3D porous polymers for selective removal of CO2 and H2 storage: experimental and computational studies
- 1Chemistry Department, King Fahd University of Petroleum and Minerals, Dhahran, Saudi Arabia
- 2Interdisciplinary Research Center for Membranes and Water Security, King Fahd University of Petroleum and Minerals, Dhahran, Saudi Arabia
- 3Interdisciplinary Research Center for Hydrogen and Energy Storage, King Fahd University of Petroleum and Minerals, Dhahran, Saudi Arabia
- 4Chemical Engineering Department, King Fahd University of Petroleum and Minerals, Dhahran, Saudi Arabia
Abstract
In this article, newly designed 3D porous polymers with tuned porosity were synthesized by the polycondensation of tetrakis (4-aminophenyl) methane with pyrrole to form M1 polymer and with phenazine to form M2 polymer. The polymerization reaction used p-formaldehyde as a linker and nitric acid as a catalyst. The newly designed 3D porous polymers showed permanent porosity with a BET surface area of 575 m2/g for M1 and 389 m2/g for M2. The structure and thermal stability were investigated by solid 13C-NMR spectroscopy, Fourier-transform infrared (FT-IR) spectroscopy, and thermogravimetric analysis (TGA). The performance of the synthesized polymers toward CO2 and H2 was evaluated, demonstrating adsorption capacities of 1.85 mmol/g and 2.10 mmol/g for CO2 by M1 and M2, respectively. The importance of the synthesized polymers lies in their selectivity for CO2 capture, with CO2/N2 selectivity of 43 and 51 for M1 and M2, respectively. M1 and M2 polymers showed their capability for hydrogen storage with a capacity of 66 cm3/g (0.6 wt%) and 87 cm3/g (0.8 wt%), respectively, at 1 bar and 77 K. Molecular dynamics (MD) simulations using the grand canonical Monte Carlo (GCMC) method revealed the presence of considerable microporosity on M2, making it highly selective to CO2. The exceptional removal capabilities, combined with the high thermal stability and microporosity, enable M2 to be a potential material for flue gas purification and hydrogen storage.
1 Introduction
Global warming caused by the elevated levels of CO2 has garnered significant attention in recent years. The elevated levels of CO2 have become a serious problem due to their hazardous effects on the environment; these effects encompass a gradual increase in the temperature of the earth, resulting in droughts, fluctuations in the weather, and elevated oceanic water levels and ocean acidification (Feldman et al., 2015; Leal et al., 2018; Zakeri et al., 2022). During the last 40 years, the concentration of CO2 has increased tremendously from 319 ppm to 414 ppm in 2021, setting a new record, and is estimated to increase to 800 ppm within the next 100 years if we continue relying on fossil fuels as a primary energy source (Mercer, 1978; Feldman et al., 2015; Abdelhakim et al., 2022). Fossil fuels, serving as energy sources, are typically divided into three types: natural gas, coal, and petroleum. Upon combustion, they release CO2, SOx, and NOx gases; mercury; and various particulates that cause pollution in the environment and have a large impact on human health (Khraisheh et al., 2020; Perera and Nadeau, 2022). Due to the major concern for the environment and human health, several methods and techniques have been identified to reduce the effect of CO2 (Taylor et al., 2020; Long et al., 2021). These techniques and methods include finding new sources of energy, such as hydrogen gas, as an alternative energy source, reducing energy consumption by increasing energy efficiency, and finding new methods for capturing CO2 (Kar et al., 2022; Paramati et al., 2022). Capturing CO2 is one of these methods and has garnered considerable attention over the years. Two major methods have been used: chemisorption of CO2, which involves the formation of a chemical bond between CO2 and the adsorbent. Such an example for chemisorption is the absorption of CO2 by liquid amines which is the most commonly used method by refineries to capture CO2 from natural gas streams, their operation is non-costeffective and requires high energy for regeneration. Furthermore, degradation of the liquid amines thermally and oxidatively causes corrosion in refinery setups (Bobek et al., 2016; Dey et al., 2017; Kong et al., 2019). The other major technique that is emerging is capturing CO2 by physisorption. Physisorption is a process where CO2 is bonded weakly with the adsorbent by weak van der Waals forces of attraction, which allows the sorbent to be capable of reversibly adsorbing CO2 from flue gas streams by solid sorbents (Plaza et al., 2007; Oschatz and Antonietti, 2018; Kong et al., 2019). Solid sorbents have been developed through the years, and several key features should be included in the design of these sorbents for efficient CO2 capture, such as i) high sorption capacity, ii) selectivity, and iii) adequate stability in the presence of contaminants (Zou et al., 2017; Abdelnaby et al., 2019; Khraisheh et al., 2020). Different classes of solid sorbents have emerged as promising materials for CO2 reduction, such as metal–organic frameworks (MOFs), covalent organic frameworks (COFs), zeolites, carbonaceous materials, such as activated carbon, and porous organic polymers (POPs) (Cheung and Hedin, 2014; Gadipelli and Guo, 2015; Lohse and Bein, 2018; Zhao et al., 2018; Qasem et al., 2020). POPs are an interesting class of materials that possess excellent features such as low density, high surface area with a tunable pore size distribution, good thermal and chemical stability, and synthetic versatility (Zou et al., 2017; Gao et al., 2019; Gu et al., 2022). These features are considered requirements for the selective removal of CO2 from flue gas and natural gas streams (Rufford et al., 2012; Ahmed et al., 2015; Alloush et al., 2022). In our endeavor to design and synthesize porous organic polymers for CO2 capture and hydrogen storage, we demonstrate the design and synthesis of new 3D porous organic polymers with tuned porosity in this study. The synthesized 3D polymers were evaluated for their CO2 and H2 adsorption capabilities and for their selectivity of CO2 over N2 and CH4 to assess their potential use in flue gas and natural gas treatment.
2 Experimental
2.1 Materials and methods
Tetrakis (4-aminophenyl) methane (99%), phenazine (99%), and pyrrole (98%) were all purchased from Sigma-Aldrich Co. p-Formaldehyde (PF, ≥99.9% purity) was purchased from Fluka™ AG. Nitric acid (65%wt.) and N,N-dimethylformamide (DMF, 99% purity) were obtained from Alpha Chemika™. Methanol (MeOH, ≥99.9% purity) was acquired from Merck Millipore™. Except for pyrrole, which was distilled at 150°C immediately before use, all chemicals were used as received. Ultrahigh-purity-grade nitrogen (N2, 99.999%), helium (He, 99.999%), and high-purity carbon dioxide (CO2, 99.9%) gases were supplied by Abdullah Hashem Industrial Co., Saudi Arabia. Natural abundance solid-state 13C-NMR spectra were collected using a Bruker 400 MHz spectrometer set to 125.65 MHz at room temperature (11.74 T). Samples were packed into 4 mm zirconium oxide rotors. Cross-polarization and high-power decoupling were used. The pulse delay was 2.5 s, and the magic angle spinning rate was 10 kHz. A PerkinElmer FT-IR spectrometer was used to obtain FT-IR spectra. FT-IR spectra were obtained in the range of 4,000–400 cm−1 using a PerkinElmer 16F PC FT-IR spectrometer and solid potassium bromide (KBr) pellets (mid-IR region). TGA was performed using the STA 429® (NETZSCH group, Germany) thermal analyzer. All gas uptake measurements were performed on the Quantachrome® Autosorb IQ instrument, and isotherms were obtained at 273 K and 298 K.
2.2 Synthesis
In a typical experiment (Abdelnaby et al., 2018), tetrakis (4-aminophenyl) methane (2.73 × 10−3 mol, 1.0 g) and pyrrole (0.0109 mol, 0.73 g) were stirred in a 50-mL round-bottomed flask equipped with a magnetic bar containing 25 mL DMF until a homogeneous solution was obtained. p-Formaldehyde (0.02187 mol, 0.66 g) and nitric acid (10% of p-formaldehyde; 0.002187 mol, 0.199 g) were then added to the reaction mixture. The reaction mixture was flushed with N2 gas and sealed and stirred for 24 h at 90°C. Once the reaction was completed, the product was filtered and washed with methanol for 3 days with continuous exchange of methanol to ensure the removal of any monomers or unreacted materials left in the reaction. The product was vacuum-dried at 90°C for 24 h to get M1 as a fine black powder (yield % = 65%). M2 was obtained as a bright yellow powder (yield % = 49%) under similar reaction conditions with tetrakis (4-aminophenyl) methane, phenazine (M2), and p-formaldehyde taken at a molar ratio of 1:4:8 and 10 mol% of nitric acid relative to p-formaldehyde. The yield of the polymerization reaction was calculated as the mass of the product relative to the mass of all reactants.
2.3 MD simulation procedure
Molecular dynamics simulations (Supplementary Material) were performed to reveal the underlying mechanism of adsorption of CO2, CH4, and N2 gases by the polymers M1 and M2. The structural geometries of the polymers were built and optimized using the smart algorithm in the Forcite module of Materials Studio 8.0 software. The COMPASS II force field (Sun et al., 2016) was adopted, while the self-consistent field (SCF) convergence threshold, maximum force tolerance, and energy tolerance were set to 1.0 × 10−5 Ha, 0.001 Ha/Å, and 1.0 × 10−5 Ha, respectively. Thereafter, using the “Locate” task bar on the Sorption module, the suitable adsorption sites of the gases on M1 and M2 were identified, and the adsorption capacities were estimated based on the principle of simulated annealing using the GCMC method (Aljamaan et al., 2017; Song et al., 2018). The adsorption isotherms at 273.15, 298.15, and 313.15 K were calculated using the Langmuir fitting equation:
where a is the limit of adsorption capacity in mmol/g and b is the adsorption constant in MPa−1. The estimated adsorption capacities were given in the units of average molecules/cell and were converted to the amount of gas adsorbed in mmol/g using the following equation (Zhang et al., 2021):
where Mwcell is the relative molecular mass of M1 and M2 polymers in the constructed supercell.
3 Results and discussion
3.1 Synthesis and characterization
This paper describes two new 3D porous amine-based polymers. The polymerization method was based on a modified Mannich polycondensation reaction, with tetrakis (4-aminophenyl) methane added as a common component in the polymers. The polymers were realized by polymerizing tetrakis (4-aminophenyl) methane with pyrrole to obtain M1 and phenazine to form M2. The polymerization reaction was conducted using DMF as a solvent and concentrated HNO3 as a catalyst (Scheme 1).
The structural features of the polymers were characterized by solid 13C-NMR, as shown in Figure 1A. The peaks residing from 100 ppm to 150 ppm correspond to the aromatic carbons of pyrrole, phenazine, and tetrakis (4-aminophenyl) methane. A peak at 65 ppm corresponds to the quaternary carbon of tetrakis (4-aminophenyl) methane, linking the four aniline moieties. A peak at 55 ppm corresponds to the methylene linkage (-CH2-) between tetrakis (4-aminophenyl) methane and pyrrole or phenazine. A peak at ∼30 ppm corresponds to the methylene linkage (-CH2-) present between pyrrole and pyrrole moieties (Luo et al., 2012; Abdelnaby et al., 2018). Figure 1B represents the FT-IR spectra for the synthesized polymers. The figure shows a broadband in the region from 3,300 to 3,500 cm−1 resulting from the overlap between both the 1° amine (-NH2) stretching vibrations of the aniline moiety in the tetrakis (4-aminophenyl) methane monomer and the 2° amine (-NH-) stretching band of the pyrrole moiety. The bands between 1,400 and 1,700 cm−1 correspond to the aromatic -C=C- and -C=N- stretching vibrations of phenazine, pyrrole, and aniline moieties. A band at 1,631 cm−1 attributed to -NH2 scissoring can be observed overlapping with the -C=C- aromatic vibrational bands that appear in the same region. Both -NH2 and -NH- wagging bands are at 694 and 755 cm−1, respectively (Wu et al., 2002; Tian et al., 2009). Figure 1C shows the powder X-ray diffraction patterns of the 3D porous polymers. The powder X-ray diffraction patterns reveal the amorphous nature of the synthesized polymers with a broad signal at ∼15° 2Ө with some degree of crystallinity shown by the signal at ∼ 22° 2Ө present in M1 and M2 (Wei et al., 1992; Errahali et al., 2014). Figure 1D reveals the good thermal stability of the synthesized polymers, which could be related to the stiff cross-linked structures of M1 and M2. The thermograms in Figure 4D show a 5% weight loss of small, trapped molecules in M2 up to 200°C, followed by a second degradation at ∼500°C, where the degradation of the polymer structure occurs by the loss of the methylene linkages, followed by the degradation of the polymer backbone. On the other hand, M1 begins to thermally degrade at ∼300°C up to 440°C, which may be attributed to the loss of the methylene linkages between the moieties, followed by the complete degradation of the polymer structure at ∼600°C (Li et al., 2014; Yan et al., 2016).
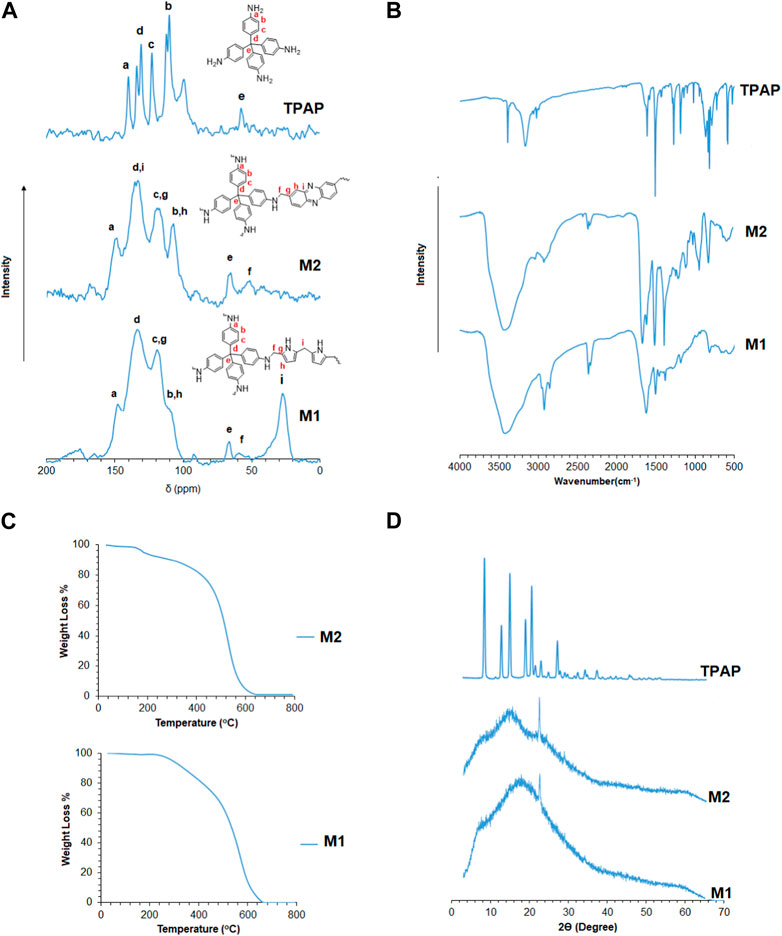
FIGURE 1. (A) Solid 13C-NMR CP/MAS spectra for 3D porous polymers. (B) FT-IR spectra for 3D porous polymers and identification of peaks. (C) Thermogravimetric analysis of 3D porous polymers. (D) Powder X-ray diffraction of 3D porous polymers.
3.2 Porosity of the 3D porous polymers
As shown in Figure 2A, the nitrogen adsorption/desorption isotherms suggest that M1 and M2 are porous in nature. M1 polymer shows permanent porosity with a BET surface area of 575 m2/g. The BET isotherm of M1 suggests that the polymer exhibits Type I characteristics with a steep increase in nitrogen uptake at low relative pressure (P/P0 < 0.05). The hysteresis in the M1 isotherm suggests the high interaction between M1 porous polymer and N2 molecules, which could be due to the entrapment of N2 molecules in the pores of M1 that leads to the hysteresis found in the adsorption/desorption isotherm (Li et al., 2022). The pore size distribution analysis based on density functional theory (DFT) calculations reveals two distinct regions in the M2 porous polymer. As shown in Figure 2B, there is a prominent peak at approximately 10 Å, indicating the presence of micropores, and another strong peak at an average pore width of approximately 33 Å, representing the mesoporous region. M2 polymer shows a permanent porosity with a BET surface area of 389 m2/g. The nitrogen adsorption isotherm of M2 suggests that the polymer is microporous in nature and exhibits Type I characteristics. Further examination using DFT calculations reveals that the apertures of M2 polymer are mainly in the range of micropores with pore widths less than 20 Å, as shown in Figure 2B.
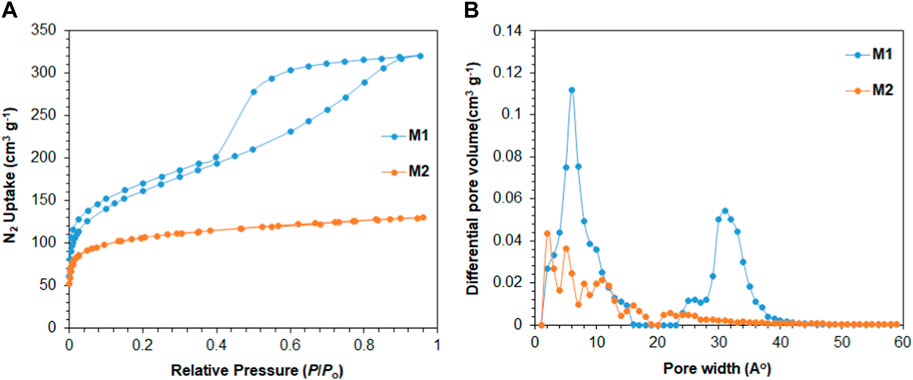
FIGURE 2. (A) Nitrogen adsorption/desorption isotherms of 3D porous polymers at 77 K; (B) pore size distribution using DFT.
The tuned pore size distribution, accompanied by the good surface areas, encouraged us to investigate the capabilities of M1 and M2 for CO2 adsorption compared with CH4 and N2 for applications in natural gas purification and flue gas treatment. For the polymers to perform well, they should be selective toward CO2 and that can be produced by enhancing the microporosity of the polymer. As shown in Figure 2B, M2 is microporous in nature, with pore size distributions falling in the microporous region less than 20 Å with a high intensity close to the kinetic diameter of CO2 (3.3 Å). This is shown by the adsorption capacities observed in Figures 3A–D, where the adsorption capacity at 273 K of CO2 is higher in M2 (2.1 mmol/g) compared to M1 (1.85 mmol/g). At 298 K, the adsorption capacities behave in a similar manner, where the adsorption capacity of M2 for CO2 is 1.41 mmol/g, for CH4 is 0.44 mmol/g, and for N2 is 0.050 mmol/g, whereas the adsorption capacity of M1 for CO2 is 1.24 mmol/g, for CH4 is 0.32 mmol/g, and for N2 is 0.08 mmol/g. Comparing the efficiency between M1 and M2, it is shown that the adsorption capacity of M2 was higher than M1, which is attributed to the microporous nature of the pores and the absence of mesopores in M2 (Song et al., 2022). The isosteric heat of adsorption (Qst) of CO2 shows the interaction energy between a sorbent and CO2 gas. Figures 3E, F show the Qst vs uptake of CO2. The values of Qst decrease with the coverage of the surface of the polymer with CO2, indicating that the adsorption process occurred on a heterogeneous surface. The Qst values for the adsorption of CO2 by M1 and M2 were found to be 33.1 kJ/mol and 33.6 kJ/mol, respectively. This indicates that the adsorption process is of physisorption in nature (Khosrowshahi et al., 2022; Ravi et al., 2023). Another feature that an adsorbent should possess is high selectivity. As shown in Figures 4A–D, the selectivity was investigated at 298 K to mimic ambient conditions, which is in agreement with post-combustion treatment conditions. By using the initial slope ratios of Henry’s law constants at 298 K, the selectivity of M1 for CO2/N2 is 43 and CO2/CH4 is 9, whereas the selectivity of M2 for CO2/N2 is 51 and CO2/CH4 is 10. The selectivity of M2 was higher than that of M1 even though it has a lower surface area, which could be explained by the microporous nature of the polymer with a similar observation for CO2/CH4 selectivity. As shown in Table 1, despite having lower surface areas, M1 and M2 exhibit superior adsorption capacity and selectivity for CO2/CH4 and CO2/N2 compared to reported porous materials with higher surface areas.
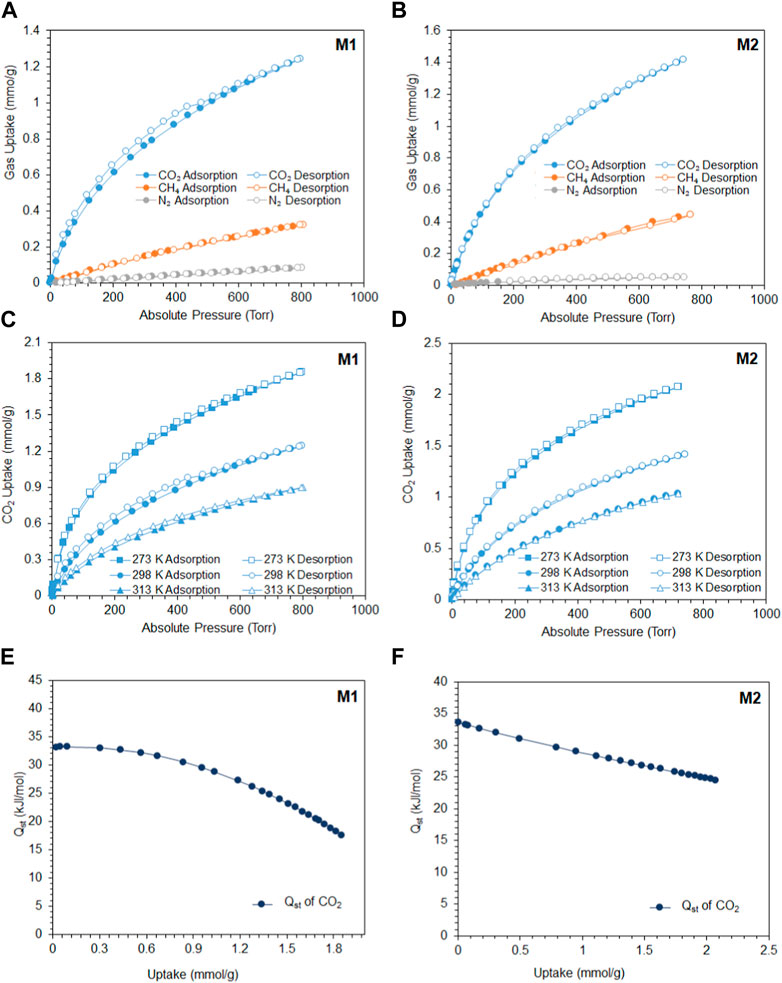
FIGURE 3. (A) Adsorption/desorption of M1 to CO2, CH4, and N2 at 298 K; (B) adsorption/desorption of M2 to CO2, CH4, and N2 at 298 K; (C) M1 adsorption/desorption of CO2 at 273, 298, and 313 K; (D) M2 adsorption/desorption of CO2 at 273, 298, and 313 K (filled circles refer to adsorption, and unfilled circles refer to desorption); (E) M1 isosteric heat of adsorption (Qst) vs CO2 uptake (mmol/g); and (F) M2 isosteric heat of adsorption (Qst) vs CO2 uptake (mmol/g).
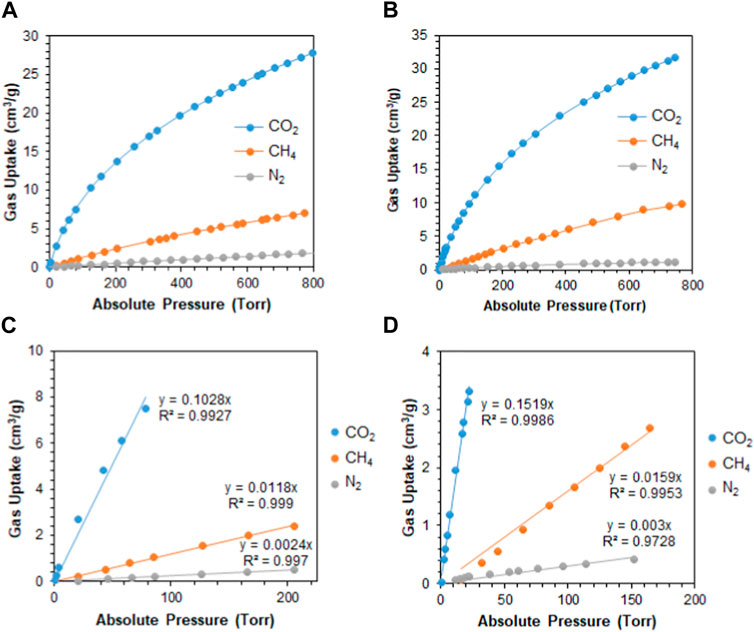
FIGURE 4. (A) Adsorption of CO2, CH4, and N2 by M1 at 298 K; (B) adsorption of CO2, CH4, and N2 by M2 at 298 K; (C) initial slope fitting of M1 at 298 K; and (D) initial slope fitting of M2 at 298 K.
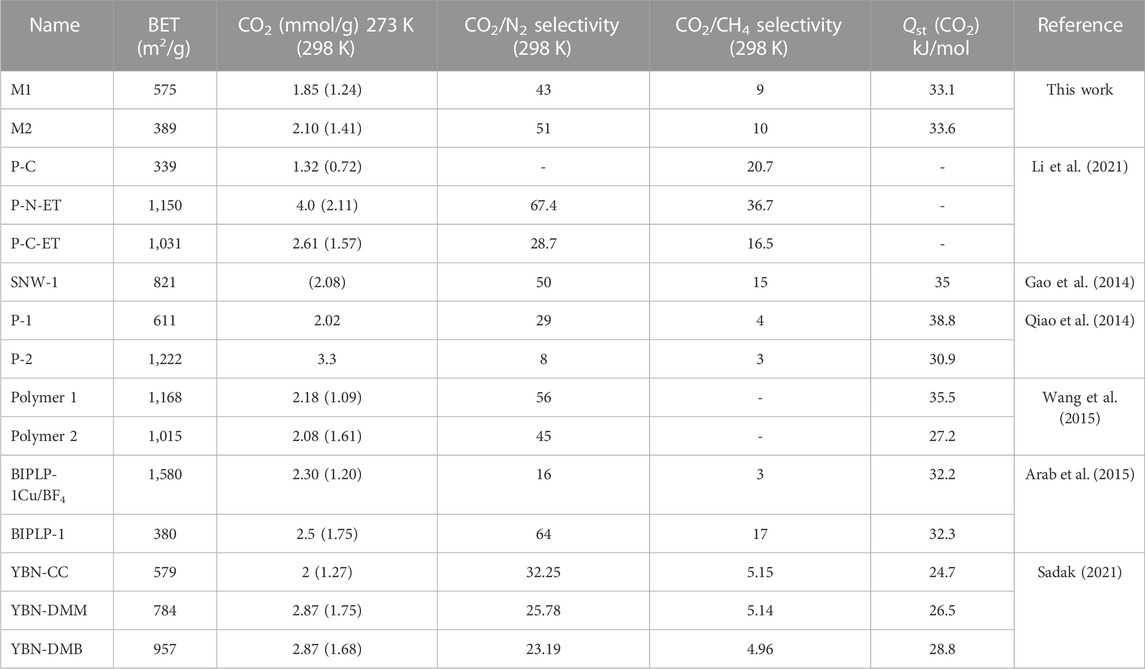
TABLE 1. Comparison of porous materials with M1 and M2 with respect to surface area, CO2 uptake at 273 K and 298 K, CO2/N2 and CO2/CH4 selectivity, and Qst (CO2).
The molecular dynamics of the synthesized polymers were studied to correlate the experimental results with the theoretical calculations (Supplementary Material). The adsorption of single-component gases, CO2, CH4, and N2, on M1 and M2 polymers at 298.15 K was simulated in supercells of dimension 30 × 30 × 40 Å, comprising of 20 repeating units of the polymer molecules, as presented in Figures 5A–C. The corresponding simulated adsorption isotherms are shown in Figures 5D, E. Both polymers demonstrated strong van der Waals attraction toward CO2 molecules via the pyrrolic and pyridinic nitrogen atoms on M1 and M2, respectively. Moreover, CO2 adsorption binding sites were located on both molecules, with fewer sites for CH4 and N2 gases. However, M2 demonstrated rapid uptake of CO2 below 2 MPa (20 bar), indicating the presence of microporosity within the polymer framework (Rizzuto et al., 2017), and the selectivity of the polymer to CO2 gas is consistent with the experimental findings. Using the Langmuir isotherm model (Table 2), the limit adsorption capacities of CO2 at 298.15 K on M1 and M2 were estimated as 2.99 and 3.74 mmol/g, while for CH4 and N2, the values were 0.44 and 0.17, and 0.98 and 0.20 mmol/g, respectively. Meanwhile, the corresponding theoretical isosteric heat of adsorption (Qst) for CO2 at 298.15 K was calculated as 43.1 and 43.9 kJ/mol on M1 and M2, respectively. While the theoretical values are slightly higher than the experimental values, which could be ascribed to the overestimation from the general assumptions input into the simulation software (Meng et al., 2018). The order of selectivity of the polymers is in good agreement with the experimental findings and revealed the preferential selectivity of M2 to CO2 gas. The adsorption of CO2 on M1 and M2 at temperatures of 273.15, 298.15, and 313.15 K was further investigated, and the results are presented in Figures 6A, B. A slight decrease in the adsorption capacity of both polymers was observed with increasing temperature. This suggests that the adsorption of the gas molecules is strictly dependent on the van der Waals force of attraction between them and the active sites on the polymers, which tend to weaken with the increase in temperature due to the increase in the inherent kinetic energy of the gas molecules. Thus, M2 experiences less decline in the adsorption capacity, suggesting its greater adsorption preference for CO2 gas.
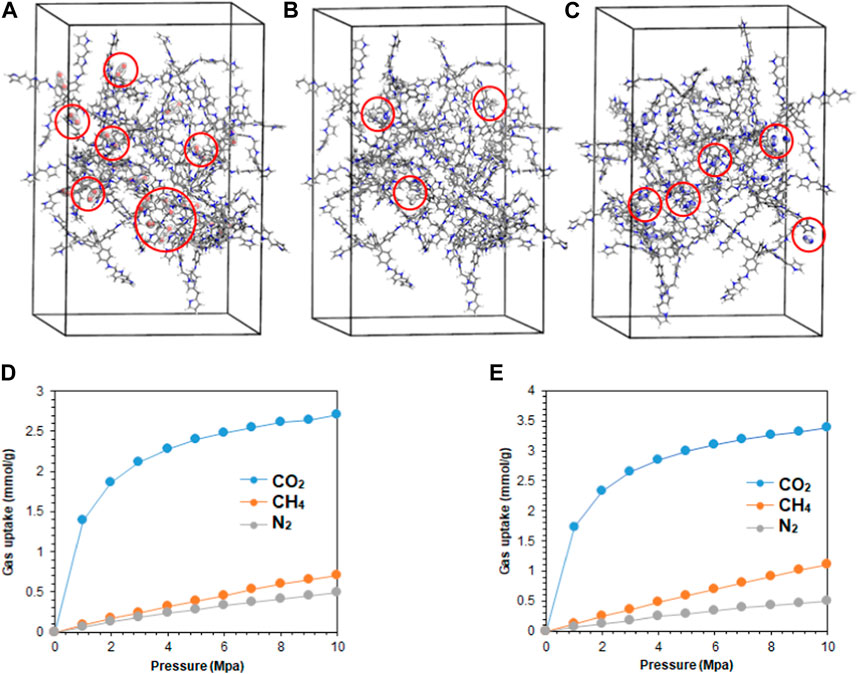
FIGURE 5. (A) CO2, (B) CH4, and (C) N2 adsorption sites located on the M2 polymer packed in amorphous cells of dimension 30 × 30 × 40 Å, comprising 20 repeating units. The red spheres represent the adsorbed gas molecules. The corresponding simulated adsorption isotherms for both polymers at 298.15 K are presented in (D) M1 and (E) M2.
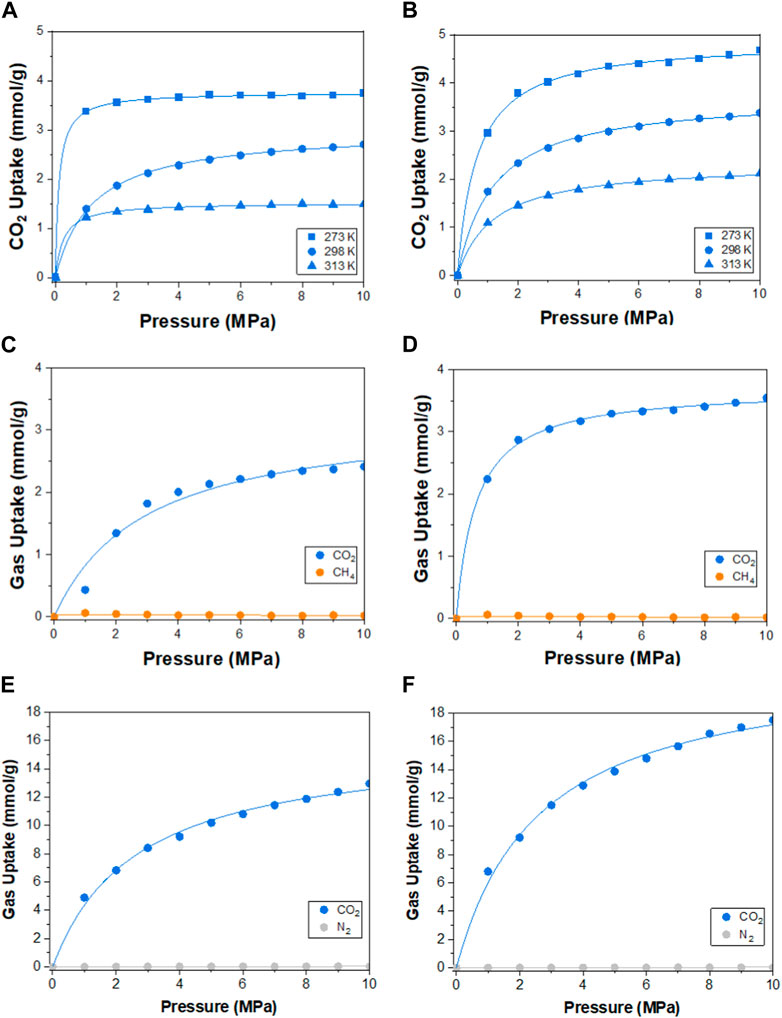
FIGURE 6. Theoretical adsorption isotherms of CO2 at 273.15, 298.15, and 313.15 K on (A) M1 and (B) M2. Adsorption isotherms of CO2/CH4 in multi-component streams with a molar ratio of 50:50 on (C) M1 and (D) M2 at 298.15 K; and CO2/N2 with a molar ratio of 20:80 on (E) M1 and (F) M2 are also presented.
Meanwhile, the selectivity of the polymers toward CO2 in multi-component gas streams, comprising CO2 and CH4 at a molar ratio of 50:50 and CO2 and N2 gases at a molar ratio of 20:80, was further explored theoretically, as shown in Figures 6C–F. The presence of equimolar volumes of CH4 and the abundance of N2 gases did not impede the selectivity of the polymers to CO2 gas, as the presence of quadrupole C=O bonds favors the van der Waals attraction to the polymers. On the other hand, CH4 and N2 molecules experienced a drastic decrease in adsorption affinity by the polymers due to the strong competition by CO2 molecules, resulting in fewer molecular interactions, as shown in Figures 6C–F. In all cases, the M2 polymer rapidly adsorbs CO2 molecules in the presence of competing gas molecules, affirming its selectivity and aligning well with the experimental findings.
In our endeavor to tap into the world of clean energy and seek alternatives to overcome the pollution of petroleum products, we assessed our polymers for their capability to store hydrogen gas. The results in Figure 7A revealed the adsorption capacity of M1 and M2 toward H2 at 77 K and 1 atm to be 66 cm3/g (0.6 wt%) and 87 cm3/g (0.8 wt%), respectively. Interestingly, the absence of mesopores in M2 reflected the higher adsorption capacity toward H2 compared to M1, which is consistent with Rong et al. (2021). The storage capacity is higher and comparable to that of porous polymers with similar or higher surface areas, as shown in Table 3. Finally, we simulate the potential of hydrogen storage on the polymer molecules by constructing supercells and conducting the grand canonical Monte Carlo simulation, as shown in Figures 7B, C. The theoretical H2 uptake capacities of M1 and M2 at 77 K were calculated to be 61.7 and 83.2 cm3/g, respectively. These values are in good agreement with the experimental adsorption capacities of both polymers and corroborate the microporosity of M2, making it a potential material for H2 storage.
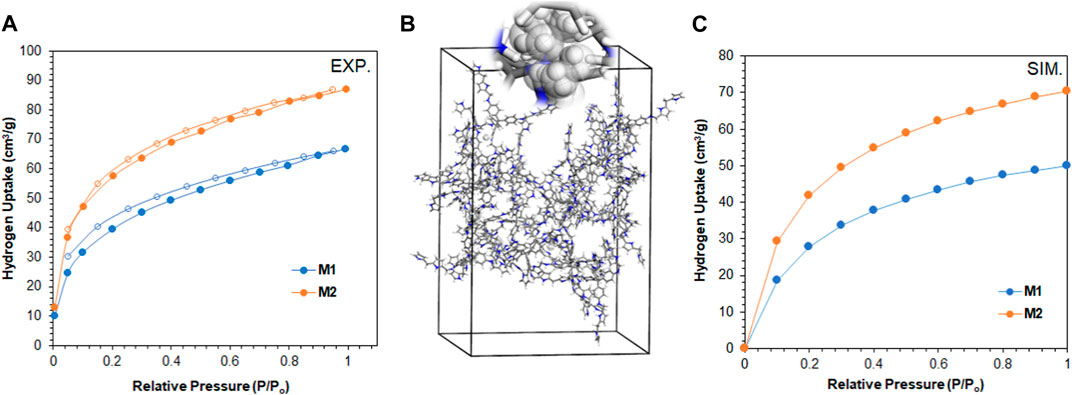
FIGURE 7. (A) H2 adsorption/desorption isotherm of M1 and M2 at 77 K (filled circles refer to adsorption, and unfilled circles refer to desorption). (B) Supercell of dimension 30 × 30 × 40 Å, comprising 20 repeating units of M2 for the simulation of H2 storage capacity and (C) theoretical adsorption isotherms of H2 at 77 K on M1 and M2.
4 Conclusion
In this study, we report the synthesis of 3D porous polymers with tuned porosity. The choice of reaction conditions and monomers leads to polymers with microporous and meso-/microporous structures. The produced polymers were found to be thermally stable up to temperatures of 400°C. Analysis revealed the porous nature of polymers with a BET surface area of 575 m2/g for M1 and 389 m2/g for M2. The M1 polymer showed defined micropores of 7 Å and mesopores of 33 Å, whereas M2 exhibited micropores with a pore size distribution of <20 Å. The study also revealed the effect of microporosity on adsorption ability and selectivity. The results revealed superior performance of M2 in the absence of mesoporosity. The adsorption capacities at 273 K of CO2 are higher in M2 (2.1 mmol/g) compared to M1 (1.85 mmol/g). In addition, at 298 K, the adsorption capacity of M2 for CO2 was 1.41 mmol/g, for CH4 was 0.44 mmol/g, and for N2 was 0.050 mmol/g, while the adsorption capacity of M1 for CO2 was 1.24 mmol/g, for CH4 was 0.32 mmol/g, and for N2 was 0.08 mmol/g. Furthermore, the absence of mesoporosity in M2 was evidenced by its superior performance in hydrogen storage. The molecular dynamics simulation confirmed the superior performance of M2 and coincided with the experimental values to prove the efficiency and capability of porous polymers to be a potential adsorbent for selective removal of CO2 and H2 storage.
Data availability statement
The original contributions presented in the study are included in the article/Supplementary Material; further inquiries can be directed to the corresponding author.
Author contributions
MA-B: Data curation, Investigation, Writing–original draft. IAb: Methodology, Software, Writing–original draft, Writing–review and editing. MA: Data curation, Methodology, Writing–original draft, Writing–review and editing. IAl: Data curation, Writing–original draft, Writing–review and editing. OA: Conceptualization, Data curation, Formal Analysis, Funding acquisition, Investigation, Methodology, Project administration, Resources, Software, Supervision, Validation, Visualization, Writing–original draft, Writing–review and editing.
Funding
The authors declare that financial support was received for the research, authorship, and/or publication of this article.
Acknowledgments
This article is supported by King Fahd University of Petroleum and Minerals. The Authors at KFUPM acknowledge the Interdisciplinary Research Center for Hydrogen and Energy Storage for the support received under Grant number: INHE2207.
Conflict of interest
The authors declare that the research was conducted in the absence of any commercial or financial relationships that could be construed as a potential conflict of interest.
Publisher’s note
All claims expressed in this article are solely those of the authors and do not necessarily represent those of their affiliated organizations, or those of the publisher, the editors, and the reviewers. Any product that may be evaluated in this article, or claim that may be made by its manufacturer, is not guaranteed or endorsed by the publisher.
Supplementary material
The Supplementary Material for this article can be found online at: https://www.frontiersin.org/articles/10.3389/fchem.2023.1265324/full#supplementary-material
References
Abdelhakim, L. O. A., Zhou, R., and Ottosen, C.-O. (2022). Physiological responses of plants to combined drought and heat under elevated CO2. Agronomy 12 (10), 2526. doi:10.3390/agronomy12102526
Abdelnaby, M. M., Alloush, A. M., Qasem, N. A. A., Al-Maythalony, B. A., Mansour, R. B., Cordova, K. E., et al. (2018). Carbon dioxide capture in the presence of water by an amine-based crosslinked porous polymer. J. Mater. Chem. A 6 (15), 6455–6462. doi:10.1039/c8ta00012c
Abdelnaby, M. M., Qasem, N. A. A., Al-Maythalony, B. A., Cordova, K. E., and Al Hamouz, O. C. S. (2019). A microporous organic copolymer for selective CO2 capture under humid conditions. Acs Sustain Chem. Eng. 7 (16), 13941–13948. doi:10.1021/acssuschemeng.9b02334
Ahmed, A., Babarao, R., Huang, R., Medhekar, N., Todd, B. D., Hill, M. R., et al. (2015). Porous aromatic frameworks impregnated with lithiated fullerenes for natural gas purification. J. Phys. Chem. C 119 (17), 9347–9354. doi:10.1021/acs.jpcc.5b01144
Aljamaan, H., Al Ismail, M., and Kovscek, A. R. (2017). Experimental investigation and Grand Canonical Monte Carlo simulation of gas shale adsorption from the macro to the nano scale. J. Nat. Gas Sci. Eng. 48, 119–137. doi:10.1016/j.jngse.2016.12.024
Alloush, A. M., Abdulghani, H., Amasha, H. A., Saleh, T. A., and Al Hamouz, O. C. S. (2022). Microwave-assisted synthesis of novel porous organic polymers for effective selective capture of CO2. J. Industrial Eng. Chem. 113, 215–225. doi:10.1016/j.jiec.2022.05.049
Arab, P., Verlander, A., and El-Kaderi, H. M. (2015). Synthesis of a highly porous bis(imino)pyridine-linked polymer and its postsynthetic modification with inorganic fluorinated ions for selective CO2 capture. J. Phys. Chem. C 119 (15), 8174–8182. doi:10.1021/acs.jpcc.5b00690
Bobek, J., Rippel-Pethő, D., Molnár, É., and Bocsi, R. (2016). Selective hydrogen sulphide removal from acid gas by alkali chemisorption in a jet reactor. Hung. J. Industry Chem. 44 (1), 51–54. doi:10.1515/hjic-2016-0006
Cheng, G., Hasell, T., Trewin, A., Adams, D. J., and Cooper, A. I. (2012). Soluble conjugated microporous polymers. Angew. Chem. Int. Ed. 51 (51), 12899–12903. doi:10.1002/ange.201205521
Cheung, O., and Hedin, N. (2014). Zeolites and related sorbents with narrow pores for CO2 separation from flue gas. RSC Adv. 4 (28), 14480–14494. doi:10.1039/c3ra48052f
Dey, S., Bhunia, A., Boldog, I., and Janiak, C. (2017). A mixed-linker approach towards improving covalent triazine-based frameworks for CO2 capture and separation. Microporous Mesoporous Mater. 241, 303–315. doi:10.1016/j.micromeso.2016.11.033
Dybtsev, D. N., Chun, H., Yoon, S. H., Kim, D., and Kim, K. (2004). Microporous manganese formate: A simple Metal−Organic porous material with high framework stability and highly selective gas sorption properties. J. Am. Chem. Soc. 126 (1), 32–33. doi:10.1021/ja038678c
Errahali, M., Gatti, G., Tei, L., Paul, G., Rolla, G. A., Canti, L., et al. (2014). Microporous hyper-cross-linked aromatic polymers designed for methane and carbon dioxide adsorption. J. Phys. Chem. C 118 (49), 28699–28710. doi:10.1021/jp5096695
Feldman, D. R., Collins, W. D., Gero, P. J., Torn, M. S., Mlawer, E. J., and Shippert, T. R. (2015). Observational determination of surface radiative forcing by CO2 from 2000 to 2010. Nature 519 (7543), 339–343. doi:10.1038/nature14240
Gadipelli, S., and Guo, Z. X. (2015). Tuning of ZIF-derived carbon with high activity, nitrogen functionality, and yield – a case for superior CO2 capture. ChemSusChem 8 (12), 2123–2132. doi:10.1002/cssc.201403402
Gao, X., Zou, X., Ma, H., Meng, S., and Zhu, G. (2014). Highly selective and permeable porous organic framework membrane for CO2 capture. Adv. Mater. 26 (22), 3644–3648. doi:10.1002/adma.201400020
Gao, H., Li, Q., and Ren, S. (2019). Progress on CO2 capture by porous organic polymers. Curr. Opin. Green Sustain. Chem. 16, 33–38. doi:10.1016/j.cogsc.2018.11.015
Germain, J., Fréchet, J. M. J., and Svec, F. (2009). Nanoporous polymers for hydrogen storage. Small 5 (10), 1098–1111. doi:10.1002/smll.200801762
Gu, J., Shao, P., Luo, L., Wang, Y., Zhao, T., Yang, C., et al. (2022). Microporous triazine-based ionic hyper-crosslinked polymers for efficient and selective separation of H2S/CH4/N2. Sep. Purif. Technol. 285, 120377. doi:10.1016/j.seppur.2021.120377
Kar, S. K., Harichandan, S., and Roy, B. (2022). Bibliometric analysis of the research on hydrogen economy: An analysis of current findings and roadmap ahead. Int. J. Hydrogen Energy 47 (20), 10803–10824. doi:10.1016/j.ijhydene.2022.01.137
Khosrowshahi, M. S., Abdol, M. A., Mashhadimoslem, H., Khakpour, E., Emrooz, H. B. M., Sadeghzadeh, S., et al. (2022). The role of surface chemistry on CO2 adsorption in biomass-derived porous carbons by experimental results and molecular dynamics simulations. Sci. Rep. 12 (1), 8917. doi:10.1038/s41598-022-12596-5
Khraisheh, M., Almomani, F., and Walker, G. (2020). Solid sorbents as a retrofit technology for CO2 removal from natural gas under high pressure and temperature conditions. Sci. Rep. 10 (1), 269. doi:10.1038/s41598-019-57151-x
Kong, X., Li, S., Strømme, M., and Xu, C. (2019). Synthesis of porous organic polymers with tunable amine loadings for CO2 capture: Balanced physisorption and chemisorption. Nanomaterials 9 (7), 1020. doi:10.3390/nano9071020
Leal, P. P., Hurd, C. L., Sander, S. G., Armstrong, E., Fernández, P. A., Suhrhoff, T. J., et al. (2018). Copper pollution exacerbates the effects of ocean acidification and warming on kelp microscopic early life stages. Sci. Rep. 8 (1), 14763. doi:10.1038/s41598-018-32899-w
Li, G., Zhang, B., Yan, J., and Wang, Z. (2014). Micro- and mesoporous poly(Schiff-base)s constructed from different building blocks and their adsorption behaviors towards organic vapors and CO2 gas. J. Mater. Chem. A 2 (44), 18881–18888. doi:10.1039/c4ta04429k
Li, Z., Wang, W., Xu, Y., Zhu, Y., and Guo, X. (2021). Truxene/triazatruxene-based conjugated microporous polymers with flexible@rigid mutualistic symbiosis for efficient CO2 storage. J. CO2 Util. 49, 101550. doi:10.1016/j.jcou.2021.101550
Li, M., Jian, Z., Hassanpouryouzband, A., and Zhang, L. (2022). Understanding hysteresis and gas trapping in dissociating hydrate-bearing sediments using pore network modeling and three-dimensional imaging. Energy and Fuels 36 (18), 10572–10582. doi:10.1021/acs.energyfuels.2c01306
Liao, Y., Cheng, Z., Trunk, M., and Thomas, A. (2017). Targeted control over the porosities and functionalities of conjugated microporous polycarbazole networks for CO2-selective capture and H2 storage. Polym. Chem. 8 (46), 7240–7247. doi:10.1039/c7py01439b
Lohse, M. S., and Bein, T. (2018). Covalent organic frameworks: Structures, synthesis, and applications. Adv. Funct. Mater. 28 (33), 1705553. doi:10.1002/adfm.201705553
Long, Z., Huang, Y., Zhang, W., Shi, Z., Yu, D., Chen, Y., et al. (2021). Effect of different industrial activities on soil heavy metal pollution, ecological risk, and health risk. Environ. Monit. Assess. 193 (1), 20. doi:10.1007/s10661-020-08807-z
Luo, Y., Li, B., Wang, W., Wu, K., and Tan, B. (2012). Hypercrosslinked aromatic heterocyclic microporous polymers: A new class of highly selective CO2Capturing materials.Capturing Mater. 24 (42), 5703–5707. doi:10.1002/adma.201202447
Makhseed, S., Samuel, J., Bumajdad, A., and Hassan, M. (2008). Synthesis and characterization of fluoropolymers with intrinsic microporosity and their hydrogen adsorption studies. J. Appl. Polym. Sci. 109 (4), 2591–2597. doi:10.1002/app.28372
McKeown, N. B., Gahnem, B., Msayib, K. J., Budd, P. M., Tattershall, C. E., Mahmood, K., et al. (2006). Towards polymer-based hydrogen storage materials: Engineering ultramicroporous cavities within polymers of intrinsic microporosity. Angew. Chem. Int. Ed. 45 (11), 1804–1807. doi:10.1002/anie.200504241
Meng, J., Zhong, R., Li, S., Yin, F., and Nie, B. (2018). Molecular model construction and study of gas adsorption of zhaozhuang coal. Energy and Fuels 32 (9), 9727–9737. doi:10.1021/acs.energyfuels.8b01940
Mercer, J. H. (1978). West antarctic ice sheet and CO2 greenhouse effect: A threat of disaster. Nature 271 (5643), 321–325. doi:10.1038/271321a0
Oschatz, M., and Antonietti, M. (2018). A search for selectivity to enable CO2 capture with porous adsorbents. Energy and Environ. Sci. 11 (1), 57–70. doi:10.1039/c7ee02110k
Paramati, S. R., Shahzad, U., and Doğan, B. (2022). The role of environmental technology for energy demand and energy efficiency: Evidence from OECD countries. Renew. Sustain. Energy Rev. 153, 111735. doi:10.1016/j.rser.2021.111735
Perera, F., and Nadeau, K. (2022). Climate change, fossil-fuel pollution, and children’s health. N. Engl. J. Med. 386 (24), 2303–2314. doi:10.1056/nejmra2117706
Plaza, M. G., Pevida, C., Arenillas, A., Rubiera, F., and Pis, J. J. (2007). CO2 capture by adsorption with nitrogen enriched carbons. Fuel 86 (14), 2204–2212. doi:10.1016/j.fuel.2007.06.001
Qasem, N. A. A., Abuelyamen, A., and Ben-Mansour, R. (2020). Enhancing CO 2 adsorption capacity and cycling stability of Mg-MOF-74. Arabian J. Sci. Eng. 46, 6219–6228. doi:10.1007/s13369-020-04946-0
Qiao, S., Du, Z., and Yang, R. (2014). Design and synthesis of novel carbazole–spacer–carbazole type conjugated microporous networks for gas storage and separation. J. Mater. Chem. A 2 (6), 1877–1885. doi:10.1039/c3ta14017b
Ravi, S., Choi, Y., and Bae, Y.-S. (2023). Melamine-functionalized aromatic carbonyl-based polymer with high surface area for efficient CO2 capture. Sep. Purif. Technol. 317, 123828. doi:10.1016/j.seppur.2023.123828
Rizzuto, C., Caravella, A., Brunetti, A., Park, C. H., Lee, Y. M., Drioli, E., et al. (2017). Sorption and diffusion of CO2/N2 in gas mixture in thermally-rearranged polymeric membranes: A molecular investigation. J. Membr. Sci. 528, 135–146. doi:10.1016/j.memsci.2017.01.025
Rong, M., Yang, L., Yang, C., Yu, J., and Liu, H. (2021). Tetraphenyladamantane-based microporous polyaminals for efficient adsorption of CO2, H2 and organic vapors. Microporous Mesoporous Mater. 323, 111206. doi:10.1016/j.micromeso.2021.111206
Rufford, T. E., Smart, S., Watson, G. C. Y., Graham, B. F., Boxall, J., Diniz da Costa, J. C., et al. (2012). The removal of CO2 and N2 from natural gas: A review of conventional and emerging process technologies. J. Petroleum Sci. Eng. 94-95, 123–154. doi:10.1016/j.petrol.2012.06.016
Sadak, A. E. (2021). A comparative gas sorption study of dicarbazole-derived microporous hyper-crosslinked polymers. Microporous Mesoporous Mater. 311, 110727. doi:10.1016/j.micromeso.2020.110727
Song, W., Yao, J., Ma, J., Li, A., Li, Y., Sun, H., et al. (2018). Grand canonical Monte Carlo simulations of pore structure influence on methane adsorption in micro-porous carbons with applications to coal and shale systems. Fuel 215, 196–203. doi:10.1016/j.fuel.2017.11.016
Song, K. S., Fritz, P. W., and Coskun, A. (2022). Porous organic polymers for CO2 capture, separation and conversion. Chem. Soc. Rev. 51 (23), 9831–9852. doi:10.1039/d2cs00727d
Sun, H., Jin, Z., Yang, C., Akkermans, R. L., Robertson, S. H., Spenley, N. A., et al. (2016). Compass II: Extended coverage for polymer and drug-like molecule databases. J. Mol. Model 22 (2), 47. doi:10.1007/s00894-016-2909-0
Taylor, A. A., Tsuji, J. S., Garry, M. R., McArdle, M. E., Goodfellow, W. L., Adams, W. J., et al. (2020). Critical review of exposure and effects: Implications for setting regulatory health criteria for ingested copper. Environ. Manag. 65 (1), 131–159. doi:10.1007/s00267-019-01234-y
Tian, Q., Yuan, Y. C., Rong, M. Z., and Zhang, M. Q. (2009). A thermally remendable epoxy resin. J. Mater. Chem. 19 (9), 1289–1296. doi:10.1039/b811938d
Wang, J., Wei Yang, J. G., Yi, G., and Zhang, Y. (2015). Phosphonium salt incorporated hypercrosslinked porous polymers for CO2 capture and conversion. Chem. Commun. 51 (86), 15708–15711. doi:10.1039/c5cc06295k
Wei, Y., Jang, G.-W., Hsueh, K. F., Scherr, E. M., MacDiarmid, A. G., and Epstein, A. J. (1992). Thermal transitions and mechanical properties of films of chemically prepared polyaniline. Polymer 33 (2), 314–322. doi:10.1016/0032-3861(92)90988-9
Wu, Z., Li, C., Wei, Z., Ying, P., and Xin, Q. (2002). FT-IR spectroscopic studies of thiophene adsorption and reactions on Mo2N/γ-Al2O3 catalysts. J. Phys. Chem. B 106 (5), 979–987. doi:10.1021/jp011577l
Yan, J., Zhang, B., and Wang, Z. (2016). Monodispersed ultramicroporous semi-cycloaliphatic polyimides for the highly efficient adsorption of CO2, H2 and organic vapors. Polym. Chem. 7 (47), 7295–7303. doi:10.1039/c6py01734g
Yao, Q.-X., Pan, L., Jin, X.-H., Li, J., Ju, Z.-F., and Zhang, J. (2009). Bipyridinium array-type porous polymer displaying hydrogen storage, charge-transfer-type guest inclusion, and tunable magnetic properties. Chem. – A Eur. J. 15 (44), 11890–11897. doi:10.1002/chem.200901707
Zakeri, B., Paulavets, K., Barreto-Gomez, L., Echeverri, L. G., Pachauri, S., Boza-Kiss, B., et al. (2022). Pandemic, war, and global energy transitions. Energies 15 (17), 6114. doi:10.3390/en15176114
Zhang, J., Wang, J., Zhang, C., Li, Z., Lu, B., and Zhu, J. (2021). Molecular simulation of C2H4/CO2/N2/O2 adsorption characteristics in lignite and anthracite. AIP Adv. 11 (8), 085205. doi:10.1063/5.0057456
Zhao, H., Luo, X., Zhang, H., Sun, N., Wei, W., and Sun, Y. (2018). Carbon-based adsorbents for post-combustion capture: A review. Greenh. Gases Sci. Technol. 8 (1), 11–36. doi:10.1002/ghg.1758
Keywords: 3D porous polymers, global warming, flue gas purification, CO2 capture, H2 storage
Citation: Al-Bukhari MS, Abdulazeez I, Abdelnaby MM, Aljundi IH and Al Hamouz OCS (2023) 3D porous polymers for selective removal of CO2 and H2 storage: experimental and computational studies. Front. Chem. 11:1265324. doi: 10.3389/fchem.2023.1265324
Received: 22 July 2023; Accepted: 24 August 2023;
Published: 07 September 2023.
Edited by:
Basem Moosa, King Abdullah University of Science and Technology, Saudi ArabiaReviewed by:
Himanshu Aggarwal, Birla Institute of Technology and Science, IndiaStefan Chisca, King Abdullah University of Science and Technology, Saudi Arabia
Copyright © 2023 Al-Bukhari, Abdulazeez, Abdelnaby, Aljundi and Al Hamouz. This is an open-access article distributed under the terms of the Creative Commons Attribution License (CC BY). The use, distribution or reproduction in other forums is permitted, provided the original author(s) and the copyright owner(s) are credited and that the original publication in this journal is cited, in accordance with accepted academic practice. No use, distribution or reproduction is permitted which does not comply with these terms.
*Correspondence: Othman Charles S. Al Hamouz, othmanc@kfupm.edu.sa