- 1Chongqing Key Laboratory of Green Synthesis and Application, College of Chemistry, Chongqing Normal University, Chongqing, China
- 2Chongqing Key Laboratory of Inorganic Functional Materials, College of Chemistry, Chongqing Normal University, Chongqing, China
- 3Chongqing College of Electronic Engineering, Chongqing, China
In this work, a 4’-(4-cynaophenyl)-4,2’:6′,4-terpyridine supported CuI MOFs photocatalyst (CuI MOF) was applied to the photocatalytic CO2 reduction for the first time. The micro-structural and physicochemical properties of the CuI MOF were systematically studied by the powder X-ray diffraction (PXRD), Single crystal X-ray diffraction (SCXRD), scanning electron microscope (SEM), X-ray photoelectron spectroscopy (XPS), Fourier transform infrared (FT-IR), UV-Vis diffuse spectroscopy (UV-vis DRS), ns-level photoluminescence spectra (ns-level PL), Ultraviolet photoelectron spectroscopy (UPS), and N2 adsorption-desorption test (BET-BJH). Moreover, the in situ diffuse reflectance infrared fourier transform spectroscopy (in situ DRIFTS) was applied to investigate the adsorption and reaction intermediates of photocatalytic CO2 reduction. As a result, CuI MOF exhibited good performance and outstanding selectivity toward photocatalytic reduction of CO2 to CO under full-spectrum and visible light illumination. Notably, 100% selective photocatalytic conversion of CO2 to CO was achieved. Thus, the study presents the high selectivity and CO2 reduction efficiency of CuI MOF as a potential family of photocatalysts.
Introduction
Photocatalytic CO2 reduction has been regarded as an especially promising approach in light of generating valuable chemical fuels to confront the waste gas CO2 issues (Chen et al., 2021; Jamjoum et al., 2021). Moreover, owing to its advantages such as the simplicity of utilization, good reusability, low cost, high efficiency and environmental friendliness, various photocatalysts have been designed and applied in photocatalytic CO2 reduction (Xuan et al., 2020). However, a large amount of photocatalysts still suffers from poor light absorption capacity, high recombination of photo-generated carriers and low selectivity of product, which limits their practical applications in photocatalytic CO2 reduction (Fu et al., 2019). Especially, selectivity of product plays the key role during photocatalytic CO2 reduction. As we all known, photocatalytic CO2 reduction involves a multi-electron reaction to generate a wide variety of products, including CO, CH4, CH3OH and HCOOH, etc., as well as even higher hydrocarbons (Li et al., 2019; Abbas and Sial, 2021) as carbon is in its highest oxidation state. Therefore, catalytic challenge is the precise tuning of the electron density to cater high selectivity. Attempts have been focused on the production of redox photosensitizers to transfer the excited electrons for the catalytic reduction of CO2. Although the first row transition metal, namely Fe, Co and Ni complexes (Cárdenas-Arenas et al., 2020; Zha et al., 2020; Liu et al., 2021), have been adopted to the substitution of low abundance metal analogues (such as Ru, Re, Os and Ir) (Deng et al., 2018; Deng et al., 2021; Karmakar et al., 2021) as multi-electron catalysts, their quick deactivation excited states (owing to the low lying d-d excited state) has limited the catalytic performance of the CO2 reduction. Very recently, the heteroleptic CuI coordination compounds has gained more attention due to their long lifetimes, showing strong metal-to-ligand (MLCT) excited state emission even in a solution at room temperature dominated by the CuI center’s d10 configuration nature (Yamazaki et al., 2019). Thus, the fine tuning of electron density around the metal center is of great significance. The photophysics and photochemistry of remote substituent effects in coordination compounds have been explored in the past by many groups (Fernández-Terán and Sévery, 2021a). Among them, Fernández-Terán and coworkers adopted 4‘-(4-substituted-phenyl)-terpyridine bearing substituents of different electrondonating abilities allowed the remote control of the electron density on the ligands. As the result, the readily tuning of ground- and excited-state properties of the resulting coordination compounds shows the potential of the terpyridine frameworks for high activity/selectivity of photocatalytic reduction chemistry (Fernández-Terán and Sévery, 2021b).
Meanwhile, metal-organic frameworks (MOFs) often possess high thermal and chemical stabilities and allow the incorporation of desired organic ligands featuring various electron-donating abilities through self-assembly and have been gradually applied in the field of catalysis (Zhang and Lin, 2014). Among the reported examples, Cu-based MOFs are highly concerned for its low-price and abundance in nature. In addition, Cu-MOF-based materials have gained extensive attentions as MOF-based catalysts for photocatalytic CO2 reduction. Wang et al. (Wang X.-K. et al., 2019). Reported that [Cu3(TCA)2 (dpe)3(H2O)3]n material for photocatalytic CO2 reduction with the mixture products being CO and H2. Although the material exhibited good CO2 reduction activity (CO yield: 68.0 μmol g−1·h−1), the CO selectivity was merely 22.6%. He et al. (He and Wang, 2018). Fabricated Cu3(BTC)2-based photocatalysts which can efficiently reduce CO to a mixture of CO and CH4 (preferential product). Wang et al. (Wang L. et al., 2019). Synthesized PCN-224(Cu) for the photocatalytic reduction of CO2 under liquid-solid system, which possessed good light harvesting ability. The main reduction products of PCN-224(Cu) were CH4 and CO. However, although these Cu-MOF-based catalysts exhibited high CO2 reduction activity, the selectivity was poor. Therefore, it is highly desirable to further regulate Cu MOF catalysts to meet the high selectivity. While, similar to traditional heterogeneous and homogeneous catalysts, the reported CuI-MOFs catalysts supported by non-terpyridine ligands also suffer from low CO2 photocatalytic activity/selectivity. This evokes us to study the photocatalytic CO2 reduction activity and selectivity of CuI-MOFs supported by terpyridine ligands given the aforementioned high activity/selectivity potential of photocatalytic reduction. Thus, the 4’-(4-cynaophenyl)-4,2’:6′,4-terpyridine (L) supported CuI metal-organic-framework (CuI MOF) reported by Hu and coworkers in 2005 is a good candidate in which L features a V-shaped large π-conjugated nonlinear structure. In addition, the cyano group in L could be substituted by different electron donating ability groups, allowing the remote control of the electron density to cater the high selectivity of multiple CO2 reduction products (Xi et al., 2015).
In this work, we adopted CuI MOF as photocatalyst and its photocatalytic CO2 reduction activity and selectivity have been studied. It is notable that the CuI MOF photocatalyst achieved 100% selcetive conversion of CO2 to CO. Furthermore, UV-vis DRS results indicated that CuI MOF possesses good light absorption ability (200–800 nm). According to UPS and BET-BJH of CuI MOF, suitable reduction potential position and high specific surface areas contributed to the high activity of the photocatalytic CO2 reduction. Finally, in situ DRIFT spectra revealed the possible mechanism of photocatalytic CO2 reduction of CuI MOF. The synthesis of the CuI MOF was modified and optimized to allow the delivery of smaller size of the crystalline material. This work not only demonstrated the outstanding photocatalytic CO2 reduction activity/selectivity of the terpyridine ligand supported CuI heteroleptic coordination compounds but also provide a promising strategy for potentially tuning of photocatalytic CO2 reduction selectivity product based on the ligand substituent group induced rich-electrondonating-diversity featuring terpyridine ligand supported CuI-MOFs.
Experimental section
Materials
N,N-dimethylacetamide (DMA), ethanol, potassium hydroxide (KOH), ammonia solution and methanol were purchased from Chengdu kelong chemical Co., Ltd. Copper cyanide (CuCN), 4-acetylpyridine and 4-formylbenzonitrile were purchased from Shanghai macklin biochemical Co., Ltd. All reagents were directly used as received without further purification.
Synthesis of L
Terpyridine ligand L was synthesized according to the literature method (Xi et al., 2015).
Synthesis of CuI MOF
In a schlenk flask, the mixture of CuCN (1 mmol, 0.09 g), L (2 mmol, 0.70 g) and DMA (20 ml) was stirred under reflux for 12 h. Subsequently, the yellowish green suspension was allowed to cool down to room temperature and washed with DMA (2 × 20 ml), ethanol (2 × 20 ml) and deionized water (2 × 20 ml), in order, by centrifugation. Finally, the product was dried at 80 °C for 12 h (Yield: 0.54g, 67.9%). Anal. Calcd for C108H63Cu9N27 (%): C, 56.09; H, 2.73; N, 16.36. Found: C, 56.43; H, 2.89; N, 16.41.
Activation of CuI MOF
The as-obtained CuI MOF sample needed be further activated (3 steps). Step 1: 0.30 g of CuI MOF was dispersed in 30 ml of DMA with 1 h stirring. Then, the suspension was transferred into a 50 ml Teflon-lined autoclave and heated to 80 °C for 24 h. Step 2: After the CuI MOF sample cooled down, the obtained catalyst was collected by centrifugation and activated again with ethanol. The activated steps were similar with Step 1, except that conditions was heated to 70 °C for 12 h. Step 3: After the sample cooled to room temperature, the obtained CuI MOF was collected by centrifugation and washed by ethanol (3 × 20 ml) and deionized water (3 × 20 ml). Next, the sample was dispersed with 5 ml of deionized water and frozen by liquid nitrogen. Finally, the sample was freeze-dried for 24 h.
Characterization and analytical methods
The phase structure of sample was investigated by powder X-ray diffraction (PXRD: model D/max RA, Rigaku Co., Japan). Single crystal X-ray diffraction (SCXRD: Rigaku Oxford Diffraction, Rigaku Co., Japan) was applied to analyze single crystal structure. The morphology and micro-structure were studied with a scanning electron microscope (SEM: JEOL model JSM-6490, Japan). Fourier transform infrared (FT-IR) spectroscopy were implemented by the PerkinElmer Spectrum Two (U.K.), using KBr pellet and analyzing from 400 to 4,000 cm−1. The quantum efficiency and charge carrier lifetime of CuI MOF were conducted by a fluorescent spectrophotometer (FLS1000, Edinburgh Instruments, U.K.) with 450 nm of excited wavelength. The UV-Vis diffuse spectroscopy (UV-vis DRS: UV2550PC, Shimadzu, Japan) was used to characterize the optical properties. Ultraviolet photoelectron spectroscopy (UPS) measurements (Thermo Fisher Scientific) were carried out on valence band with using a He I (hν = 21.2 eV) source. The surface chemical compositions and valence states were analyzed by X-ray photoelectron spectroscopy (XPS) measurements (K-alpha, Thermo Scientific). The specific surface area and pore volume of the sample were measured by the N2 adsorption-desorption specific surface analyzer (BET, BeiShiDe Instrument, BSD-PS). The elemental analysis for C, H, N were performed on a Perkin-elmer 240C analytical instrument.
Photocatalytic CO2 reduction
The photocatalytic CO2 reduction experiment was evaluated using the Labsolar-6A system (Beijing Perfectlight). Before light irradiation, 10 mg of the catalyst was dispersed in 2 ml of pure water, and dropped by droplet onto a fiberglass filter and dried for using. After placing the dried sample in the reactor, the reactor was evacuated until no O2 or N2 could be detected via gas chromatography (kechuang, GC 2002). Then, the reactor filled with high-purity CO2 (≥99.999%, Chongqing lituoqiti Co., Ltd) several times and added 100 μL of deionized water, after which the reaction system pressure in the reactor was maintained at 90.0 ± 1.0 kPa. Then the reactor was exposed to the Xe lamp (lamp current: 20 A, PLS-SXE300+) full-spectrum light illumination for 8 h. The temperature of the entire reaction system was maintained at 20 ± 0.03°C through a recirculating cooling water system. The gaseous products were analyzed every 1 h on the gas chromatograph equipped with a methanizer, flame ionization detector (FID), and thermal conductivity detector (TCD), which could detect CO, CH4, and H2, O2, N2, respectively.
In situ DRIFTS investigation on photocatalytic CO2 reduction
In situ DRIFTS measurements (diffuse reflectance infrared Fourier transform spectra) were conducted on a Nicolet iS50FT-IR spectrometer (Thermo Fisher, USA) equipped with a designed reactor and a liquid nitrogen cooled HgCdTe (MCT) detector. Then the loaded samples were purged with Ar (50 mlmin−1) for 1 h at 120°C to remove all the impurities. After the reactor temperature was dropped to room temperature, the background spectrum was collected. Next, the mixed gas (25 mlmin−1 of Ar, 5 mlmin−1 of CO2 and a trace of H2O vapor) was introduced into the reactor for about 30 min until reaching sorption equilibrium before illumination. Furthermore, the background spectrum was recorded again. Afterward, the turn on the light illumination (300 W Xe Lamp, AM 1.5 G) and the FTIR spectra were recorded as a function of time to investigate the dynamics of the conversion of the reactants under illumination.
Results and discussion
Phase structure
As shown in Figure 1A, the characteristic diffraction peaks can be well matched with single crystal simulation (SIM) patterns, which not only indicating the successful synthesis of CuI MOF, but also conformed the phase purity of it. Notably, the considerably sharp diffraction peaks of CuI MOF reveals the good crystallinity. The structure illustration of CuI MOF was presented in Supplementary Figure S1 and Figure 1B. CuI MOF is a three dimensional interpretation structure. The asymmetric unit comprised by nine CuI ions, four and a half ligands and nine cyanide ions with all the bond lengths and angles are identical to the structure reported (Xi et al., 2015).
Micro-structure and morphology
SEM was carried out to explore the micro-structures and morphologies of the CuI MOF. As depicted in Figure 2A, the as-obtained CuI MOF demonstrates irregular and fluffy porous structure. Figure 2B further confirms that the tremella-like morphology of CuI MOF is self-assembled by plenty of the stacked nanosheets and nanoparticles.
FT-IR spectra
The FT-IR spectra of the as-prepared CuI MOF, CuCN and L were illustrated in Figure 3. The significant peaks at 1,590 and 1,600 cm−1 were attributed to the C=C/C=N stretching vibration of pyridine (Singh et al., 2021). The band at 2,126 and 2,160 cm−1 were ascribed to the C≡N stretching vibration (Zhang et al., 2018). Compared to CuCN (2,160 cm−1) and L (1,590 cm−1), the C≡N and C=C/C=N characteristic peaks of CuI MOF shifted, respectively, suggesting that CuCN and L are coodinated. Moreover, the existence of CuCN and L characteristic peaks of CuI MOF sample indicated that CuI MOF was successfully synthesized by this method.
XPS
The XPS was carried out to investigate the surface chemical compositions and valence states of CuI MOF sample. As can be seen from Figure 4A, the XPS survey spectrum reveals the presence of C, N, O and Cu elements in CuI MOF sample. In the high resolution Cu 2p spectra (Figure 4B), the main peaks at 952.6 and 932.8 eV are assigned to CuI (Zhu et al., 2021). To further identify CuI, Cu LMM Auger spectrum as shown in Figure 4F. The Cu Auger LMM peak was observed at 571.9 eV (Jiang et al., 2022) in the binding energy scale, which was corresponded to the characteristic peak of CuI. These results show that the valance of copper existed as CuI in CuI MOF. As displayed in Figure 4C, the peaks at 285.7 and 284.8 eV in the high resolution C 1s spectra are ascribed to the C-N and C=C/C-C bond in the L (Zhu et al., 2021). The high resolution N 1s spectra of CuI MOF sample is provided in Figure 4D. Binding energy at 399.3 and 398.6 eV are indexed to Cu-N bond (Zhu et al., 2021) and pyridinic N (Singh et al., 2021), respectively. The formation of Cu-N bond proved that the Cu elements were successfully coordinated with the cyano groups and the N in the L. The high resolution O 1s spectra is demonstrated in Figure 4E. It can be found that the binding energies at 533.4 and 531.8 eV correspond to O-H bonds of surface absorption water and Cu-O (H2O) interactions (Liu et al., 2021), respectively.
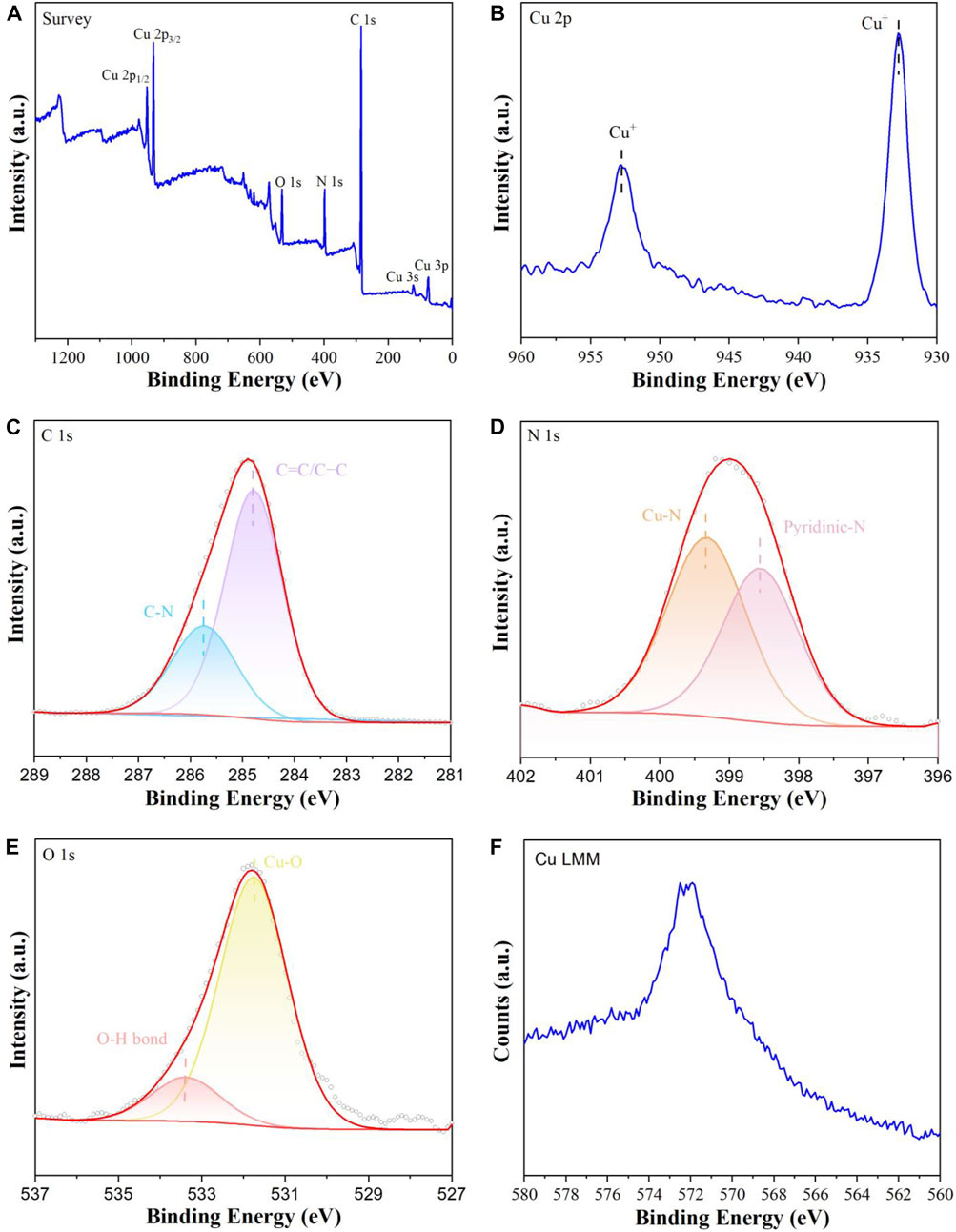
FIGURE 4. (A) XPS survey spectra of CuI MOF, high resolution spectra of (B) Cu 3d, (C) C 1s, (D) N 1s, (E) O 1s, (F) Cu LMM Auger spectrum for in CuI MOF.
UV-vis DRS and ns-level PL
The UV-vis diffuse reflectance spectra of CuI MOF have been conducted to evaluate its light absorption ability (Figure 5A). CuI MOF displayed good absorption ability in the ultraviolet and visible region, which is in good consistent with the color of CuI MOF sample (inset of Figure 5A). In Figure 5B, the band gap of CuI MOF has been calculated from the intercept of the tangents to the plots of (αhν)1/2 νs. Photo energy is 2.36 eV. The time-resolved PL spectra as depicted in the Figure 5C, the carrier lifetime of CuI MOF is 0.08 ns? Furthermore, the quantum efficiency of CuI MOF is 1.32% under visible light (450 nm) illumination.
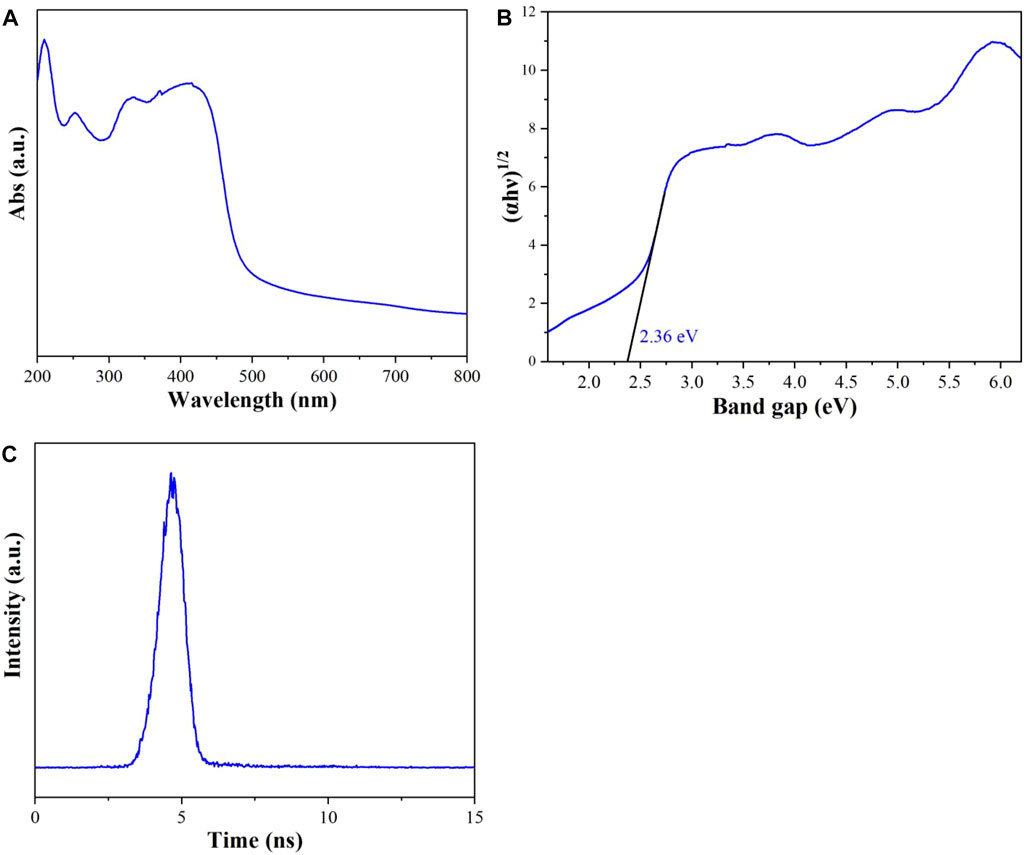
FIGURE 5. (A) UV-vis diffuse reflectance spectra, (B) plots of (αhν)1/2 νs. Photo energy and (C) time-resolved PL spectra monitored at under 450 nm excitation at 298 K for CuI MOF.
UPS
UPS was conducted to investigate the position of valence band (VB) and conduction band (CB). The abscissa is the binding energy which is relative to the Fermi energy (EF) of Au. It is defined by the energy of the electron before excitation relative to the vacuum level. The high binding energy cutoff (Ecutoff) of CuI MOF is illustrated in Figure 6A. Ecutoff is decided by linear extrapolation to zero of the yield of secondary electrons. In Figure 6A, Ecutoff = 16.3 ± 0.03 eV for CuI MOF. The HOMO region for CuI MOF is observed in Figure 6B. The HOMO energy is decided using the incident photon energy, hν = 21.2 eV, Ecutoff, and the Eonset (the onset of CuI MOF relative to the EF of Au). In Figure 6B, Eonset = 0.98 ± 0.03 eV for CuI MOF. The HOMO energy is thus gained directly from the UPS measurement, EHOMO = hν-(Ecutoff - Eonset) (Gong et al., 2011; Sun et al., 2011; Zheng et al., 2018).
For CuI MOF, EHOMO = -5.88 ± 0.06 eV. The LUMO energy is calculated using the HOMO levels and the optical gaps (Eg) obtained from UV-Vis DRS (Figure 5B). Thus, the ELUMO = -3.52 ± 0.06 eV is for CuI MOF. Concequnently, the photo-generated electrons of CuI MOF could reduce CO2 to CO. According to the characterization results of UPS and UV-vis diffuse reflectance spectra (Figure 5A, Figure 6), the diagram of band structure is shown in Figure S4.
BET-BJH
As can be seen from Figure 7A, the N2 adsorption-desorption isotherm of CuI MOF exhibits typical IV isotherms with H3 type hysteresis loop, which indicates the existence of mesopores. The pore size distribution curve further demonstrates the existence of mesopores (Figure 7B). The specific surface area, corresponding pore volume and average pore diameter are 116.98 m2 g−1, 0.77 cm3 g−1 and 26.40 nm respectively. The high specific surface area and large pore volume could provide more active sites for the reactant adsorption and photocatalytic reaction, which is in agreement with SEM result.
Photocatalytic performance
The experiment of full-spectrum light (AM 1.5 G) driven CO2 reduction was performed to evaluate the photocatalytic activity. Before the beginning of photocatalytic CO2 reduction, no CO and other organic matter are detected under the reaction conditions of without light, photocatalyst and with Ar atomosphere, respectively, demonstrating that CO2 is the sole carbon source for the reaction. As shown in Figure 8A, it is interesting that the CO concentration was found to increase gradually with the extension of the illumination time. After 8 h of photocatalytic reaction, no other product can be detected additional to CO, which suggests that the selectivity of CO production is 100%. It is worthwhile mentioning that the yield rate of CO is 2.58 μmol g−1·h−1 under 8 h full-spectrum light illumination. Remarkably, CuI MOF also shows a good visible light photocatalytic activity for CO2 reduction, whose yield rate of CO is 1.83 μmol g−1·h−1 under 8 h illumination (Figure 8B). Moreover, the photocatalytic stability of CuI MOF was assessed through three sequent tests of photocatalytic CO2 reduction for 24 h (Supplementary Figure S2). The yield of CO dropped from about 2.58 to 0.87 μmol g−1·h−1, which suggested poor photocatalytic stability. The poor photocatalytic stability could be due to photo-destabilization of functional groups (Feng et al., 2020), which decomposed the framework of CuI MOF and caused poor photocatalytic stability.
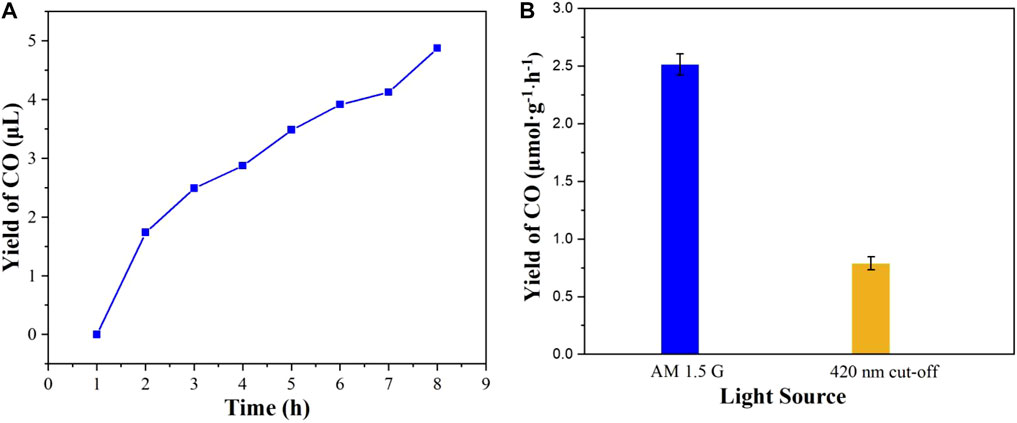
FIGURE 8. (A) The yields of CO reduced by CO2 under AM 1.5 G illumination and (B) the yields rate of CO under different light source illumination.
Mechanisms of photocatalytic CO2 reduction
As can be seen in Figure 9A, the peaks at 2,333, 2,345 and 2,369 cm−1 are indexed to CO2 (Coenen et al., 2018) and the peaks at 1,632 and 3,000–3,600 cm−1 are attributed to H2O (Tang et al., 2019; Zhao Y. et al., 2020), respectively. Furthermore, the intensities of the peaks increase with prolonged absorption time, which indicates that CO2 molecules are activated on the surface of CuI MOF.
In Figure 9B, after light turned on, a number of intermediates are detected, including HCO3− (825 and 1,358 cm−1) (Liu et al., 2017; Ma et al., 2017), b-CO32− (1,554 cm−1) (Tang et al., 2019), m-CO32− (1,494 and 1,532 cm−1) (Liu et al., 2017), −COOH (1,591 cm−1) (Zhao J. et al., 2020) and CO (2,162 and 2,222 cm−1) (Ma et al., 2017). Moreover, the intensities of thoes peaks gradually enhanced with the extension of illumination time. Accordingly, the possible CO2 conversion pathway was proposed as following:
Conclusion
In summary, the synthesis of CuI MOF was successfully optimized for photocatalytic CO2 reduction. The CO evolution reached 2.58 μmol g−1·h−1 and achieved 100% conversion. Moreover, UV-vis DRS result indicates that CuI MOF displays good light absorption capacity (200–800 nm). The BET-BJH result reveals that CuI MOF possesses high specific surface area (116.98 m2 g−1) and large pore volume (0.77 cm3 g−1). The position of conduction band (-3.52 ± 0.06 eV) of CuI MOF is negative enough to meet the photocatalytic CO2 reduction. Finally, the possible mechanisms of photocatalytic CO2 reduction were revealed by the in situ DRIFTS. This study demonstrated the promising potential of terpyridine ligand supported CuI-MOFs for the high activity/selectivity of photocatalytic CO2 reduction.
Data availability statement
The original contributions presented in the study are included in the article/Supplementary Material; further inquiries can be directed to the corresponding authors.
Author contributions
W-DZ and Y-ZM designed the experiments. YW, A-LJ, HG, and X-YT carried out photocatalyst CuI MOF synthesis. W-SF, J-ZL, and PC worked on the material property data analysis.
Funding
This research is financially supported by the National Natural Science Foundation of China (Grant No. 51708078), Natural Science Foundation of Chongqing (Grant No. cstc2021jcyj-msxm2068) the Science and Technology Research Program of Chongqing Municipal Education Commission (Grant No. KJZD-K201900502), Chongqing Talent Program (Leading Talent), and the Chongqing Innovative Research Group Project (Grant No. CXQT21015, CQYC201903221).
Conflict of interest
The authors declare that the research was conducted in the absence of any commercial or financial relationships that could be construed as a potential conflict of interest.
Publisher’s note
All claims expressed in this article are solely those of the authors and do not necessarily represent those of their affiliated organizations, or those of the publisher, the editors and the reviewers. Any product that may be evaluated in this article, or claim that may be made by its manufacturer, is not guaranteed or endorsed by the publisher.
Supplementary material
The Supplementary Material for this article can be found online at: https://www.frontiersin.org/articles/10.3389/fchem.2022.974907/full#supplementary-material
References
Abbas, M., and Sial, M. A. Z. G. (2021). New Horizon in stabilization of single atoms on metal-oxide supports for CO2 reduction. Nano Mater. Sci. 3, 368–389. doi:10.1016/j.nanoms.2021.07.009
Cárdenas-arenas, A., Quindimil, A., DAVó-QUIñONERO, A., BAILóN-GARCíA, E., Lozano-CASTELLó, D., De-La-Torre, U., et al. (2020). Isotopic and in situ DRIFTS study of the CO2 methanation mechanism using Ni/CeO2 and Ni/Al2O3 catalysts. Appl. Catal. B Environ. 265, 118538. doi:10.1016/j.apcatb.2019.118538
Chen, P., Zhang, Y., Zhou, Y., and Dong, F. (2021). Photoelectrocatalytic carbon dioxide reduction: Fundamental, advances and challenges. Nano Mater. Sci. 3, 344–367. doi:10.1016/j.nanoms.2021.05.003
Coenen, K., Gallucci, F., Mezari, B., Hensen, E., and Van Sint Annaland, M. (2018). An in-situ IR study on the adsorption of CO2 and H2O on hydrotalcites. J. CO2 Util. 24, 228–239. doi:10.1016/j.jcou.2018.01.008
Deng, L. M., Hu, F., Ma, M. Y., Huang, S. C., Xiong, Y. X., Chen, H. Y., et al. (2021). Electronic modulation caused by interfacial Ni-O-M (M=Ru, Ir, Pd) bonding for accelerating hydrogen evolution kinetics. Angew. Chem. Int. Ed. Engl. 60, 22450–22456. doi:10.1002/ange.202110374
Deng, X. Y., Albero, J., Xu, L. Z., Garcia, H., and Li, Z. H. (2018). Construction of a stable Ru-Re hybrid system based on multifunctional MOF-253 for efficient photocatalytic CO2 reduction. Inorg. Chem. 57, 8276–8286. doi:10.1021/acs.inorgchem.8b00896
Feng, L., Wang, K. Y., Day, G. S., Ryder, M. R., and Zhou, H. C. (2020). Destruction of metal-organic frameworks: Positive and negative aspects of stability and lability. Chem. Rev. 120, 13087–13133. doi:10.1021/acs.chemrev.0c00722
Fernández-terán, R. J., and Sévery, L. (2021b). Coordination environment prevents access to intraligand charge-transfer states through remote substitution in rhenium(I) terpyridinedicarbonyl complexes. Inorg. Chem. 60, 1325–1333. doi:10.1021/acs.inorgchem.0c02914
Fernández-terán, R., and Sévery, L. (2021a). Living long and prosperous: Productive intraligand charge-transfer states from a rhenium(I) terpyridine photosensitizer with enhanced light absorption. Inorg. Chem. 60, 1334–1343. doi:10.1021/acs.inorgchem.0c01939
Fu, H.-C., You, F., Li, H.-R., and He, L.-N. (2019). CO2 capture and in situ catalytic transformation. Front. Chem. 7, 525. doi:10.3389/fchem.2019.00525
Gong, X., Tong, M., Brunetti, F. G., Seo, J., Sun, Y., Moses, D., et al. (2011). Bulk heterojunction solar cells with large open-circuit voltage: Electron transfer with small donor-acceptor energy offset. Adv. Mat. 23, 2272–2277. doi:10.1002/adma.201003768
He, X., and Wang, W.-N. (2018). MOF-Based ternary nanocomposites for better CO2photoreduction: Roles of heterojunctions and coordinatively unsaturated metal sites. J. Mat. Chem. A Mat. 6, 932–940. doi:10.1039/c7ta09192c
Jamjoum, H. A. A., Umar, K., Adnan, R., Razali, M. R., and Mohamad Ibrahim, M. N. (2021). Synthesis, characterization, and photocatalytic activities of graphene oxide/metal oxides nanocomposites: A review. Front. Chem. 9, 752276. doi:10.3389/fchem.2021.752276
Jiang, G., Peng, M., Hu, L., Ouyang, J., Lv, X., Yang, Z., et al. (2022). Electron-deficient Cuδ+ stabilized by interfacial Cu–O-Al bonding for accelerating electrocatalytic nitrate conversion. Chem. Eng. J. 435, 134853. doi:10.1016/j.cej.2022.134853
Karmakar, S., Barman, S., Rahimi, F. A., and Maji, T. K. (2021). Covalent grafting of molecular photosensitizer and catalyst on MOF-808: Effect of pore confinement toward visible light-driven CO2 reduction in water. Energy Environ. Sci. 14, 2429–2440. doi:10.1039/d0ee03643a
Li, X., Yu, J., Jaroniec, M., and Chen, X. (2019). Cocatalysts for selective photoreduction of CO2 into solar fuels. Chem. Rev. 119, 3962–4179. doi:10.1021/acs.chemrev.8b00400
Liu, H., Liu, Z., Yi, J., Ma, D., Xia, F., Tian, D., et al. (2021). A dual-signal electroluminescence aptasensor based on hollow Cu/Co-MOF-luminol and g-C3N4 for simultaneous detection of acetamiprid and malathion. Sensors Actuators B Chem., 331, 129412. doi:10.1016/j.snb.2020.129412
Liu, L., Zhao, C., Miller, J. T., and Li, Y. (2017). Mechanistic study of CO2 photoreduction with H2O on Cu/TiO2 nanocomposites by in situ X-ray absorption and infrared spectroscopies. J. Phys. Chem. C 121, 490–499. doi:10.1021/acs.jpcc.6b10835
Ma, Z., Li, P., Ye, L., Zhou, Y., Su, F., Ding, C., et al. (2017). Oxygen vacancies induced exciton dissociation of flexible BiOCl nanosheets for effective photocatalytic CO2 conversion. J. Mat. Chem. A Mat. 5, 24995–25004. doi:10.1039/c7ta08766g
Singh, A. K., Jaiswal, N., Gautam, R. K., and Tiwari, I. (2021). Development of g-C3N4/Cu-DTO MOF nanocomposite based electrochemical sensor towards sensitive determination of an endocrine disruptor BPSIP. J. Electroanal. Chem. 887, 115170. doi:10.1016/j.jelechem.2021.115170
Sun, Y., Takacs, C. J., Cowan, S. R., Seo, J. H., Gong, X., Roy, A., et al. (2011). Efficient, air-stable bulk heterojunction polymer solar cells using MoOx as the anode interfacial layer. Adv. Mat. 23, 2226–2230. doi:10.1002/adma.201100038
Tang, J.-Y., Guo, R.-T., Pan, W.-G., Zhou, W.-G., and Huang, C.-Y. (2019). Visible light activated photocatalytic behaviour of Eu (III) modified g-C3N4 for CO2 reduction and H2 evolution. Appl. Surf. Sci. 467-468, 206–212. doi:10.1016/j.apsusc.2018.10.143
Wang, L., Jin, P., Huang, J., She, H., and Wang, Q. (2019a). Integration of copper(II)-Porphyrin zirconium metal–organic framework and titanium dioxide to construct Z-scheme system for highly improved photocatalytic CO2 reduction. ACS Sustain. Chem. Eng. 7, 15660–15670. doi:10.1021/acssuschemeng.9b03773
Wang, X.-K., Liu, J., Zhang, L., Dong, L.-Z., Li, S.-L., Kan, Y.-H., et al. (2019b). Monometallic catalytic models hosted in stable metal–organic frameworks for tunable CO2 photoreduction. ACS Catal. 9, 1726–1732. doi:10.1021/acscatal.8b04887
Xi, Y., Wei, W., Xu, Y., Huang, X., Zhang, F., and Hu, C. (2015). Coordination polymers based on substituted terpyridine ligands: Synthesis, structural diversity, and highly efficient and selective catalytic oxidation of benzylic C–H bonds. Cryst. Growth & Des. 15, 2695–2702. doi:10.1021/acs.cgd.5b00008
Xuan, X., Chen, S., Zhao, S., Yoon, J. Y., Boczkaj, G., and Sun, X. (2020). Carbon nanomaterials from metal-organic frameworks: A new material horizon for CO2 reduction. Front. Chem. 8, 573797. doi:10.3389/fchem.2020.573797
Yamazaki, Y., Onoda, T., Ishikawa, J., Furukawa, S., Tanaka, C., Utsugi, T., et al. (2019). Photocatalytic CO(2) reduction using various heteroleptic diimine-diphosphine Cu(I) complexes as photosensitizers. Front. Chem. 7, 288. doi:10.3389/fchem.2019.00288
Zha, Q. Q., Li, M. X., Liu, Z. H., and Ni, Y. H. (2020). Hierarchical Co, Fe-MOF-74/Co/carbon cloth hybrid electrode: Simple construction and enhanced catalytic performance in full water splitting. ACS Sustain. Chem. Eng. 8, 12025–12035. doi:10.1021/acssuschemeng.0c02993
Zhang, D., Han, X., Dong, T., Guo, X., Song, C., and Zhao, Z. (2018). Promoting effect of cyano groups attached on g-C3N4 nanosheets towards molecular oxygen activation for visible light-driven aerobic coupling of amines to imines. J. Catal. 366, 237–244. doi:10.1016/j.jcat.2018.08.018
Zhang, T., and Lin, W. B. (2014). Metal-organic frameworks for artificial photosynthesis and photocatalysis. Chem. Soc. Rev. 43, 5982–5993. doi:10.1039/c4cs00103f
Zhao, J., Wang, T., Deng, J., Shu, C.-M., Zeng, Q., Guo, T., et al. (2020a). Microcharacteristic analysis of CH4 emissions under different conditions during coal spontaneous combustion with high-temperature oxidation and in situ FTIR. Energy 209, 118494. doi:10.1016/j.energy.2020.118494
Zhao, Y., Shi, T., Shang, J., Ding, L., Cao, X., Chen, C., et al. (2020b). Rapid proton exchange between surface bridging hydroxyls and adsorbed molecules on TiO2, Appl. Catal. B Environ., 277. 119234, doi:10.1016/j.apcatb.2020.119234
Zheng, L., Zhu, T., Xu, W., Liu, L., Zheng, J., Gong, X., et al. (2018). Solution-processed broadband polymer photodetectors with a spectral response of up to 2.5 μm by a low bandgap donor–acceptor conjugated copolymer. J. Mat. Chem. C Mat. 6, 3634–3641. doi:10.1039/c8tc00437d
Keywords: photocatalytic CO2 reduction, high selectivity, terpyridine ligand, CuI MOF, photocatalyst
Citation: Zhang W-D, Wang Y, Liang Y, Jiang A-L, Gong H, Tian X-Y, Fu W-S, Liao J-Z, Chen P and Ma Y-Z (2022) High selectivity of photocatalytic reduction of CO2 to CO based on terpyridine ligand supported CuI metal organic framework. Front. Chem. 10:974907. doi: 10.3389/fchem.2022.974907
Received: 21 June 2022; Accepted: 06 July 2022;
Published: 05 August 2022.
Edited by:
Jianping Sheng, University of Electronic Science and Technology of China, ChinaReviewed by:
Yuxin Zhang, Chongqing University, ChinaGuangming Jiang, Chongqing Technology and Business University, China
Copyright © 2022 Zhang, Wang, Liang, Jiang, Gong, Tian, Fu, Liao, Chen and Ma. This is an open-access article distributed under the terms of the Creative Commons Attribution License (CC BY). The use, distribution or reproduction in other forums is permitted, provided the original author(s) and the copyright owner(s) are credited and that the original publication in this journal is cited, in accordance with accepted academic practice. No use, distribution or reproduction is permitted which does not comply with these terms.
*Correspondence: Ying-Zhao Ma, eXptYUBjcW51LmVkdS5jbg==; Wen-Sheng Fu, ZnV3ZW5zaGVuZ0BjcW51LmVkdS5jbg==; Peng Chen, Y3RidXBlbmdjaGVuQDEyNi5jb20=
†These authors have contributed equally to this work and share first authorship