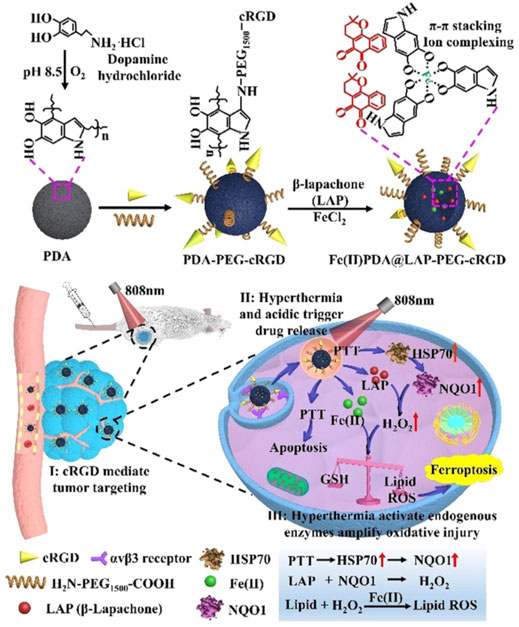
FIGURE 5. Synthesis scheme of the Fe2+PDA@LAP-PEG-cRGD nanomaterial and mechanism of PTT/FBT for cancer. Adapted from Xue et al., (2021) Copyright @ 2021 (Wiley).
Iron ions could also be coordinated to some nanomaterials. For example, Jiang Y. et al. reported a hybrid semiconducting nanozyme (HSN) possessing high photothermal (PT) conversion efficiency and NIR-II PAI-guided PTT/FBT (Jiang, Y. et al., 2020). HSN comprised the amphiphilic semiconducting polymer as a PT converter, PA emitter, and iron-chelating Fenton catalyst. Upon NIR-II irradiation, HSN induced PT transduction, which triggered PTT and potentiated the Fenton reaction. Furthermore, •OH transformed HSN to approximately 1.7 nm–sized fragments. The noninvasive therapy possesses several advantages, such as deep ablation, reduced expression of metastasis-related proteins, and inhibition of distant metastasis. Recently, artemisinin and its derivatives have been investigated as potential FINs for cancer treatment. The nanocarrier-containing artemisinin was based on TA and Fe2+ coated on the zeolitic imidazolate framework-8 (ZIF-8) (Li, Z. et al., 2021). After 10 h, 59% artemisinin was released from TA-Fe2+/artemisinin@ZIF-8 in pH 5.0. The intracellular ROS production and GPX4 inhibition could lead to suppression of triple-negative breast cancer growth.
3.1.2 Iron‐Based Metal‐Organic Frameworks (MOFs)
The nanoscale MOFs formed by Fe2+ and 2-aminoterephthalic acid (BDC-NH2) possessed excellent stability and pH-responsive degradation to release Fe2+ in the acidic TME, which could catalyze the Fenton reaction and produce considerable quantities of ROS to induce Fe2+-mediated ferroptosis (Xu et al., 2020). The perfluoropentane@Fe/Cu-SS MOF is displayed as an effective FIN because it could increase the production of LPO through the Fenton reaction and inhibit the GPX4 that prevents LPO transfer to LOH in the presence of GSH, whereas the Fe3+ and Cu2+ in the MOF could consume GSH, further inhibiting GPX4 activity. Perfluoropentane@Fe/Cu-SS MOF also enabled T1-weighted magnetic resonance imaging (MRI), US imaging, and PTT (He, H. et al., 2020). Au1Ag24 nanoclusters and Fe3+ could be effortlessly assembled in an oil phase and modified by the vesicles of the PEG block grafted polyketal copolymer (PbP). The core-shell cancer theranostic nanomaterial Fe3+@Au1Ag24@PbP possessed several excellent multiproperties, including NIR laser/TME co-responsiveness and PAI/PT imaging-guided PTT/PDT/FBT both in vitro and in vivo (Cheng, H. et al., 2021). In addition, the iron-chelated hybrid polymeric NPs comprising Fe3+ and an amphiphilic semiconducting complex have been reported, in which these components acted as PT transducers and Fe3+ chelators, respectively (He, S. et al., 2020). In the acidic TME, Fe3+ was released to generate •OH; in addition, local heat was generated under the NIR laser irradiation to enhance the Fenton reaction and achieve PAI-guided PTT.
The addition of cancer cell membranes to nanomaterials enables homologous targeting and immune escaping capabilities. Wan et al. reported a nanomaterial containing Fe3+-based MIL-100 and glucose oxidase (GOx) that catalyzed the degradation of glucose to generate sufficient H2O2 for FBT (Wan et al., 2020). When the nanomaterial was obtained by the tumor cells, intracellular GSH triggered the disruption of MOF via reducing Fe3+ to release Fe2+ and GOx. The nanomaterial exhibited highly effective tumor inhibition and enabled precise collaborative tumor therapy with spatiotemporal controllability. Another MOF nanomaterial loading with GOx and DOX was also coated with the cancer cell membrane (denoted as mFe(SS)/DG) (Figure 6) (Yang, J. et al., 2021). The mFe(SS)/DG system based on Fe3+ and disulfide-bearing ligands depleted GSH and suppressed GPX4 activity to induce ferroptosis. The increasing ROS facilitated ferroptosis and restrained glycolysis. Thus, immunogenic cell death (ICD) induced by the synergistic effect of ferroptosis and DOX released tumor antigens to trigger antitumor immunity when the inhibition of glycolysis remodeled the tumor immunogenicity and immunosuppressive microenvironment to strengthen the antitumor immunity.
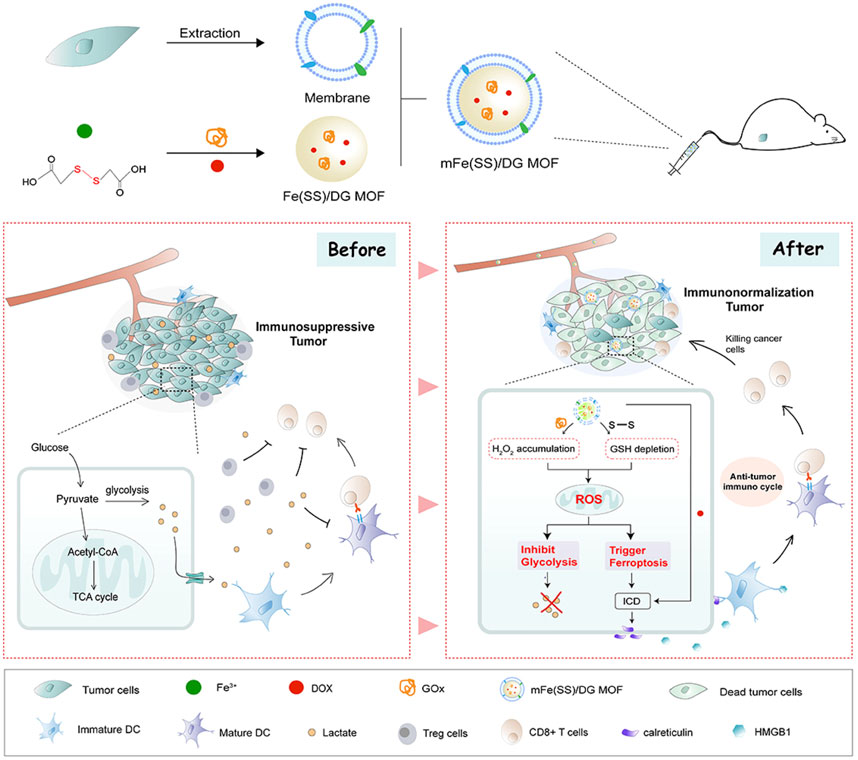
FIGURE 6. Schematic illustration of mFe (SS)/DG and the mechanism based on ferroptosis–glycolysis regulation. Adapted from Yang J et al., (2021); Yang Y et al., (2021) Copyright @ 2021 (Elsevier).
In addition, iron-based MOFs can be loaded with FINs to enhance ferroptosis. For example, MIL88B loaded with RSL3 forced M2 macrophages to increase the glycolytic metabolism (Gu et al., 2021). MIL88B/RSL3 downregulated the expression of M2-associated signaling pathways and activated M1-related signaling. The ferroptosis-strengthened macrophage regulation strategy had great potential for TAM-centered antitumoral applications. In the same year, the surface of MIL-101 (Fe)-loaded sorafenib was modified with an iRGD peptide that could bind to neruopilin-1 receptor yielding a nanodrug that induced ferroptosis of the hepatocellular carcinoma by consuming GSH, decreasing GPX4 expression, and enhancing LPO generation (Liu et al., 2021). Fe3+ and TA spontaneously formed a network-like corona onto the sorafenib nanocores that were loaded with methylene blue (Liu et al., 2018). The corona is disrupted in the lysosomal acid environment, whereas TA could reduce Fe3+ to Fe2+, paving the way for iron redox cycling to produce LPO. Furthermore, the concomitant methylene blue release induced fluorescence recovery, endowing the nanomaterials with multimodal imaging-guided PDT/FBT. In another example, Fe3+-containing MOFs loaded with piperlongumine (a FIN) were coated with a transferrin-decorated pH sensitive lipid layer to form the nanodrug (Xu, R. et al., 2021). Crucially, after treatment with this nanoagent, the cellular iron concentration increased dramatically due to the iron-containing MOF, and piperlongumine strengthened the ferroptosis via providing H2O2 for the dual induction system.
3.1.3 FePt-Based Nanomaterials
FePt-based nanomaterials have potential clinical applications in cancer therapy. For example, a ferroptosis nanotheranostic agent was designed by integrating FePt as the core, Eu3+-based luminescent molecule, and FA as the targeting molecule (Yue et al., 2018). FePt-based nanomaterials exhibited an excellent MRI, computed tomography (CT), and time-gated luminescence properties in the clinical diagnosis and had excellent capacity to trigger ferroptosis of cancer in vitro and in vivo.
The FePt NPs can also be loaded onto diverse nanomaterials to show their advantages. An acidic responsive nanoagent FePt@MnO@DSPE-PEG-FA NP was successfully constructed for MRI-guided FBT/CDT of cancer (Figure 7) (Yang et al., 2019). It could release active Fe2+ to elevate ROS production via the Fenton reaction to induce ferroptosis. In addition, Mn2+ could be released from the nanomaterial in the acidic TME and scavenge GSH to enhance the T1/T2-weighted MRI, which could obviously distinguish the solid tumors. Prussian blue (PB) nanocubes were utilized as PT agents, and FePt NPs were loaded onto the surface of the nanocube via in situ reduction (Hu et al., 2020). The surface of the nanocomposite PB@FePt was wrapped with HA and PEG, and the nanoagent PB@FePt–HA-g-PEG served as a multifunctional nanomaterial, enabling CDT/PTT and triple-modal imaging (MRI/CT/PT imaging) capability. Covalent organic polymers (COPs) possess specific surface area and good biocompatibility, making them promising nano delivery carriers (Shi et al., 2019). FePt@COP-FA with excellent PT potential was developed for cancer treatment (Meng et al., 2020). It could efficiently ablate the primary tumors with the presentation of NIR irradiation and release a mass of tumor-associated antigens in situ. With the assistance of anticytotoxic T-lymphocyte antigen-4 (anti-CTLA4), the specific immune response was triggered to suppress the growth of metastatic tumors. In particular, the synergistic therapy could form a valid immunological memory to further restrain tumor relapses. FePt NPs could also be loaded onto polyethylenimine (PEI)-modified ultrathin black phosphorus (BP) nanosheets, and this nanomaterial showed enhanced synergistic PTT/PDT/CDT for targeting primary tumors (Yao et al., 2020). More significantly, combined with the CTLA-4 checkpoint blockade, PTT induced by FePt/BP–PEI–FA could control the growth of both the primary and untreated distant tumor.
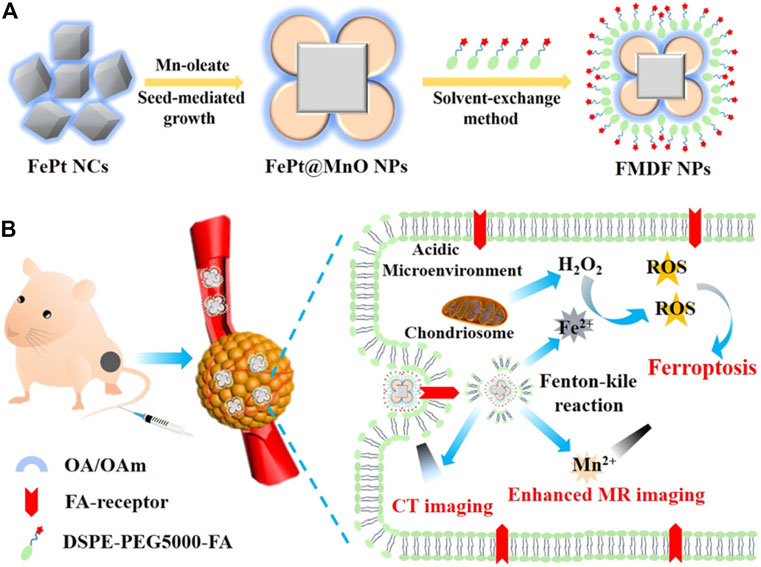
FIGURE 7. (A) Synthesis scheme of FMDF NPs. (B) Acidic-responsive and dual-model imaging-guided FBT/CDT. Adapted from Yang et al., (2019) Copyright @ 2019 (ACS).
3.1.4 Iron Oxide (IO)–Based Nanomaterials
IONPs, which have been approved by the FDA, have been widely applied in cancer therapy because they produce ROS via the Fenton reaction.
An innovative local chemotherapy combined with a gene therapy–based IONP was developed as a treatment for patients with glioblastoma (Zhang et al., 2020). The porous structure of IONPs attached -COOH groups and could encapsulate the small interfering RNA (siRNA) targeting GPX4 (siGPX4) and cisplatin. After uptake, the IONPs degraded and released Fe2+/Fe3+, and the H2O2 level increased owing to the activating reduced NOX. Furthermore, the cisplatin destroyed both nuclear and mitochondrial DNA, while siGPX4 restrained GPX4 expression. The manganese-deposited IO (FMO) NPs were loaded with the cisplatin prodrug Pt (IV) to form Pt-FMO, which was modified with PEG to increase colloidal stability (Cheng, J. et al., 2021). FMO released Mn2+ and Fe2+/Fe3+ in the weakly acidic TME, and the Pt (IV) could deplete GSH to generate cisplatin. Mn2+ enhanced the ferroptosis induced by Fe2+/Fe3+ released from FMO, and cisplatin elevated the intracellular H2O2 levels, which obviously strengthened ferroptosis via the Fenton reaction. Therefore, the synergism between the ferroptosis induced by FMO and the apoptosis induced by cisplatin resulted in tumor ablation.
Biomimetic magnetic NPs coated with platelets can make the cancer cells sensitize to ferroptosis, generate mild immunogenicity, and improve the response rate of noninflammatory tumors to immunotherapy (Jiang, Q. et al., 2020). Thus, a Fe3O4-sulfasalazine@platelet system was designed by using mesoporous magnetic Fe3O4 NPs, sulfasalazine, and platelet membranes. Fe3O4–sulfasalazine@platelet-mediated ferroptosis could evidently improve the potency of programed cell death 1 (PD-1) and achieve persistent tumor elimination in 4T1 metastatic tumors. Moreover, the Fe3O4–sulfasalazine@platelet-mediated ferroptosis also repolarize macrophages from the M2 phenotype to M1 phenotype. The magnetosomes contained Fe3O4 magnetic nanoclusters and pre-engineered the leukocyte membranes as the core and shell, and the transforming growth factor-β (TGF-β) inhibitor and PD-1 antibody were, respectively, loaded inside and on the surface of the membrane (Figure 8) (Zhang et al., 2019). The membrane camouflage endowed the nanomaterial with a long circulation time, and the nanocluster core enabled magnetic targeting under MRI guidance. When the nanomaterial reached the tumor site, the cooperation of the TGF-β inhibitor and PD-1 antibody increased the rates of CD4+ T/Treg cells, CD8+ T/Treg cells, and M1/M2 macrophages to create an immunogenic TME, and the elevated H2O2 from M1 polarization enhanced the Fenton reaction. The production of •OH subsequently induced ferroptosis of the cancer cells and the released tumor antigens, thus increasing TME immunogenicity.
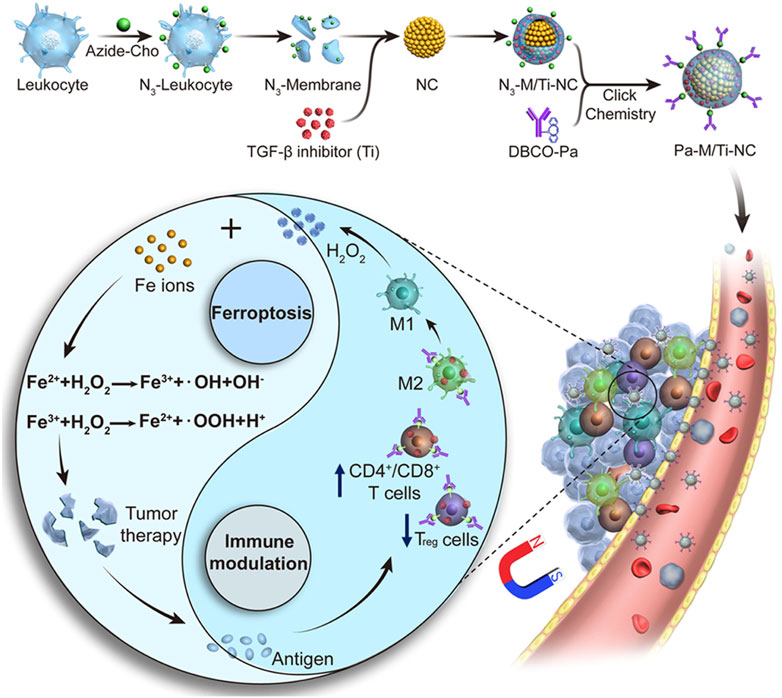
FIGURE 8. Synthesis scheme and mechanism of the magnetosome for FBT/immunomodulation in cancer. Adapted from Zhang et al., (2019) Copyright @ 2019 (ACS).
IONPs can also be loaded onto or into different nanomaterials. Superparamagnetic IONPs (SPIONPs) are considered excellent carriers to deliver nucleic acid and drug because of the controllable properties, outstanding stability, and easy modification. The sorafenib and ultrasmall SPIONPs (approximately 8.5 nm) were, respectively, loaded onto the mesopores of mesoporous PDA (MDPA) NPs and onto the surface of the MDPA NPs to form sorafenib@MPDA-SPIONP (Guan et al., 2020). The heat generated by the MPDA NPs upon NIR laser irradiation boosted the effect of ferroptosis. Furthermore, the application of moderate heat (45°C) in the presence of the IONPs could significantly suppress the expression of the antioxidative proteins and cause tumor cells to undergo specific lipid metabolism (Xie et al., 2021). By virtue of the IONPs and 1H-perfluoropentane (1H-PFP), a heat-responsive ferroptosis strategy was rationally designed by utilizing a polypeptide-modified, IO-containing nanomaterial that loaded 1H-PFP. The phase transition of 1H-PFP was triggered under 808-nm laser irradiation, which led to the release of IONPs in situ to produce ROS in the TME. The inhibition of GSH synthesis restrained the antioxidant response of tumors due to heat stress. Furthermore, the combined treatments reprogramed the lipid metabolism by generating a large amount of LPO and inducing acyl-CoA synthetase bubblegum family member 1 (ACSBG1)–dependent ferroptosis in the C4-2 tumor model. More recently, the polypeptide vehicle-based theranostics (Pt&IONPs@PP) were self-assembled by mixing poly (L-glutamic acid) (PGA), poly (L-lysine) (PLL), Pt prodrug, and carboxyl-modified IONPs through electrostatic interactions, and the surface of this nanomaterial was modified with PEG (Gao et al., 2020). The acidic TME degraded Pt&IONPs@PP to release Pt drug and Fe2+/Fe3+. Moreover, Pt&IONPs@PP could induce T2-weighted, MRI-guided chemotherapy/FBT to inhibit cancer cell growth without systemic toxicity in vitro and in vivo.
A nanosystem containing Fe3O4 and chlorin E6 (Ce6) was designed for the synergistic cancer therapy (Chen, Q. et al., 2021). The poly (lactic-co-glycolic acid) (PLGA) was used as the shell to load Fe3O4 and Ce6, which degraded in the acidic TME and released Fe2+/Fe3+ and Ce6. The reaction between Fe2+/Fe3+ and intracellular H2O2 produced •OH to induce ferroptosis of tumor cells. The released Ce6 could increase the ROS levels upon laser irradiation to offer PDT, and this boosted the ferroptosis effect in 4T1 tumors. A gel-delivery platform with embedded Au nanorods (AuNRs) and IONPs was reported, the so-called AuNRs&IONPs@Gel, for bladder cancer therapy (Guo, P. et al., 2020). The gel platform was based on a dextran aldehyde that could selectively adhere with the cancer collagen. AuNRs could induce imaging-guided PTT under NIR radiation, and the locally high concentration of IONPs induced ferroptosis. Moreover, TAMs would be repolarized by the IONPs from the M2 phenotype into M1 phenotype to achieve a direct antitumor effect and antigen presentation of dead cells. This process triggered an effective innate and adaptive immune response to protect against tumor rechallenge in the long term. The black hole quencher (BHQ)–based fluorescence ‘off−on’ nanomaterials were self-assembled with chitosan oligosaccharide (CSO), IR780, hexadecane (Hex), magnetic IONPs (MIONPs), and sorafenib to form CSO-BHQ-IR780-Hex/MIONPs/sorafenib (Sang et al., 2019a). BHQ and IR780 were combined via a GSH-responsive ether bond. After uptake, the CSO–BHQ–IR780–Hex NPs collapsed and IR780–Hex anchored the mitochondrial membrane. When NIR irradiation was applied to the NPs, the iron level increased, and the xCT/GSH/GPX4 system was triggered. In addition, a similar nanomaterial (CSO–SS–Cy7–Hex/SPIONP/sorafenib) was developed to overcome the therapy-resistant state of cancer (Sang et al., 2019b). This system could scavenge GSH and increase the concentration of iron and LPO, and the ferroptosis induced by CSO-SS-Cy7-Hex/SPIONP/sorafenib complex defeated MDR, reduced invasion, and limited the metastasis of cancers undergoing the epithelial-to-mesenchymal transition.
The IO-hydroxide (IOOH) nanospindles have been used as MRI contrast agents for cancer diagnosis; thus, a biocompatible H2S-responsive IOOH nanospindle for colon cancer is developed (Li, Y. et al., 2020). The IOOH nanospindles, synthesized from Fe3+ and dopamine and modified by PEG, could efficiently deplete endogenous H2S via the reduction reaction to inhibit CT26 colon cancer growth. Crucially, the overproduction of H2S drove a cascade reaction producing FeS; thus, this system could be used for NIR-responsive PTT and Fe2+-induced ferroptosis.
3.1.5 Iron-Containing Organic Transition Metal Compound–Based Nanomaterials
Ferrocene (Fc) is a stable organic transition metal compound that can produce ROS under physiological condition, and thus, is a potential adjuvant to enhance the therapeutic effect of chemotherapy drugs (Patra and Gasser, 2017). As known to all, the iron in Fc is Fe2+, (Xu et al., 2013) making it an ideal exogenous Fe2+ to induce ferroptosis. For example, sequential release of the NPs based on the presence of heparinase was reported; these NPs were modified with β-cyclodextrin and coloaded with DOX, Fc, and TGF-β receptor inhibitor SB431542 (Figure 9) (Zhang et al., 2021). DOX and Fc could increase the intracellular ROS levels to activate ferroptosis and apoptosis and reduce MMP-9 expression to enhance FBT in the tumor. SB431542 could be gradually released due to the heparanase-driven nature, which prevented the tumor metastasis via modulating the TME, decreasing tumor-associated fibroblast activation, and reducing TGF-β secretion. Furthermore, ferroptosis-induced antitumor immune response enhanced the tumor therapy. A CDT nanomaterial, RSL3@COF–Fc, was fabricated by the covalent organic framework (COF), Fc, and RSL3 (Zhou et al., 2021). The RSL3@COF–Fc could promote the production of •OH in cancer cells, and the repair mechanism under oxidative stress was attenuated by the irreversible inhibition of the GPX4. Finally, these two ways synergistically resulted in massive LPO accumulation.
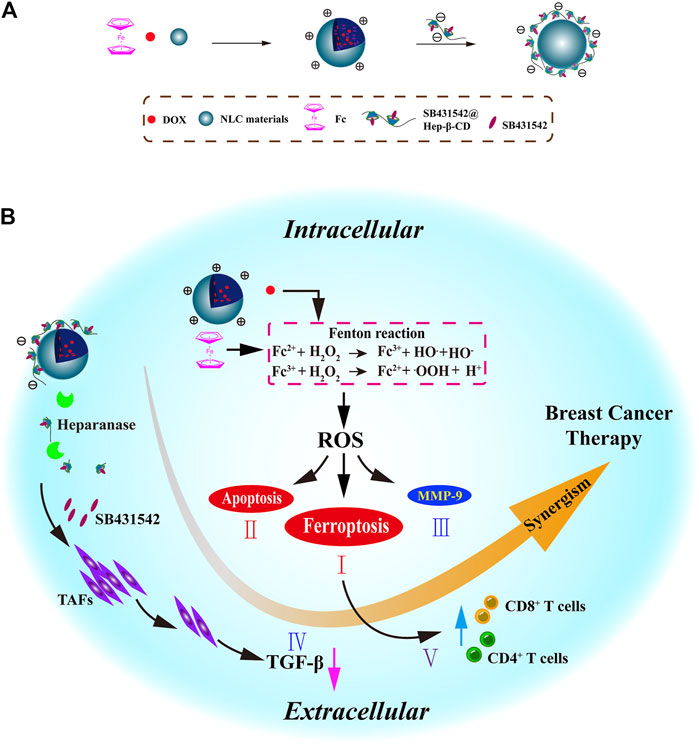
FIGURE 9. (A) Synthesis scheme of NLC/H (DOX + Fc + SB431542) NPs. (B) Mechanism of NLC/H (DOX + Fc + SB431542) NPs for breast cancer therapy. Adapted from Zhang et al., (2021) Copyright @ 2021 (Elsevier).
A multifunctional carbon monoxide (CO)/thermo/chemotherapy nanoplatform comprises the mesoporous carbon NPs (MCNPs) as NIR-responsive drug carriers and DOX and hydrophobic iron carbonyl (FeCO) as thermosensitive CO prodrug (Yao et al., 2019). The nanoplatform produced sufficient heat upon NIR illumination to trigger the release of CO and DOX in the acidic TME. The CO successfully increased the sensitivity of the cancer cells to chemotherapeutics through the ferroptosis pathway. Subsequently, the FeCO-DOX@MCNP nanoplatform showed high therapeutic efficacy in combination with chemotherapy, PTT, and gas therapy both in vitro and in vivo.
3.1.6 Ferritin-Based Nanomaterials
Ferritin is an iron-sequestering protein that plays key roles in iron delivery, cell proliferation, and immunosuppression (Shi et al., 2021). The novel protein-based nanomaterial BCFe@sorafenib was developed by covalently crosslinking the Ce6-conjugated bovine serum albumin (BSA) and ferritin, together with the sorafenib encapsulated inside the protein shell (Wang et al., 2021). Under hypoxic condition, the BCFe@sorafenib could be degraded to release more Ce6 after light irradiation, whereas the ferritin released Fe3+ to consume GSH to produce more ROS. In addition, the released sorafenib destroyed the tumor’s antioxidative defense to enhance oxidative damage. In addition, a novel carrier-free nanodrug NFER containing ferritin, erastin, and rapamycin was prepared by using an emulsification technique (Li et al., 2019). The NFER displayed strong ferroptosis capability by downregulating the GPX4 and increasing LPO production, which was increased by the presence of rapamycin. Using this nanodrug, tumor recurrence of the 4T1 tumor resection model was controlled.
3.1.7 Iron‐Based Up‐Conversion (UC) Nanomaterials
The photic UC is achieved by the UCNPs that contain lanthanide or actinide metals. The Fe3+-containing UCNP and DOX were capsulated into the oxidized starch-based gel NPs (Figure 10) (Bao et al., 2019). As the core, the UCNPs that convert NIR light to ultraviolet (UV) light overcome the obstacle of limited penetration depth and reduced Fe3+ to Fe2+, which led to the deconstruction of gel networks of this nanomaterial and subsequently rapid release of Fe2+ and DOX. As a result, this nanomaterial could provide a safe and efficient platform for ferroptosis-/apoptosis-based anticancer therapy.
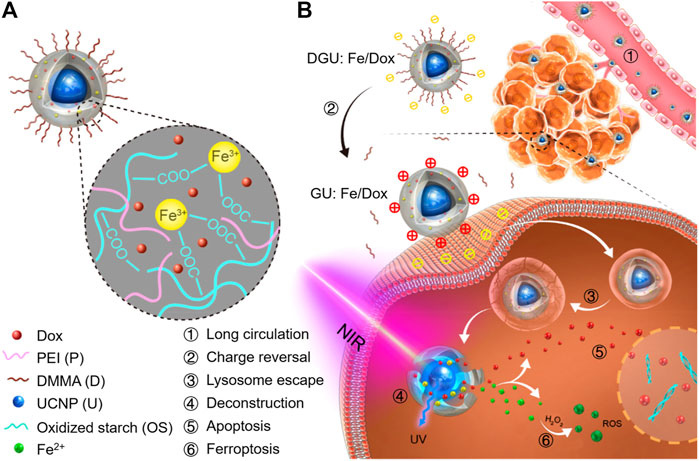
FIGURE 10. (A) Schematic illustration of DGU:Fe/DOX NPs. (B) Mechanism of DGU:Fe/DOX NPs for cancer therapy. Adapted from Bao et al., (2019) Copyright @ 2019 (ACS).
3.2 Iron-Free Nanomaterials
In addition to the iron-contained nanomaterials, there are several types of nanomaterials without iron that could induce ferroptosis of the cancer cells.
3.2.1 Manganese-Based Nanomaterials
A versatile porphyrin-based manganese MOF (Mn-MOF) nanoplatform exhibited high catalase activity for the conversion of H2O2 to O2, thus decreasing the intracellular GSH content and GPX4 activity and inhibiting the tumor growth and metastasis via inducing SDT and FBT (Figure 11) (Xu, Q. et al., 2021). In addition, the Mn-MOF efficiently reshaped the tumor immune microenvironment upon US treatment by increasing the activated CD8+ T cells and matured dendritic cells (DCs) and decreasing the myeloid-derived suppressor cells in tumor tissue. The arginine-rich manganese silicate nanobubbles (AMSNs) could be synthesized through a one-pot method with highly efficient GSH depletion (Wang et al., 2018). The arginine component provides ideal dispersibility, biocompatibility, and tumor-targeting capacity. Compared with the traditional NPs, the arginine-based ultrathin surface coating and nanobubble structure significantly improved the ability of the AMSNs to consume GSH, which led to GPX4 inactivation. In addition, the degradation of AMSNs led to the corelease of Mn2+ and DOX, which resulted in T1-weighted MRI and on-demand chemotherapeutic drug release for the synergistic cancer therapy. In addition, manganese-doped MSNPs (MMSNPs) possess the manganese–oxygen bonds that could consume GSH rapidly (Tang et al., 2019). MMSNPs were modified with FA-PFG and loaded with dihydroartemisinin (DHA) to form an innovative nanomaterial (Fei et al., 2020b). After endocytosis, the degradation of nanomaterials consumed intracellular GSH due to the reaction between the manganese–oxygen bonds and GSH, which resulted in the release of DHA and Mn2+. The sorafenib could also be loaded into MMSNPs to induce ferroptosis of tumor cells (Tang et al., 2019; Tang et al., 2020).
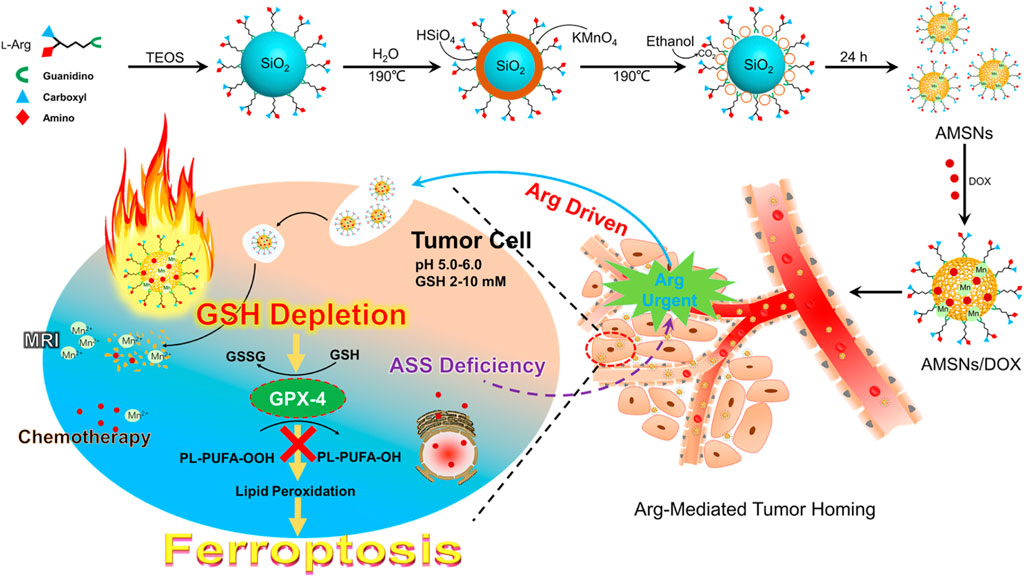
FIGURE 11. Schematic illustration of AMSNs/DOX for cancer therapy. Adapted from Wang et al., (2018) Copyright @ 2018 (ACS).
3.2.2 Manganese Oxide–Based Nanomaterials
The MnO2@HMCu2−xS nanocomposites (HMCMs) for tumor ablation were reported (An et al., 2019). The HMCMs had the ability of depletion of GSH enhanced by PTT, thus inducing PTT-enhanced ferroptosis of cells by GPX4 inactivation. The GSH-responsive Mn2+ release increased the ROS concentration by a Fenton-like reaction, thus resulting in the accumulation of LPO. The rapamycin was encapsulated into the HMCM to improve sensitization of the tumor cells to ferroptosis. The HMCM showed an outstanding anticancer effect in vitro and in vivo. In addition, MnOx nanospikes and ICD drugs were developed as cancer nanovaccines (Figure 12) (Ding et al., 2020). Because of the double induction of CDT mediated by Mn2+ and FBT that depleted GSH, MnOx nanospikes could be used as an immune adjuvant for efficient antigen loading and ICD drugs. The nanovaccines achieved the TME-responsive, dual-mode MRI/PAI, while effectively inhibiting tumor growth and metastasis.
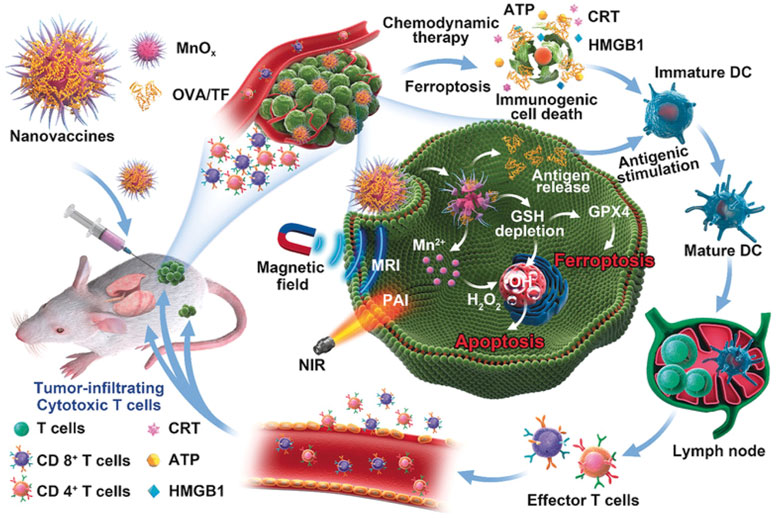
FIGURE 12. Schematic illustration of MnOx-OVA/tumor cell fragment (TF) nanovaccines for MRI-/PAI-induced cancer immunotherapy. Adapted from Ding et al., (2020) Copyright @ 2020 (ACS).
3.2.3 Gold-Based Nanomaterials
The salinomycin that conjugated with the Au NPs was used to kill breast cancer stem cells that were derived from CD24low/CD44high subpopulation (Zhao et al., 2019). The salinomycin-AuNPs exhibited higher ability to induce ferroptosis via higher iron accumulation and GPX4 inactivation. Pt-decorated Au nanostars were presented as novel nanoprodrugs for FBT against MDR tumors (Del Valle et al., 2020). Upon NIR light irradiation, Pt and Au were released, which resulted in GSH depletion, GPX4 inactivation, and accumulation of lipid hydroperoxides. The NIR light-activation of the prodrugs showed efficacy of FBT against tumors without long-term side effects in vivo.
3.2.4 Photosensitizer-Based Nanomaterials
The Ce6 and erastin could be self-assembled to form a novel nanodrug via hydrogen bonding and π–π interactions (Zhu et al., 2019). The erastin could induce accumulation of LPO via the Fenton reaction. Under the light irradiation, the generation of O2 ensured efficient photochemical reactions to produce ROS. The imidazole ligand containing disulfide and Zn2+ was coordinated to form an MOF nanocarrier for Ce6 loading. Regardless of the light irradiation, nanocarriers loaded with Ce6 induced GSH depletion through the disulfide−thiol exchange reaction in 4T1 tumor cells (Meng et al., 2019). In the 4T1 tumor–bearing mouse model, the nanomaterials showed excellent in vivo tumor growth inhibition, and the animal survival rate was improved. However, the combined use of iron chelating agents weakened the antitumor ability of the nanocarrier because it restrained ferroptosis.
The nanoactivator was assembled by DOX, TA, and IR820. The endogenous iron stored in the endo-lysosome was fully utilized. In addition, the ferroptosis and its related oxidative stress were induced by an artificial intracellular positive feedback loop (Figure 13) (Xiong et al., 2021). Interestingly, this process could also promote the ICD-related immunotherapy through the endoplasmic reticulum stress. After the laser treatment, the intracellular ROS in cells could be effectively distributed in the lysosomes and endoplasmic reticulum to promote FBT and immunotherapy, respectively.
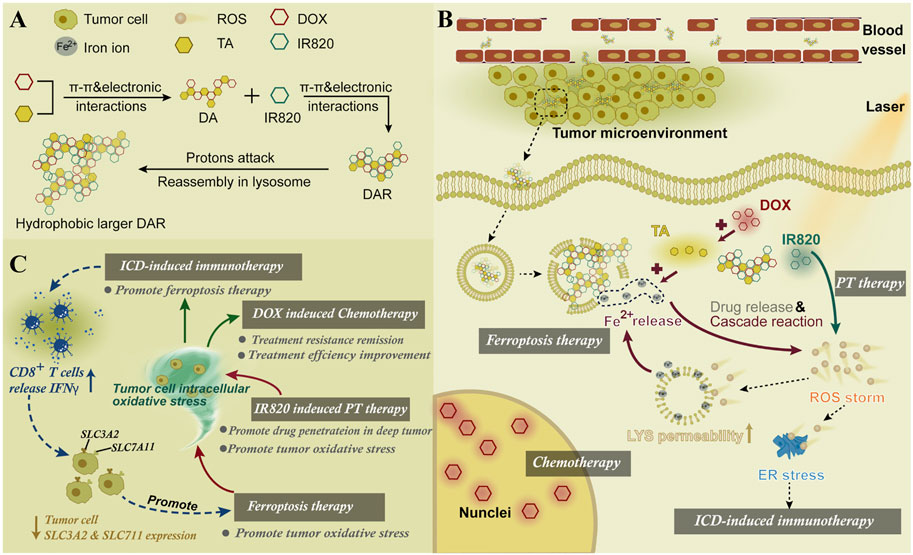
FIGURE 13. (A) Synthesis scheme of DAR. (B) Mechanism of DAR for cancer therapy. (C) Combined therapy mechanisms of DAR. Adapted from Xiong et al., (2021) Copyright @ 2021 (Elsevier).
3.2.5 RSL3-Loaded Nanomaterials
RSL3 can be loaded onto the non-iron nanomaterials. The ionizable block copolymer and phenylboronate ester (PBE) were integrated by covalent bonds to prepare a platform for RSL-3 delivery (Figure 14) (Song et al., 2021). At neutral pH, the NPs could stably embed RSL-3 in the hydrophobic core through π–π interaction with the PBE groups and release the payload in the endogenous vesicles through acidity-triggered cleavage of dynamic covalent bonds of PBE. In addition, the NPs could perform acid-activatable PDT by ionizing nuclear protonation and significantly recruiting tumor infiltrating T lymphocytes to secrete IFN-γ, and thus make the B16–F10 melanoma tumor sensitive to RSL-3–induced ferroptosis. The maltose ligand and azobenzene were linked by PEG and self-assembled to load RSL3, yielding Malt-PEG-Abz@RSL3 (Li et al., 2022). Malt-PEG-Abz@RSL3 micelles could actively target HepG2 because of the high expression of glucose transporters, thus achieving RSL3 delivery. Malt-PEG degraded to release RSL3 for inhibiting GPX4 activity, whereas NADPH that took part in synthesis of GSH and thioredoxin-SH2 [Trx (SH) 2] was consumed by the azobenzene moiety, resulting in the reduction of GSH and Trx (SH) 2. The azobenzene was also connected to a nitroimidazole-conjugated polypeptide and self-assembled with RSL3 (Guo, X. et al., 2020). Under anoxic conditions, the azobenzene moiety caused PEG to be removed and reinforced micelle uptake in 4T1 cells, while the nitroimidazole moiety not only resulted in the rapid release of RSL3 but also exhausted intracellular NADPH with the help of overexpression of nitroreductase in the tumor site.
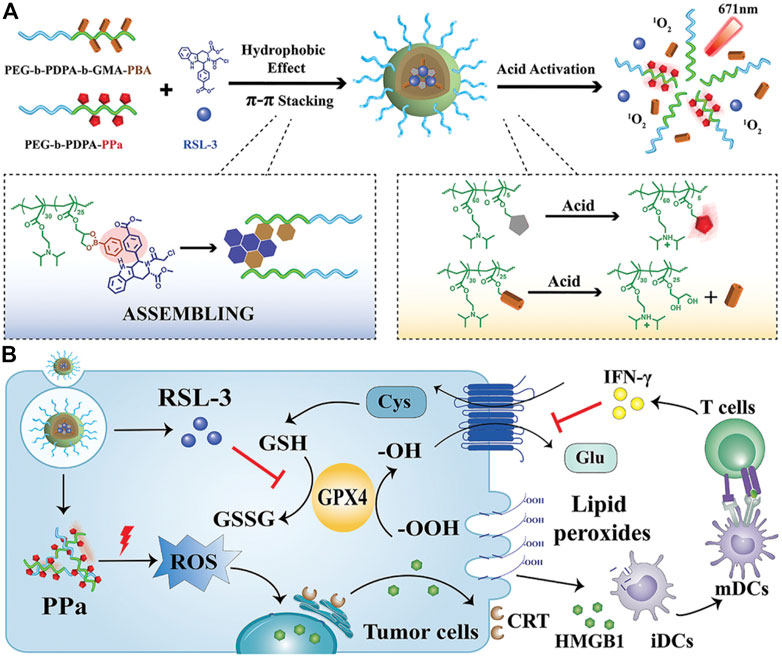
FIGURE 14. (A) Synthesis scheme of NPs. (B) Schematic illustration of the mechanism. Adapted from Song et al., (2021) Copyright @ 2021 (Wiley).
3.2.6 UC Nanomaterials
The core-shell UCNPs (NaGdF4:20%Yb, 0.5%Tm3+@NaGdF4) could absorb NIR light and emit UV to transfer azobenzene combretastatin A4 (azobenzene-CA4) to activate azobenzene-CA4, while UV light could induce reduction of intracellular Fe3+ to Fe2+ (Figure 15) (Zhu et al., 2020). Upon NIR irradiation, the viability of the triple-negative breast cancer cells treated with nanocarriers loaded with azobenzene-CA4 evidently decreased. Another UCNP (NaYF4:20%Yb, 2%Er@NaYF4) was coated by the MS and liposome to encapsulate Ce6 and buthionine sulfoximine (BSO) (Li D et al., 2021; Li Z et al., 2021). Notably, Ce6-induced ROS production, BSO-caused GSH depletion, and inactivation of GPX4 could amplify apoptosis and ferroptosis. Efficient tumor destruction led to increased exposure of high mobility group box 1 (HMGB1) and calreticulin enhancing antitumor immune responses, including maturation of DCs and effector function of tumor-infiltrating T lymphocytes, which resulted in the elimination of the residual melanoma cells.
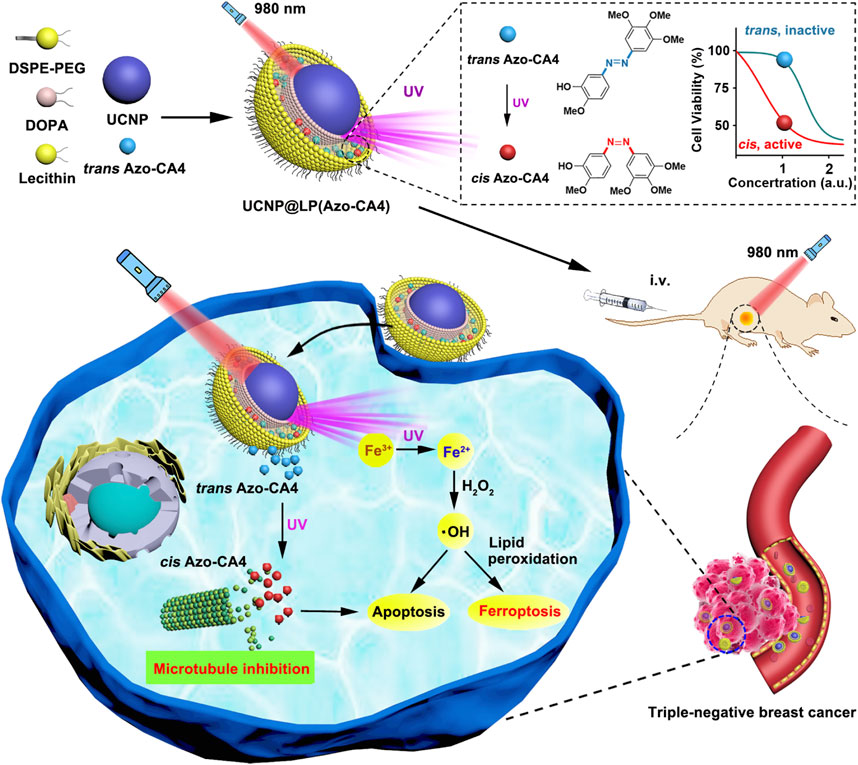
FIGURE 15. Synthesis scheme and mechanism of UCNP@LP (Azo-CA4). Adapted from Zhu et al., (2020) Copyright @ 2020 (ACS).
4 Conclusions and Future Perspectives
With the development of life sciences and medicine, the genetic and molecular mechanisms of cancer have been extensively investigated by researchers worldwide. Many countries have invested considerable material and financial resources in this endeavor. However, tumorigenesis, progression, metastasis, recurrence, and death caused by cancer are still poorly understood because of the tumor complexity including diverse molecular types and heterogeneity; thus, the therapeutic outcomes remain unsatisfactory. Therefore, finding novel therapeutic strategies to solve the human cancer issue has become the most significant problem that needs to be urgently addressed to protect human health. Ferroptosis, termed in 2012, has been widely researched concerning the cellular mechanisms and signaling pathways in cancers. As a novel type of cell death, ferroptosis has great potential as a new cancer therapy, and several ferroptosis-inducing small molecules (such as erastin, sorafenib, sulfasalazine, altretamine, and artemisinin) and various genes related to ferroptosis (e.g., xCT, GPX4, p53, NQO1, and NOX) have been discovered by the researchers. Nonetheless, there are also some disadvantages: 1) there are potential adverse effects of ferroptosis on normal tissues due to the low specificity of small-molecule compounds; 2) small-molecule compounds have short half-lives in blood and low accumulation at tumor sites because of rapid renal clearance; 3) gene-based FBT for cancer is essentially one type of gene therapy, which might bring controversy at ethics and law (Shen et al., 2018). Hence, although FBT based on small molecules and genes is promising, significant research is required before it can be applied clinically.
With the rapid development of nanomedicine, numerous ferroptosis-inducing nanomaterials have been developed by chemists, pharmacists, and material scientists. The adverse effects on normal tissues could be decreased because of the precise targetability of the nanomaterials to tumor sites, and the nanomaterials possess long circulating half-lives in the blood, which enhances the accumulation of the nanomaterials at tumor sites. The ferroptosis-based nanomaterials could be triggered by several factors (such as light, US, and TME) to induce the release of metal irons, metal oxides, or drugs to induce or enhance ferroptosis. The FBT of cancer could integrate with different models of imaging guidance (such as US imaging, PAI, and MRI) and combine with diverse cancer therapies (such as CDT, SDT, PDT, PTT, and immunotherapy). In this review, we roughly divide the reported ferroptosis-based nanomaterials into two types: iron-contained nanomaterials and iron-free nanomaterials. The former induces ferroptosis via increasing the external iron to enhance the Fenton reaction; meanwhile, the latter induces ferroptosis via GSH depletion, GPX4 inactivation, or increasing intracellular ROS, which leads to the prospective that it is difficult for the iron-free nanomaterials to achieve satisfactory treatment. Therefore, iron-contained nanomaterials might be more promising than iron-free nanomaterials because the latter is limited by utilizing the intracellular restricted iron to produce ROS via the Fenton reaction.
Undoubtedly, in the future, a mass of ferroptosis-based nanomaterials will be investigated for cancer therapy. The future research might focus on the following aspects: 1) more ferroptosis small-molecular compounds will be discovered and synthesized, and subsequently they might be utilized to be encapsulated into the iron-contained nanomaterials for FBT; 2) the siRNAs targeting ferroptosis-related genes (e.g., p53, GPX4, and xCT) might be used to enhance the effect of FBT; 3) the ferroptosis-based nanomaterials might combine with radiotherapy to make tumor sensitized to radiation; 4) more nanomedical research about the combination treatment of FBT and immunotherapy needs to be brought to the forefront because of its great clinical application prospects. Meanwhile, multiple issues should be taken into consideration in the rational design of the nanomaterials based on ferroptosis in the future, such as safety and feasibility of clinical application, possible toxicity, and industrial feasibility.
Author Contributions
ZS, JZ, WT, and CS designed and conceptualized the review. All authors contributed to the writing of the manuscript.
Funding
This work was supported by the National Natural Science Foundation of China (No. 81972373), the Natural Science Foundation of Fujian Province of China (No.2019J01016), the funding of the Science and Technology Planned Project of Medical and Health of Xiamen City (No. 3502Z20194038), The Scientific Research Foundation for Advanced Talents of Xiang’an Hospital of Xiamen University (No. PM201809170001), and the Medical Leading Talents of Xiamen City.
Conflict of Interest
The authors declare that the research was conducted in the absence of any commercial or financial relationships that could be construed as a potential conflict of interest.
Publisher’s Note
All claims expressed in this article are solely those of the authors and do not necessarily represent those of their affiliated organizations, or those of the publisher, the editors, and the reviewers. Any product that may be evaluated in this article, or claim that may be made by its manufacturer, is not guaranteed or endorsed by the publisher.
Acknowledgments
ZS would like to thank his wife Meiying Huang and his parents who have given him strong spiritual and financial support during his hard times. Thanks to editor and typesetter for their kind help.
References
An, P., Gao, Z., Sun, K., Gu, D., Wu, H., You, C., et al. (2019). Photothermal-Enhanced Inactivation of Glutathione Peroxidase for Ferroptosis Sensitized by an Autophagy Promotor. ACS Appl. Mater. Inter. 11 (46), 42988–42997. doi:10.1021/acsami.9b16124
Asghari, F., Khademi, R., Esmaeili Ranjbar, F., Veisi Malekshahi, Z., and Faridi Majidi, R. (2019). Application of Nanotechnology in Targeting of Cancer Stem Cells: A Review. Ijsc 12 (2), 227–239. doi:10.15283/ijsc19006
Bao, W., Liu, X., Lv, Y., Lu, G.-H., Li, F., Zhang, F., et al. (2019). Nanolongan with Multiple On-Demand Conversions for Ferroptosis-Apoptosis Combined Anticancer Therapy. ACS Nano 13 (1), 260–273. doi:10.1021/acsnano.8b05602
Bray, F., Laversanne, M., Weiderpass, E., and Soerjomataram, I. (2021). The Ever‐increasing Importance of Cancer as a Leading Cause of Premature Death Worldwide. Cancer 127 (16), 3029–3030. doi:10.1002/cncr.33587
Cao, J. Y., and Dixon, S. J. (2016). Mechanisms of Ferroptosis. Cell. Mol. Life Sci. 73 (11-12), 2195–2209. doi:10.1007/s00018-016-2194-1
Chen, G., Yang, Y., Xu, Q., Ling, M., Lin, H., Ma, W., et al. (2020). Self-Amplification of Tumor Oxidative Stress with Degradable Metallic Complexes for Synergistic Cascade Tumor Therapy. Nano Lett. 20 (11), 8141–8150. doi:10.1021/acs.nanolett.0c03127
Chen, H., Yan, Z., Wu, S., and Li, F. (2021). A Glutathione-Responsive Polyphenol - Constructed Nanodevice for Double Roles in Apoptosis and Ferroptosis. Colloids Surf. B: Biointerfaces 205, 111902. doi:10.1016/j.colsurfb.2021.111902
Chen, L., Lin, Z., Liu, L., Zhang, X., Shi, W., Ge, D., et al. (2019). Fe2+/Fe3+ Ions Chelated with Ultrasmall Polydopamine Nanoparticles Induce Ferroptosis for Cancer Therapy. ACS Biomater. Sci. Eng. 5 (9), 4861–4869. doi:10.1021/acsbiomaterials.9b00461
Chen, Q., Ma, X., Xie, L., Chen, W., Xu, Z., Song, E., et al. (2021). Iron-based Nanoparticles for MR Imaging-Guided Ferroptosis in Combination with Photodynamic Therapy to Enhance Cancer Treatment. Nanoscale 13 (9), 4855–4870. doi:10.1039/d0nr08757b
Cheng, H., Wang, X., Liu, X., Wang, X., Wen, H., Cheng, Y., et al. (2021). An Effective NIR Laser/tumor-Microenvironment Co-responsive Cancer Theranostic Nanoplatform with Multi-Modal Imaging and Therapies. Nanoscale 13 (24), 10816–10828. doi:10.1039/d1nr01645h
Cheng, J., Zhu, Y., Xing, X., Xiao, J., Chen, H., Zhang, H., et al. (2021). Manganese-deposited Iron Oxide Promotes Tumor-Responsive Ferroptosis that Synergizes the Apoptosis of Cisplatin. Theranostics 11 (11), 5418–5429. doi:10.7150/thno.53346
Cheng, W., Zeng, X., Chen, H., Li, Z., Zeng, W., Mei, L., et al. (2019). Versatile Polydopamine Platforms: Synthesis and Promising Applications for Surface Modification and Advanced Nanomedicine. ACS Nano 13 (8), 8537–8565. doi:10.1021/acsnano.9b04436
Cui, X., Lu, G., Fang, F., Xiong, Y., Tian, S., Wan, Y., et al. (2021). Iron Self-Boosting Polymer Nanoenzyme for Low-Temperature Photothermal-Enhanced Ferrotherapy. ACS Appl. Mater. Inter. 13 (26), 30274–30283. doi:10.1021/acsami.1c01658
Debela, D. T., Muzazu, S. G., Heraro, K. D., Ndalama, M. T., Mesele, B. W., Haile, D. C., et al. (2021). New Approaches and Procedures for Cancer Treatment: Current Perspectives. SAGE Open Med. 9, 205031212110343. doi:10.1177/20503121211034366
Ding, B., Zheng, P., Jiang, F., Zhao, Y., Wang, M., Chang, M., et al. (2020). MnO X Nanospikes as Nanoadjuvants and Immunogenic Cell Death Drugs with Enhanced Antitumor Immunity and Antimetastatic Effect. Angew. Chem. Int. Ed. 59 (38), 16381–16384. doi:10.1002/anie.202005111
Dixon, S. J., Lemberg, K. M., Lamprecht, M. R., Skouta, R., Zaitsev, E. M., Gleason, C. E., et al. (2012). Ferroptosis: an Iron-dependent Form of Nonapoptotic Cell Death. Cell 149 (5), 1060–1072. doi:10.1016/j.cell.2012.03.042
Dolma, S., Lessnick, S. L., Hahn, W. C., and Stockwell, B. R. (2003). Identification of Genotype-Selective Antitumor Agents Using Synthetic Lethal Chemical Screening in Engineered Human Tumor Cells. Cancer Cell 3 (3), 285–296. doi:10.1016/s1535-6108(03)00050-3
Fang, J., Nakamura, H., and Maeda, H. (2011). The EPR Effect: Unique Features of Tumor Blood Vessels for Drug Delivery, Factors Involved, and Limitations and Augmentation of the Effect. Adv. Drug Deliv. Rev. 63 (3), 136–151. doi:10.1016/j.addr.2010.04.009
Fei, W., Chen, D., Tang, H., Li, C., Zheng, W., Chen, F., et al. (2020b). Targeted GSH-Exhausting and Hydroxyl Radical Self-Producing Manganese-Silica Nanomissiles for MRI Guided Ferroptotic Cancer Therapy. Nanoscale 12 (32), 16738–16754. doi:10.1039/d0nr02396e
Fei, W., Zhang, Y., Ye, Y., Li, C., Yao, Y., Zhang, M., et al. (2020a). Bioactive Metal-Containing Nanomaterials for Ferroptotic Cancer Therapy. J. Mater. Chem. B 8 (46), 10461–10473. doi:10.1039/d0tb02138e
Feng, H., and Stockwell, B. R. (2018). Unsolved Mysteries: How Does Lipid Peroxidation Cause Ferroptosis? Plos Biol. 16 (5), e2006203. doi:10.1371/journal.pbio.2006203
Fu, J., Li, T., Yang, Y., Jiang, L., Wang, W., Fu, L., et al. (2021). Activatable Nanomedicine for Overcoming Hypoxia-Induced Resistance to Chemotherapy and Inhibiting Tumor Growth by Inducing Collaborative Apoptosis and Ferroptosis in Solid Tumors. Biomaterials 268, 120537. doi:10.1016/j.biomaterials.2020.120537
Gao, Z., He, T., Zhang, P., Li, X., Zhang, Y., Lin, J., et al. (2020). Polypeptide-Based Theranostics with Tumor-Microenvironment-Activatable Cascade Reaction for Chemo-Ferroptosis Combination Therapy. ACS Appl. Mater. Inter. 12 (18), 20271–20280. doi:10.1021/acsami.0c03748
Gaschler, M. M., Andia, A. A., Liu, H., Csuka, J. M., Hurlocker, B., Vaiana, C. A., et al. (2018). FINO2 Initiates Ferroptosis through GPX4 Inactivation and Iron Oxidation. Nat. Chem. Biol. 14 (5), 507–515. doi:10.1038/s41589-018-0031-6
Golombek, S. K., May, J.-N., Theek, B., Appold, L., Drude, N., Kiessling, F., et al. (2018). Tumor Targeting via EPR: Strategies to Enhance Patient Responses. Adv. Drug Deliv. Rev. 130, 17–38. doi:10.1016/j.addr.2018.07.007
Gu, Z., Liu, T., Liu, C., Yang, Y., Tang, J., Song, H., et al. (2021). Ferroptosis-Strengthened Metabolic and Inflammatory Regulation of Tumor-Associated Macrophages Provokes Potent Tumoricidal Activities. Nano Lett. 21 (15), 6471–6479. doi:10.1021/acs.nanolett.1c01401
Guan, Q., Guo, R., Huang, S., Zhang, F., Liu, J., Wang, Z., et al. (2020). Mesoporous Polydopamine Carrying Sorafenib and SPIO Nanoparticles for MRI-Guided Ferroptosis Cancer Therapy. J. Controlled Release 320, 392–403. doi:10.1016/j.jconrel.2020.01.048
Guo, P., Wang, L., Shang, W., Chen, J., Chen, Z., Xiong, F., et al. (2020). Intravesical In Situ Immunostimulatory Gel for Triple Therapy of Bladder Cancer. ACS Appl. Mater. Inter. 12 (49), 54367–54377. doi:10.1021/acsami.0c15176
Guo, X., Liu, F., Deng, J., Dai, P., Qin, Y., Li, Z., et al. (2020). Electron-Accepting Micelles Deplete Reduced Nicotinamide Adenine Dinucleotide Phosphate and Impair Two Antioxidant Cascades for Ferroptosis-Induced Tumor Eradication. ACS Nano 14 (11), 14715–14730. doi:10.1021/acsnano.0c00764
Guo, Y., Zhang, X., Sun, W., Jia, H.-R., Zhu, Y.-X., Zhang, X., et al. (2019). Metal-Phenolic Network-Based Nanocomplexes that Evoke Ferroptosis by Apoptosis: Promoted Nuclear Drug Influx and Reversed Drug Resistance of Cancer. Chem. Mater. 31 (24), 10071–10084. doi:10.1021/acs.chemmater.9b03042
Hanahan, D. (2022). Hallmarks of Cancer: New Dimensions. Cancer Discov. 12 (1), 31–46. doi:10.1158/2159-8290.CD-21-1059
He, H., Du, L., Guo, H., An, Y., Lu, L., Chen, Y., et al. (2020). Redox Responsive Metal Organic Framework Nanoparticles Induces Ferroptosis for Cancer Therapy. Small 16 (33), 2001251. doi:10.1002/smll.202001251
He, S., Jiang, Y., Li, J., and Pu, K. (2020). Semiconducting Polycomplex Nanoparticles for Photothermal Ferrotherapy of Cancer. Angew. Chem. Int. Ed. 59 (26), 10633–10638. doi:10.1002/anie.202003004
Hu, Z., Wang, S., Dai, Z., Zhang, H., and Zheng, X. (2020). A Novel Theranostic Nano-Platform (PB@FePt-HA-g-PEG) for Tumor Chemodynamic-Photothermal Co-therapy and Triple-Modal Imaging (MR/CT/PI) Diagnosis. J. Mater. Chem. B 8 (24), 5351–5360. doi:10.1039/d0tb00708k
Huang, K.-J., Wei, Y.-H., Chiu, Y.-C., Wu, S.-R., and Shieh, D.-B. (2019). Assessment of Zero-Valent Iron-Based Nanotherapeutics for Ferroptosis Induction and Resensitization Strategy in Cancer Cells. Biomater. Sci. 7 (4), 1311–1322. doi:10.1039/c8bm01525b
JiangQ, ., Wang, K., Zhang, X., Ouyang, B., Liu, H., Pang, Z., et al. (2020). Platelet Membrane‐Camouflaged Magnetic Nanoparticles for Ferroptosis‐Enhanced Cancer Immunotherapy. Small 16 (22), 2001704. doi:10.1002/smll.202001704
JiangY, ., Zhao, X., Huang, J., Li, J., Upputuri, P. K., Sun, H., et al. (2020). Transformable Hybrid Semiconducting Polymer Nanozyme for Second Near-Infrared Photothermal Ferrotherapy. Nat. Commun. 11 (1), 1857. doi:10.1038/s41467-020-15730-x
Kim, S. E., Zhang, L., Ma, K., Riegman, M., Chen, F., Ingold, I., et al. (2016). Ultrasmall Nanoparticles Induce Ferroptosis in Nutrient-Deprived Cancer Cells and Suppress Tumour Growth. Nat. Nanotech 11 (11), 977–985. doi:10.1038/nnano.2016.164
Li, D., Ren, J., Li, J., Zhang, Y., Lou, Y., Zhu, J., et al. (2021). Ferroptosis-apoptosis Combined Anti-melanoma Immunotherapy with a NIR-Responsive Upconverting mSiO2 Photodynamic Platform. Chem. Eng. J. 419, 129557. doi:10.1016/j.cej.2021.129557
Li, J., Cao, F., Yin, H.-l., Huang, Z.-j., Lin, Z.-t., Mao, N., et al. (2020). Ferroptosis: Past, Present and Future. Cell Death Dis 11 (2), 88. doi:10.1038/s41419-020-2298-2
Li, W., Liu, X., Cheng, X., Zhang, W., Gong, C., Gao, C., et al. (2022). Effect of Malt-PEG-Abz@RSL3 Micelles on HepG2 Cells Based on NADPH Depletion and GPX4 Inhibition in Ferroptosis. J. Drug Target. 30 (2), 208–218. doi:10.1080/1061186X.2021.1953511
Li, Y., Chen, W., Qi, Y., Wang, S., Li, L., Li, W., et al. (2020). H 2 S‐Scavenged and Activated Iron Oxide‐Hydroxide Nanospindles for MRI‐Guided Photothermal Therapy and Ferroptosis in Colon Cancer. Small 16 (37), 2001356. doi:10.1002/smll.202001356
Li, Y., Wang, X., Yan, J., Liu, Y., Yang, R., Pan, D., et al. (2019). Nanoparticle Ferritin-Bound Erastin and Rapamycin: a Nanodrug Combining Autophagy and Ferroptosis for Anticancer Therapy. Biomater. Sci. 7 (9), 3779–3787. doi:10.1039/c9bm00653b
Li, Z., Wu, X., Wang, W., Gai, C., Zhang, W., Li, W., et al. (2021). Fe(II) and Tannic Acid-Cloaked MOF as Carrier of Artemisinin for Supply of Ferrous Ions to Enhance Treatment of Triple-Negative Breast Cancer. Nanoscale Res. Lett. 16 (1), 37. doi:10.1186/s11671-021-03497-z
Liang, C., Zhang, X., Yang, M., and Dong, X. (2019). Recent Progress in Ferroptosis Inducers for Cancer Therapy. Adv. Mater. 31 (51), 1904197. doi:10.1002/adma.201904197
Liu, T., Liu, W., Zhang, M., Yu, W., Gao, F., Li, C., et al. (2018). Ferrous-Supply-Regeneration Nanoengineering for Cancer-cell-specific Ferroptosis in Combination with Imaging-Guided Photodynamic Therapy. ACS Nano 12 (12), 12181–12192. doi:10.1021/acsnano.8b05860
Liu, X., Zhu, X., Qi, X., Meng, X., and Xu, K. (2021). Co-Administration of iRGD with Sorafenib-Loaded Iron-Based Metal-Organic Framework as a Targeted Ferroptosis Agent for Liver Cancer Therapy. Ijn Vol. 16, 1037–1050. doi:10.2147/IJN.S292528
Luo, C., Sun, J., Sun, B., and He, Z. (2014). Prodrug-based Nanoparticulate Drug Delivery Strategies for Cancer Therapy. Trends Pharmacol. Sci. 35 (11), 556–566. doi:10.1016/j.tips.2014.09.008
Maeda, H. (2015). Toward a Full Understanding of the EPR Effect in Primary and Metastatic Tumors as Well as Issues Related to its Heterogeneity. Adv. Drug Deliv. Rev. 91, 3–6. doi:10.1016/j.addr.2015.01.002
Meng, X., Deng, J., Liu, F., Guo, T., Liu, M., Dai, P., et al. (2019). Triggered All-Active Metal Organic Framework: Ferroptosis Machinery Contributes to the Apoptotic Photodynamic Antitumor Therapy. Nano Lett. 19 (11), 7866–7876. doi:10.1021/acs.nanolett.9b02904
Meng, Y., Zhang, D., Sun, Y., Dai, Z., Zhang, T., Yu, D., et al. (2020). Core-shell FePt-Cube@covalent Organic Polymer Nanocomposites: a Multifunctional Nanocatalytic Agent for Primary and Metastatic Tumor Treatment. J. Mater. Chem. B 8 (48), 11021–11032. doi:10.1039/d0tb01981j
Nieto, C., Vega, M. A., and Martín del Valle, E. M. (2021). Tailored-Made Polydopamine Nanoparticles to Induce Ferroptosis in Breast Cancer Cells in Combination with Chemotherapy. Ijms 22 (6), 3161. doi:10.3390/ijms22063161
Park, J., Choi, Y., Chang, H., Um, W., Ryu, J. H., and Kwon, I. C. (2019). Alliance with EPR Effect: Combined Strategies to Improve the EPR Effect in the Tumor Microenvironment. Theranostics 9 (26), 8073–8090. doi:10.7150/thno.37198
Patra, M., and Gasser, G. (2017). The Medicinal Chemistry of Ferrocene and its Derivatives. Nat. Rev. Chem. 1 (9), 0066. doi:10.1038/s41570-017-0066
Rahim, M. A., Kristufek, S. L., Pan, S., Richardson, J. J., and Caruso, F. (2019). Phenolic Building Blocks for the Assembly of Functional Materials. Angew. Chem. Int. Ed. 58 (7), 1904–1927. doi:10.1002/anie.201807804
Sang, M., Luo, R., Bai, Y., Dou, J., Zhang, Z., Liu, F., et al. (2019a). BHQ-Cyanine-Based "Off-On" Long-Circulating Assembly as a Ferroptosis Amplifier for Cancer Treatment: A Lipid-Peroxidation Burst Device. ACS Appl. Mater. Inter. 11 (46), 42873–42884. doi:10.1021/acsami.9b12469
Sang, M., Luo, R., Bai, Y., Dou, J., Zhang, Z., Liu, F., et al. (2019b). Mitochondrial Membrane Anchored Photosensitive Nano-Device for Lipid Hydroperoxides Burst and Inducing Ferroptosis to Surmount Therapy-Resistant Cancer. Theranostics 9 (21), 6209–6223. doi:10.7150/thno.36283
Shan, X., Li, S., Sun, B., Chen, Q., Sun, J., He, Z., et al. (2020). Ferroptosis-driven Nanotherapeutics for Cancer Treatment. J. Controlled Release 319, 322–332. doi:10.1016/j.jconrel.2020.01.008
Shen, Z., Song, J., Yung, B. C., Zhou, Z., Wu, A., and Chen, X. (2018). Emerging Strategies of Cancer Therapy Based on Ferroptosis. Adv. Mater. 30 (12), 1704007. doi:10.1002/adma.201704007
Shi, J., Kantoff, P. W., Wooster, R., and Farokhzad, O. C. (2017). Cancer Nanomedicine: Progress, Challenges and Opportunities. Nat. Rev. Cancer 17 (1), 20–37. doi:10.1038/nrc.2016.108
Shi, Y., Liu, S., Liu, Y., Sun, C., Chang, M., Zhao, X., et al. (2019). Facile Fabrication of Nanoscale Porphyrinic Covalent Organic Polymers for Combined Photodynamic and Photothermal Cancer Therapy. ACS Appl. Mater. Inter. 11 (13), 12321–12326. doi:10.1021/acsami.9b00361
Shi, Z., Zhang, L., Zheng, J., Sun, H., and Shao, C. (2021). Ferroptosis: Biochemistry and Biology in Cancers. Front. Oncol. 11, 579286. doi:10.3389/fonc.2021.579286
Shimada, K., Skouta, R., Kaplan, A., Yang, W. S., Hayano, M., Dixon, S. J., et al. (2016). Global Survey of Cell Death Mechanisms Reveals Metabolic Regulation of Ferroptosis. Nat. Chem. Biol. 12 (7), 497–503. doi:10.1038/nchembio.2079
Song, R., Li, T., Ye, J., Sun, F., Hou, B., Saeed, M., et al. (2021). Acidity‐Activatable Dynamic Nanoparticles Boosting Ferroptotic Cell Death for Immunotherapy of Cancer. Adv. Mater. 33 (31), 2101155. doi:10.1002/adma.202101155
Sung, H., Ferlay, J., Siegel, R. L., Laversanne, M., Soerjomataram, I., Jemal, A., et al. (2021). Global Cancer Statistics 2020: GLOBOCAN Estimates of Incidence and Mortality Worldwide for 36 Cancers in 185 Countries. CA A. Cancer J. Clin. 71 (3), 209–249. doi:10.3322/caac.21660
Tang, H., Chen, D., Li, C., Zheng, C., Wu, X., Zhang, Y., et al. (2019). Dual GSH-Exhausting Sorafenib Loaded Manganese-Silica Nanodrugs for Inducing the Ferroptosis of Hepatocellular Carcinoma Cells. Int. J. Pharmaceutics 572, 118782. doi:10.1016/j.ijpharm.2019.118782
Tang, H., Li, C., Zhang, Y., Zheng, H., Cheng, Y., Zhu, J., et al. (2020). Targeted Manganese Doped Silica Nano GSH-Cleaner for Treatment of Liver Cancer by Destroying the Intracellular Redox Homeostasis. Theranostics 10 (21), 9865–9887. doi:10.7150/thno.46771
Valle, A. C., Yeh, C. K., and Huang, Y. F. (2020). Near Infrared‐Activatable Platinum‐Decorated Gold Nanostars for Synergistic Photothermal/Ferroptotic Therapy in Combating Cancer Drug Resistance. Adv. Healthc. Mater. 9 (20), 2000864. doi:10.1002/adhm.202000864
Wan, X., Song, L., Pan, W., Zhong, H., Li, N., and Tang, B. (2020). Tumor-Targeted Cascade Nanoreactor Based on Metal-Organic Frameworks for Synergistic Ferroptosis-Starvation Anticancer Therapy. ACS Nano 14 (9), 11017–11028. doi:10.1021/acsnano.9b07789
Wang, S., Li, F., Qiao, R., Hu, X., Liao, H., Chen, L., et al. (2018). Arginine-Rich Manganese Silicate Nanobubbles as a Ferroptosis-Inducing Agent for Tumor-Targeted Theranostics. ACS Nano 12 (12), 12380–12392. doi:10.1021/acsnano.8b06399
Wang, X., Wu, M., Zhang, X., Li, F., Zeng, Y., Lin, X., et al. (2021). Hypoxia-responsive Nanoreactors Based on Self-Enhanced Photodynamic Sensitization and Triggered Ferroptosis for Cancer Synergistic Therapy. J. Nanobiotechnol 19 (1), 204. doi:10.1186/s12951-021-00952-y
Wolpaw, A. J., Shimada, K., Skouta, R., Welsch, M. E., Akavia, U. D., Pe'er, D., et al. (2011). Modulatory Profiling Identifies Mechanisms of Small Molecule-Induced Cell Death. Proc. Natl. Acad. Sci. 108 (39), E771–E780. doi:10.1073/pnas.1106149108
Xie, S., Sun, W., Zhang, C., Dong, B., Yang, J., Hou, M., et al. (2021). Metabolic Control by Heat Stress Determining Cell Fate to Ferroptosis for Effective Cancer Therapy. ACS Nano 15 (4), 7179–7194. doi:10.1021/acsnano.1c00380
Xin, H., Huang, Y., Tang, H., Chen, Y., Xia, H., Zhang, F., et al. (2021). Delivery of a System Xc− Inhibitor by a Redox-Responsive Levodopa Prodrug Nanoassembly for Combination Ferrotherapy. J. Mater. Chem. B 9 (35), 7172–7181. doi:10.1039/d1tb00742d
Xiong, H., Wang, C., Wang, Z., Lu, H., and Yao, J. (2021). Self-assembled Nano-Activator Constructed Ferroptosis-Immunotherapy through Hijacking Endogenous Iron to Intracellular Positive Feedback Loop. J. Controlled Release 332, 539–552. doi:10.1016/j.jconrel.2021.03.007
Xu, C., Lin, Y., Wang, J., Wu, L., Wei, W., Ren, J., et al. (2013). Nanoceria-Triggered Synergetic Drug Release Based on CeO2-Capped Mesoporous Silica Host-Guest Interactions and Switchable Enzymatic Activity and Cellular Effects of CeO2. Adv. Healthc. Mater. 2 (12), 1591–1599. doi:10.1002/adhm.201200464
Xu, Q., Zhan, G., Zhang, Z., Yong, T., Yang, X., and Gan, L. (2021). Manganese Porphyrin-Based Metal-Organic Framework for Synergistic Sonodynamic Therapy and Ferroptosis in Hypoxic Tumors. Theranostics 11 (4), 1937–1952. doi:10.7150/thno.45511
Xu, R., Yang, J., Qian, Y., Deng, H., Wang, Z., Ma, S., et al. (2021). Ferroptosis/pyroptosis Dual-Inductive Combinational Anti-cancer Therapy Achieved by Transferrin Decorated nanoMOF. Nanoscale Horiz. 6 (4), 348–356. doi:10.1039/d0nh00674b
Xu, X., Chen, Y., Zhang, Y., Yao, Y., and Ji, P. (2020). Highly Stable and Biocompatible Hyaluronic Acid-Rehabilitated Nanoscale MOF-Fe2+induced Ferroptosis in Breast Cancer Cells. J. Mater. Chem. B 8, 9129–9138. doi:10.1039/d0tb01616k
Xue, C.-C., Li, M.-H., Zhao, Y., Zhou, J., Hu, Y., Cai, K.-Y., et al. (2020). Tumor Microenvironment-Activatable Fe-Doxorubicin Preloaded Amorphous CaCO 3 Nanoformulation Triggers Ferroptosis in Target Tumor Cells. Sci. Adv. 6 (18), eaax1346. doi:10.1126/sciadv.aax1346
Xue, C., Li, M., Liu, C., Li, Y., Fei, Y., Hu, Y., et al. (2021). NIR‐Actuated Remote Activation of Ferroptosis in Target Tumor Cells through a Photothermally Responsive Iron‐Chelated Biopolymer Nanoplatform. Angew. Chem. Int. Ed. 60 (16), 8938–8947. doi:10.1002/anie.202016872
Yagoda, N., von Rechenberg, M., Zaganjor, E., Bauer, A. J., Yang, W. S., Fridman, D. J., et al. (2007). RAS-RAF-MEK-dependent Oxidative Cell Death Involving Voltage-dependent Anion Channels. Nature 447 (7146), 865–869. doi:10.1038/nature05859
Yamagishi, K., Kirino, I., Takahashi, I., Amano, H., Takeoka, S., Morimoto, Y., et al. (2019). Tissue-adhesive Wirelessly Powered Optoelectronic Device for Metronomic Photodynamic Cancer Therapy. Nat. Biomed. Eng. 3 (1), 27–36. doi:10.1038/s41551-018-0261-7
Yang, B., Liu, Q., Yao, X., Zhang, D., Dai, Z., Cui, P., et al. (2019). FePt@MnO-Based Nanotheranostic Platform with Acidity-Triggered Dual-Ions Release for Enhanced MR Imaging-Guided Ferroptosis Chemodynamic Therapy. ACS Appl. Mater. Inter. 11 (42), 38395–38404. doi:10.1021/acsami.9b11353
Yang, J., Ma, S., Xu, R., Wei, Y., Zhang, J., Zuo, T., et al. (2021). Smart Biomimetic Metal Organic Frameworks Based on ROS-Ferroptosis-Glycolysis Regulation for Enhanced Tumor Chemo-Immunotherapy. J. Controlled Release 334, 21–33. doi:10.1016/j.jconrel.2021.04.013
Yang, W. S., and Stockwell, B. R. (2008). Synthetic Lethal Screening Identifies Compounds Activating Iron-dependent, Nonapoptotic Cell Death in Oncogenic-RAS-Harboring Cancer Cells. Chem. Biol. 15 (3), 234–245. doi:10.1016/j.chembiol.2008.02.010
Yang, Y., Tian, Q., Wu, S., Li, Y., Yang, K., Yan, Y., et al. (202112073). Blue Light-Triggered Fe2+-Release from Monodispersed Ferrihydrite Nanoparticles for Cancer Iron Therapy. Biomaterials 271, 120739. doi:10.1016/j.biomaterials.2021.120739
Yao, X., Yang, B., Wang, S., Dai, Z., Zhang, D., Zheng, X., et al. (2020). A Novel Multifunctional FePt/BP Nanoplatform for Synergistic Photothermal/photodynamic/chemodynamic Cancer Therapies and Photothermally-Enhanced Immunotherapy. J. Mater. Chem. B 8 (35), 8010–8021. doi:10.1039/d0tb00411a
Yao, X., Yang, P., Jin, Z., Jiang, Q., Guo, R., Xie, R., et al. (2019). Multifunctional Nanoplatform for Photoacoustic Imaging-Guided Combined Therapy Enhanced by CO Induced Ferroptosis. Biomaterials 197, 268–283. doi:10.1016/j.biomaterials.2019.01.026
Youn, Y. S., and Bae, Y. H. (2018). Perspectives on the Past, Present, and Future of Cancer Nanomedicine. Adv. Drug Deliv. Rev. 130, 3–11. doi:10.1016/j.addr.2018.05.008
Yue, L., Dai, Z., Chen, X., Liu, C., Hu, Z., Song, B., et al. (2018). Development of a Novel FePt-Based Multifunctional Ferroptosis Agent for High-Efficiency Anticancer Therapy. Nanoscale 10 (37), 17858–17864. doi:10.1039/c8nr05150j
Zhang, F., Li, F., Lu, G.-H., Nie, W., Zhang, L., Lv, Y., et al. (2019). Engineering Magnetosomes for Ferroptosis/Immunomodulation Synergism in Cancer. ACS Nano 13 (5), 5662–5673. doi:10.1021/acsnano.9b00892
Zhang, J., Yang, J., Zuo, T., Ma, S., Xokrat, N., Hu, Z., et al. (2021). Heparanase-driven Sequential Released Nanoparticles for Ferroptosis and Tumor Microenvironment Modulations Synergism in Breast Cancer Therapy. Biomaterials 266, 120429. doi:10.1016/j.biomaterials.2020.120429
Zhang, Y., Fu, X., Jia, J., Wikerholmen, T., Xi, K., Kong, Y., et al. (2020). Glioblastoma Therapy Using Codelivery of Cisplatin and Glutathione Peroxidase Targeting siRNA from Iron Oxide Nanoparticles. ACS Appl. Mater. Inter. 12 (39), 43408–43421. doi:10.1021/acsami.0c12042
Zhao, Y., Zhao, W., Lim, Y. C., and Liu, T. (2019). Salinomycin-Loaded Gold Nanoparticles for Treating Cancer Stem Cells by Ferroptosis-Induced Cell Death. Mol. Pharmaceutics 16 (6), 2532–2539. doi:10.1021/acs.molpharmaceut.9b00132
Zhou, L. L., Guan, Q., Li, W. Y., Zhang, Z., Li, Y. A., and Dong, Y. B. (2021). A Ferrocene‐Functionalized Covalent Organic Framework for Enhancing Chemodynamic Therapy via Redox Dyshomeostasis. Small 17 (32), 2101368. doi:10.1002/smll.202101368
Zhu, J., Dai, P., Liu, F., Li, Y., Qin, Y., Yang, Q., et al. (2020). Upconverting Nanocarriers Enable Triggered Microtubule Inhibition and Concurrent Ferroptosis Induction for Selective Treatment of Triple-Negative Breast Cancer. Nano Lett. 20 (9), 6235–6245. doi:10.1021/acs.nanolett.0c00502
Keywords: cancer therapy, ferroptosis, nanomaterial, ferroptosis inducers, reactive oxygen species
Citation: Shi Z, Zheng J, Tang W, Bai Y, Zhang L, Xuan Z, Sun H and Shao C (2022) Multifunctional Nanomaterials for Ferroptotic Cancer Therapy. Front. Chem. 10:868630. doi: 10.3389/fchem.2022.868630
Received: 03 February 2022; Accepted: 02 March 2022;
Published: 24 March 2022.
Edited by:
Yan Zhang, University of Jinan, ChinaReviewed by:
Xin Pang, First Affiliated Hospital of Zhengzhou University, ChinaLisi Xie, Sun Yat-sen Memorial Hospital, China
Copyright © 2022 Shi, Zheng, Tang, Bai, Zhang, Xuan, Sun and Shao. This is an open-access article distributed under the terms of the Creative Commons Attribution License (CC BY). The use, distribution or reproduction in other forums is permitted, provided the original author(s) and the copyright owner(s) are credited and that the original publication in this journal is cited, in accordance with accepted academic practice. No use, distribution or reproduction is permitted which does not comply with these terms.
*Correspondence: Huimin Sun, hmsun@xah.xmu.edu.cn; Chen Shao, cshao@xah.xmu.edu.cn
†These authors have contributed equally to this work