- 1Institut de Ciència de Materials de Barcelona (ICMAB-CSIC), Barcelona, Spain
- 2ICN2 – Institut Català de Nanociència i Nanotecnologia (CSIC-ICN), Barcelona, Spain
In the past decade, the rich physics exhibited by solid interfaces combining octahedral framework structures of transition-metal oxides has fascinated the materials science community. However, their behavior still eludes the current understanding of classical semiconductor and metal epitaxy. The reason for that is rooted in the surprising versatility of linked coordination units to adapt to a dissimilar substrate and the strong sensitivity of strongly correlated electron oxides to external perturbations. The confluence of atomic control in oxide thin film epitaxy, state of the art high spatial resolution characterization techniques, and electronic-structure computations, has allowed in recent years to obtain first insights on the microscopic mechanisms governing the epitaxy of these complex materials.
Introduction
Interfaces bridging complex metal oxides have emerged in recent years as a new paradigm of epitaxy owing to their extraordinary potential to exhibit unanticipated and conceptually challenging states such as highly conducting electron gases between two insulating materials (Ohtomo and Hwang, 2004), interfacial superconductivity (Reyren et al., 2007; Gozar et al., 2008), or polarization-dependent spin transfer (Garcia et al., 2010). This fascinating behavior stems from the delicate balance existing among strongly coupled lattice, charge, spin, and orbital degrees of freedom, which in turn manifests a strong sensitivity to external perturbations (Hwang et al., 2012), such as the biaxial stress induced by a mismatched substrate, polar discontinuities, and even very subtle effects like slight dissimilarities in the orientation of the coordination units. These compounds commonly exhibit an ABO3 perovskite-type crystal structure characterized by a framework of corner sharing BO6 oxygen octahedra hosting the B cation that can be derived from a common undistorted cubic aristotype through the tilting of essentially rigid BO6 units (Glazer, 1972; Howard and Stokes, 1998). As illustrated in Figure 1A, considering that the coordination units are rigid but free to rotate about their shared corners, one obtains an articulated framework that may exhibit auxetic behavior (Alderson and Evans, 2002). In this idealized case, the misfit strain directly couples with octahedral tilts, which in turn control the chemical bonding and the physical properties through changes in the overlap between the B-site 3d and oxygen 2p orbitals (Tokura and Nagaosa, 2000). Thus, controlling the topology of the octahedral framework through misfit strain appears as an obvious strategy to control the stability of electronic and magnetic states. In practice, however, the deformation of the framework appears to be governed by the relative rigidity between the B–O–B angles bridging adjacent octahedra and the B–O bonds. The latter, in turn, can be distorted either at the expense of elastic or electronic energies depending on the electronic structure of the transition metal. As a consequence, a deterministic manipulation of interfacial structure and misfit strain in these complex materials demands simultaneous control on electronic (spin, charge, orbital) and lattice (elastic, octahedral tilting) degrees of freedom. Here, we review these mechanisms and highlight their potential in the design of self-organized oxide nanostructures with enhanced functionalities.
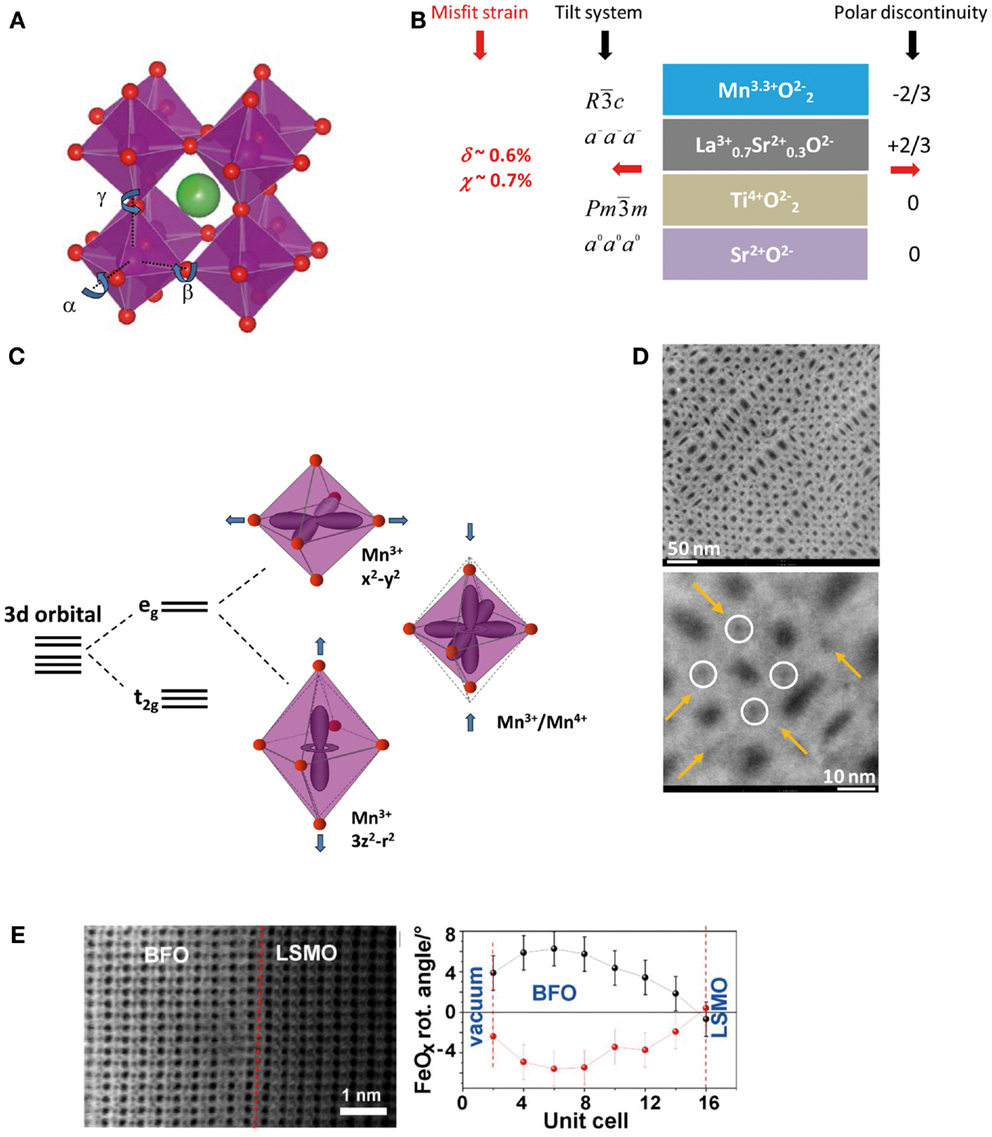
Figure 1. (A) Pseudocubic (distorted) unit cell of an ABO3 perovskite. The aristotype structure (space group Pm−3m) consists of a three-dimensional framework of untilted (α = β = γ = 0) regular BO6 octahedra (O atoms are red circles) within the cavities of which lie the A cations (large green circles). Group-theoretical analysis yields 15 tilt systems (Howard and Stokes, 1998), which are commonly noted following the Glazer notation by the manner in which adjacent octahedra rotate one relative to each other along a particular axis of the undistorted cubic unit cell (Glazer, 1972); for instance, a−(+) indicates out-of-phase (in-phase) tilts and a0 indicates no tilt. Thus the Pm-3m space group corresponds to the a0a0a0 tilt system, while α≠β≠γ≠0° (a−b−c−) corresponds to the triclinic space group P−1. (B) Different sources of dissimilarity between La0.7Sr0.3MnO3 and SrTiO3. The rhombohedral structure of La0.7Sr0.3MnO3 is forced to match the cubic SrTiO3 substrate. The film is submitted to a tensile misfit strain besides a shear strain and a discontinuity between the a−a−a− and a0a0a0 tilt systems. Moreover, as indicated in the right column, the (002) planes in La0.7Sr0.3MnO3 have alternating net charges, while the (002) planes in SrTiO3 are neutral, resulting in a polar discontinuity at the interface. (C) The six O2− anions surrounding the Mn3+/4+ cations in La0.7Sr0.3MnO3 create an octahedral crystal-field potential that partly lifts the degeneracy of the 3d one-electron orbital levels. Orbitals pointing to the oxygen ions, eg, 3z2 − r2 and x2 − y2, are higher in energy than those pointing between them, t2g: xy, yz, zx (not shown). According to recent findings (Pesquera et al., 2012), a tensile misfit strain would favor the preferential occupation of in-plane x2 − y2 orbitals, while a compressive misfit strain would favor the occupation of the out-of-plane 3z2 − r2 ones. Near the polar La0.7Sr0.3MnO3/SrTiO3 interface, the Mn3+/Mn4+ ratio is high and the strong localization of the eg electron gives rise to a measurable deformation of the MnO6 octahedra that contributes to the relaxation of the misfit strain. As the Mn3+/Mn4+ ratio is decreased away from the interface, the delocalized eg electron does not give rise to any measurable distortion of the coordination environment and the octahedra submitted to a misfit strain deform elastically (Sandiumenge et al., 2013). (D) Planar view annular dark field images of the misfit dislocation network in a 7.1 nm thick La0.7Sr0.3MnO3 film grown on a LaAlO3 substrate. The images have been obtained under low angle annular dark field (LAADF) conditions in order to emphasize the strain contrast. The images clearly reveal a self-organized strain pattern associated to the misfit dislocation network. The magnified bottom image shows in detail the correlation between the dislocations (yellow arrows indicate the positions of dislocations and circles mark their intersections) and the strain modulation. (E) Bright field scanning transmission electron microscopy image of a BiFeO3/La0.7Sr0.3MnO3/SrTiO3 heterostructure, and evolution of octahedral tilt angles. The two curves correspond to the antiphase tilting of alternate octahedra. (E) Modified from Borisevich et al. (2010a), copyright 2010, American Physical Society.
Interfacial Effects
Interfaces combining octahedral frameworks of transition-metal oxides tend to react against film/substrate dissimilarities in a cooperative way. This is because the various degrees of freedom of the system are associated with similar energies and therefore there is not a unique leading mechanism. To illustrate a common scenario, Figure 1B exemplifies the types of dissimilarities found between the canonic room temperature half-metal ferromagnet La0.7Sr0.3MnO3 (Urushibara et al., 1995) and the commonly used (001) oriented SrTiO3 substrate. In the following subsections, we briefly analyze the consequences of the various dissimilarities on the topology and the electronic structure of the epitaxial framework.
Misfit Strain
Rhombohedral perovskites like La0.7Sr0.3MnO3 (space group R-3c) are characterized by equal antiphase tilts of the BO6 octahedra about the three Cartesian axes, α = β = γ (see Figure 1A), resulting in a net rotation ω about the pseudocubic [111] direction α = β = γ = ω/31/3 (Haun et al., 1989). According to Glazer, 1972, this octahedral tilt pattern is noted as a−a−a−, where the components of the triad describe the octahedral tilts about the unit cell axes of the undistorted cubic aristotype (Pm-3m, a0a0a0). As a result of this tilting, the unit cell interaxial angles, αc, are no longer 90° (Megaw and Darlington, 1975), inducing a misfit shear strain χ = tan(αc − 90°) relative to the underlying (001) plane of the cubic substrate. Note that since the substrate does not impose constrains on the inclination of the out-of-plane pseudocubic axis, the initial −3m rhombohedral point symmetry is reduced to a 2/m monoclinic one (Daumont et al., 2010). Therefore, the total strain energy of the rhombohedral film, ET, splits into two contributing elastic energies, a shear and a normal one, ET = E(χ) + E(δ). In the absence of further perturbations at the interface, and assuming that the lattice distorts elastically (i.e., that strain couples with the B–O bonds, with no octahedral rotations), dislocation models (Matthews, 1975) predict a relaxation of E(δ) at critical thickness of ~10 nm (Abellán et al., 2009), in strong contrast with the observation of fully strained in-plane lattice parameters up to 475 nm (Sandiumenge et al., 2013). Conversely, E(χ) is expected to be relieved from the initial growth stages by twinning on the (100) and (010) planes perpendicular to the interface (Farag et al., 2005), again in conflict with experimental observations pointing the occurrence of a shear transition at a critical thickness of about 2.5 nm (Sandiumenge et al., 2013; Santiso et al., 2013). Such discrepancies constitute in fact a dramatic manifestation of the ability of octahedral frameworks to propagate interfacial perturbations over length scales exceeding a few 100 nm at a lower energy cost than that associated with the formation of the misfit dislocation network needed to plastically relax an equivalent elastic strain energy.
Misfit strains have important consequences on the electronic structure of the films. As shown in Figure 1C, the octahedral coordination of the Mn-sites splits the 3d orbitals into a degenerate t2g triplet and a degenerate eg doublet with xy/yz/xz and x2 − y2/3z2 − r2 symmetries, respectively. Misfit strains induce further lowering of the symmetry that also unfolds the eg doublet. This is commonly achieved by half-filling of the eg orbitals, in which case the strong electron-phonon coupling induces a Jahn–Teller distortion of the coordination environment that breaks the degeneracy of the x2 − y2 and 3z2 − r2 orbitals, thus modifying their electron occupancy and eventually leading to orbital reconstructions. In the La1-xSrxMnO3 solid solution, the electron occupancy of the eg doublet depends on the strength of the Jahn–Teller distortion, which in turn is determined by the hole doping level, x (Tokura and Nagaosa, 2000). In epitaxial thin films, however, similarly to the Jahn–Teller distortion, misfit strains have the ability to break the x2 − y2/3z2 − r2 orbital degeneracy (Fang et al., 2000) as recently confirmed by X-ray linear dichroism investigations (Huijben et al., 2008; Tebano et al., 2008; Pesquera et al., 2012). A thorough investigation taking into account the attenuation of the signal with the depth in the sample, suggests that the observed X-ray linear dichroism is rather a consequence of the superposition of interfacial and free surface effects (Pesquera et al., 2012). As shown in Figure 1C, those results suggest that a tensile strain would favor the occupancy of the in-plane x2 − y2 orbitals, while a compressive strain would induce the occupancy of the out-of-plane 3z2 − r2 ones, as expected from electrostatic arguments. On the other hand, the Mn3+ cations located at the free surface appear to exhibit a preferred 3z2 − r2 occupancy regardless of the sign of the interfacial biaxial strain. According to theoretical work (Calderon et al., 1999), this scenario is promoted by the absence of the apical oxygen in the coordination environment of surface cations and the resulting reduction of the repulsive electron–electron interaction between the manganese 3d (3z2 − r2) and oxygen 2p (z) orbitals.
Despite the surprising adaptability of the octahedral framework, there are situations where plastic relaxation is energetically favored. As an example, La0.7Sr0.3MnO3 films grown on LaAlO3 substrates under a compressive strain of −2.3% are relaxed by misfit dislocations at a critical thickness of about 3 nm. This indicates that there is a limited range of rigid octahedral tilts within which the film can deform to fit the substrate. In this case, the value of the out-of-plane lattice parameter prior to the onset of plastic relaxation, 3.98(1) Å, is consistent with the value derived from the Poisson’s ratio ν = 0.33, 3.975 Å, indicating that the film behaves elastically. Note that on a rigid octahedron basis, see Figure 1A, a shrinking of the in-plane lattice parameters can be either accomplished by increasing the octahedral tilt γ about the c-axis, or by increasing the octahedral tilts simultaneously about the in-plane a and b axes. While the first operation would leave the length of the c-axis unaltered, the latter would even shrink it resulting in a strong contraction of the unit cell volume. Figure 1D shows the strain pattern associated with the misfit dislocation structure of a 7.1 nm thick film projected on the interface plane, using annular dark field scanning transmission electron microscopy at low beam convergence angles (Fitting et al., 2006). The density of the pattern can be manipulated through the thickness of the film. Thus, misfit dislocations in such compounds provide a means for the self-organization and spatial modulation of strain patterns in a strongly susceptible functional matrix, expanding the possibilities of interfacial engineering beyond homogeneous manipulations of the octahedral pattern. Moreover, dislocations in complex oxides are known to exhibit distinct electrical properties compared to the host material (Szot et al., 2006). Although the control of their density and distribution has been extensively studied in other functional oxides in bulk form (Sandiumenge et al., 2000), the spontaneous formation of regular patterns at interfaces in heteroepitaxial systems offers unique opportunities for miniaturization.
Polar Discontinuities
Besides misfit strains, polar discontinuities are a common driving force for interfacial charge and orbital reconstructions. They appear as a result of the fact that individual atomic layers AO and BO2 in ABO3 perovskites can be electrically charged, as is the case of La0.7Sr0.3MnO3 (see Figure 1B). The net charge per area unit is +2/3e and −2/3e in La0.7Sr0.3MnO3, while it is null in SrTiO3 (e is the electron charge). Polar discontinuities lead to a diverging electrostatic potential which in the absence of any reconstruction results in the so-called polar catastrophe (Nakagawa et al., 2006). Since La0.7Sr0.3MnO3 exhibits metallic behavior, mobile charges can screen the diverging potential (Dagotto, 2009), resulting in a compensating charge transfer of 1/3e. This would lead to a shift of the Mn valency at the interface over a screening length of a few nanometers toward 3+, as confirmed by electron energy loss (Riedl et al., 2009), X-ray absorption (Lee et al., 2010), and x-ray photoemission (Sandiumenge et al., 2013) spectroscopies. According to the bulk La1−xSrxMnO3 phase diagram (Dabrowski et al., 1999), the transfer of charge toward the interface results in the formation of a non-ferromagnetic and insulating wetting layer called ‘‘dead-layer.” As films thicken beyond ~2.5 nm, the number of eg electrons decreases and become delocalized with strong consequences on the deformation behavior of the films; the electronic energy gained by removing the eg orbital degeneracy becomes progressively exceeded by the elastic energy opposing a similar expansion of the equatorial Mn–O distances needed to keep the in-plane lattice parameters fully strained (see Figure 1C). This unbalance evolves until a fully elastically strained rhombohedral phase condenses at a thickness of 10–20 nm, similar to the critical thickness for plastic relaxation. Strikingly, above this thickness the elastic energy is released by a composite octahedral tilting mechanism, which allows to maintain fully strained in-plane lattice parameters up to at least 475 nm (Sandiumenge et al., 2013). This complex behavior clearly reflects the competing nature of the different degrees of freedom involved in the relaxation mechanism of such materials.
Thanks to the extraordinary progress achieved in the atomic control of interfaces in oxide heterostructures, the formation of the dead-layer can be avoided by precise modifications of the interfacial architecture (Boschker et al., 2012), or even polar discontinuities can be exploited to intentionally induce hole doping avoiding disorder effects typically associated with chemical doping (Dagotto, 2009).
Octahedral Tilt Discontinuities
A more subtle dissimilarity is that between the octahedral tilt systems of the film and the substrate. The concept of interfacial octahedral coupling resides in the idea that the substrate tends to transmit its octahedral pattern to the film. This concept has motivated an intense research on its use for the engineering of novel tilt patterns with specific functionalities (Rondinelli and Spaldin, 2011; Rondinelli et al., 2012; Moon et al., 2014). Excitingly, recent advances in electron microscopy have provided direct access to oxygen positions (Jia et al., 2009; Borisevich et al., 2010a), and though direct experimental evidences are still very scarce, indeed they support the idea of octahedral coupling across the interfaces.
As an example, Figure 1E shows the variation of octahedral tilt angles in a BiFeO3 film grown on a 5 nm La0.7Sr0.3MnO3 buffer layer fully elastically strained on top of a SrTiO3 substrate, as determined by bright field scanning transmission electron microscopy imaging (Borisevich et al., 2010a). As can be seen in the right panel, the in-plane octahedral tilts of the La0.7Sr0.3MnO3 buffer are suppressed by the influence of the cubic SrTiO3 substrate, and this pattern is transmitted to the first unit cells of the bismuth ferrite film. Tilt angles in the BiFeO3 layer almost recover their bulk values at ~10 u.c. ahead of the interface. Similarly, the propagation of the octahedral tilt pattern of a rhombohedral LaAlO3 substrate into an epitaxial cubic SrTiO3 film has been directly determined using the negative spherical aberration imaging technique (Jia et al., 2009). These experiments provide direct evidence for the coupling of the antiferrodistortive order parameter (octahedral tilting) at interfaces, previously postulated for the SrTiO3/LaTiO3 (Hamann et al., 2006; Okamoto et al., 2006) and SrTiO3/LaAlO3 systems (Willmott et al., 2007) systems. Notably, a similar octahedral tilt suppression has also been determined inside the twin walls of BiFeO3 (Borisevich et al., 2010b), thus pointing an increased orbital overlap and thus bigger bandwidth as responsible for their enhanced conductivity (Catalan and Scott, 2009).
Self-Organization of Nanostructures
Island growth in heteroepitaxial systems is driven by high interface energy and high film surface energy. In octahedral framework systems, the flexibility of the lattice to accommodate large interfacial dissimilarities favors the wetting of the substrate hindering the formation of islands. However, interesting results have been obtained by the combination of different structural families using chemical solution deposition, like, for instance, perovskite/rock-salt (La0.7Sr0.3MnO3/MgO) (Abellán et al., 2012), fluorite/perovskite (CeO2/LaAlO3) (Gibert et al., 2010), and perovskite/fluorite (La0.7Sr0.3MnO3/YSZ) (Zabaleta et al., 2013). In all cases, interfaces appear highly dislocated and therefore affected by strain fields that may extend over a few unit cells into the island with negative effects on their functionalities, such as the induction of polarization instabilities in ferroelectric materials (Chu et al., 2004). Interestingly, dislocation free PbTiO3 nanoislands exhibiting spontaneous polarization have been grown under a small tensile misfit strain of 0.15% on conducting Nb-doped SrTiO3, using block copolymer micelles followed by epitaxial crystallization (Kim et al., 2010). The strong sensitivity of the growth mode to interfacial subtleties was demonstrated in the pulsed laser deposited Y-stabilized ZrO2/SrTiO3 (fluorite/perovskite) system, in which the fluorite film form continuous films or islands depending on the atomic termination plane of the SrTiO3 substrate (Cavallaro et al., 2011).
On the other hand, it has been recently reported that the extent of interfacial perturbations along the growth direction can be controlled through the growth kinetics, inducing morphological instabilities, which ultimately lead to self-organized surfaces. In particular, robust patterns of self-organized nanopits have been obtained on the surface of La0.7Sr0.3MnO3 films grown on SrTiO3 substrates (see Figure 2A) (Konstantinovic et al., 2013). The nanopits are aligned with the steps of the underlying substrate and result from the morphological evolution of continuous grooves created during the first growth stages to relax the strain concentration at step edges. In contrast with the mechanism operating in semiconductors, such as InGaN-based heterostructures, where nanopits form at the apex of threading dislocations (de Sousa Pereira et al., 2008) or associated to other strain sources embedded in the volume of the film (Liliental-Weber et al., 1997), here the formation of the nanopits does not require the presence of other defects, which could deteriorate the properties of the film. Moreover, the nanostructured surface has been successfully employed as a template for the integration of epitaxial Au nanocrystals exhibiting a perfect octahedral shape (Figures 2B–D) and excellent metallic-like behavior across the Au/La0.7Sr0.3MnO3 interface (Figure 2E). Interestingly, such films exhibiting a modified distortion not found in conventional films, exhibit enhanced colossal magnetoresistance response (Pomar et al., 2014), thus further exemplifying the wide range of applications that can be accessed through the control of octahedral frameworks.
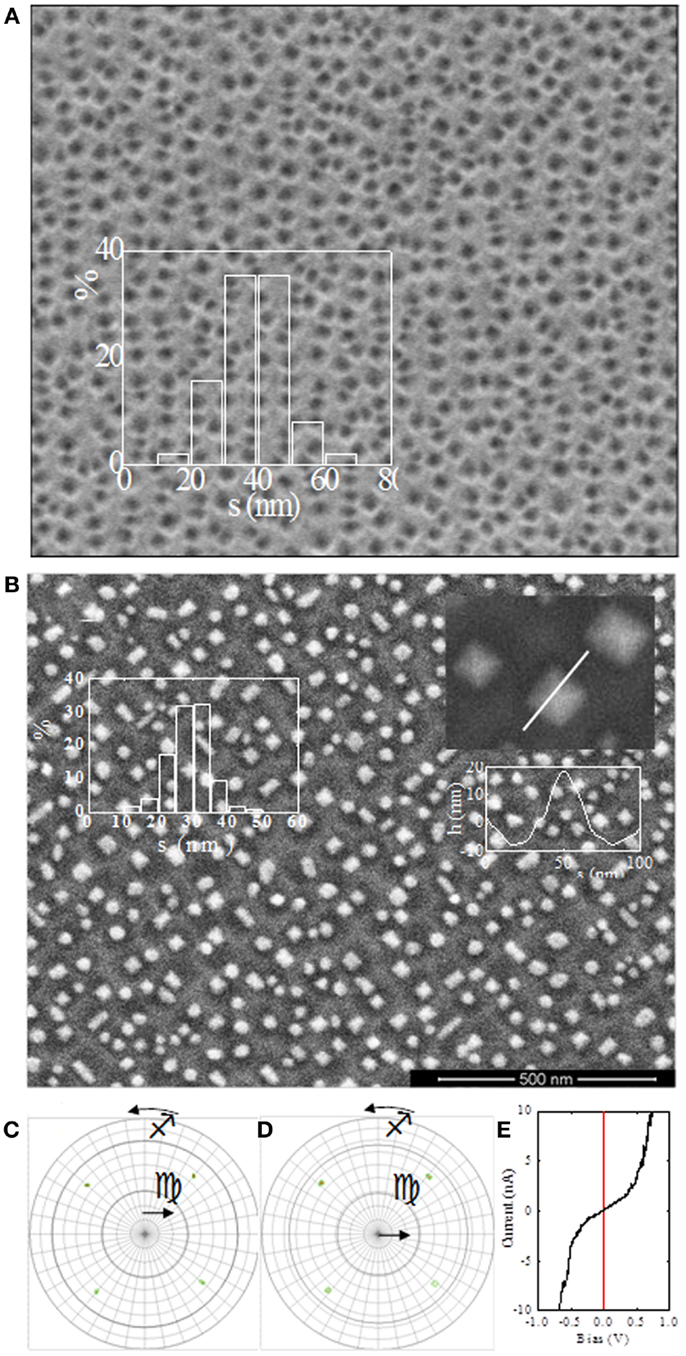
Figure 2. (A) Scanning electron micrograph showing self-organized nanopits on the surface of a La0.7Sr0.3MnO3 film. Inset shows the nanopit size distribution histogram. (B) Scanning electron micrograph of the Au nanocrystals grown inside the nanopits. Upper left inset shows the size distribution histogram of the Au nanocrystals. Upper right inset: upper panel is a magnified image of an Au nanocrystal exhibiting octahedral shape; lower panel shows a typical atomic force microscopy topographic profile of one Au nanocrystal. (C,D) (111)-X-ray diffraction pole-figures corresponding to the La0.7Sr0.3MnO3 film and the Au nanocrystals, demonstrating their cube-on-cube epitaxial relationship. (E) I–V curves obtained by using atomic force microscopy in current sensing mode when the conducting tip is placed directly on top of the bare La0.7Sr0.3MnO3 surface (black curve) or on top of an Au nanocrystal (red curve). The black curve exhibits the typical features of tunneling conduction, resulting from the deterioration of the La0.7Sr0.3MnO3 surface, while the red curve exhibits metallic-like behavior, indicating that the Au nanocrystals protect the La0.7Sr0.3MnO3 surface against degradation due to ambient conditions (from Konstantinovic et al., 2013).
Future Prospects
Last decade has witnessed the emergence of unprecedented phenomena at transition-metal oxide interfaces that could not have been anticipated from the knowledge built upon conventional semiconductor epitaxy. This behavior stems from the confluence of strongly competing charge, spin, orbital and lattice degrees of freedom, the coupling between them, and a surprisingly versatile mechanical response of octahedral frameworks to external perturbations. A radically new concept that holds strong promise for the predictive manipulation of the framework topology is the translation of the octahedral pattern of the substrate to the film (Rondinelli et al., 2012). Nevertheless, detailed knowledge on the boundaries between elastic, electronic, octahedral tilting, and plastic deformation regimes (Sandiumenge et al., 2013), as well as on the ability to control polar discontinuities (Dagotto, 2009; Boschker et al., 2012), is still needed to avoid interactions that could interfere with octahedral couplings. Besides electronic bandwidth-dependent properties, preferential orbital occupancies, particularly at free surfaces, open the door to catalytic applications (Suntivich et al., 2011a,b). An almost unexplored field is the manipulation of the octahedral framework to induce growth instabilities ultimately leading to self-organized surfaces (Konstantinovic et al., 2013), interfaces, or modulated bulk structures. On the other hand, guided organization using block copolymer micelles has been successfully used to limit the lateral dimensions of ferroelectric islands under almost null misfit strain (Kim et al., 2010). There is no doubt that octahedral frameworks still demand new challenging conceptual approaches toward nanofabrication and interface manipulation, but are becoming the working horse of many future applications.
Conflict of Interest Statement
The authors declare that the research was conducted in the absence of any commercial or financial relationships that could be construed as a potential conflict of interest.
Acknowledgments
This research was supported by Spanish MEC (MAT2011-29081 and MAT2012-33207), CONSOLIDER (CSD2007-00041 and CSD2008-00023), and FEDER program.
References
Abellán, P., Sandiumenge, F., Moreno, C., Casanove, M.-J., Puig, T., and Obradors, X. (2009). Strain relaxation of self-nanostructured solution derived La0.7Sr0.3MnO3 films. MRS Proc. 1174, doi: 10.1557/PROC-1174-V04-12
Abellán, P., Zabaleta, J., Santiso, J., Casanove, M.-J., Dix, N., Aguiar, J., et al. (2012). Interface structure governed by plastic and structural dissimilarity in perovskite La0.7Sr0.3MnO3 nanodots on rock-salt MgO substrates. Appl. Phys. Lett. 100, 083104. doi:10.1063/1.3687692
Alderson, A., and Evans, K. (2002). Molecular origin of auxetic behavior in tetrahedral framework silicates. Phys. Rev. Lett. 89, 225503. doi:10.1103/PhysRevLett.89.225503
Borisevich, A. Y., Chang, H. J., Huijben, M., Oxley, M. P., Okamoto, S., Niranjan, M. K., et al. (2010a). Suppression of octahedral tilts and associated changes in electronic properties at epitaxial oxide heterostructure interfaces. Phys. Rev. Lett. 105, 087204. doi:10.1103/PhysRevLett.105.087204
Borisevich, A. Y., Ovchinnikov, O. S., Chang, H. J., Oxley, M. P., Yu, P., Seidel, J., et al. (2010b). Mapping octahedral tilts and polarization across a domain wall in BiFeO3 from Z-contrast scanning transmission electron microscopy image atomic column shape analysis. ACS Nano 4, 6071. doi:10.1021/nn1011539
Boschker, H., Verbeeck, J., Egoavil, R., Bals, S., van Tendeloo, G., Huijben, M., et al. (2012). Preventing the reconstruction of the polar discontinuity at oxide heterointerfaces. Adv. Funct. Mater. 22, 2235–2240. doi:10.1002/adfm.201102763
Calderon, M. J., Brey, L., and Guinea, F. (1999). Surface electronic structure and magnetic properties of doped manganites. Phys. Rev. B 60, 6698–6704. doi:10.1103/PhysRevB.60.6698
Catalan, G., and Scott, J. F. (2009). Physics and applications of bismuth ferrite. Adv. Mater. 21, 2463–2485. doi:10.1002/adma.200802849
Cavallaro, A., Ballesteros, B., Bachelet, R., and Santiso, J. (2011). Heteroepitaxial orientation control of YSZ films by selective growth on SrO-, TiO2-terminated SrTiO3 crystal surfaces. CrystEngComm. 13, 1625–1631. doi:10.1039/C0CE00606H
Chu, M.-W., Szafraniak, I., Scholz, R., Harnagea, C., Hesse, D., Alexe, M., et al. (2004). Impact of misfit dislocations on the polarization instability of epitaxial nanostructure ferroelectric perovskites. Nat. Mater. 3, 87–90. doi:10.1038/nmat1057
Dabrowski, B., Xiong, X., Bukowski, Z., Dybzinski, R., Klamut, P. W., Siewenie, J. E., et al. (1999). Structure-properties phase diagram for La1-xSrxMnO3 (0.1 ≤x ≤0.2). Phys. Rev. B 60, 7006. doi:10.1103/PhysRevB.60.7006
Dagotto, E. (2009). Playing with the geometry of oxide heterostructures. Physics 2, 12–13. doi:10.1103/Physics.2.12
Daumont, C. J. M., Farokhipoor, S., Ferri, A., Wojdel, J. C., ĺñiguez, J., Kooi, B. J., et al. (2010). Tuning the atomic and domain structure of epitaxial films of multiferroic BiFeO3. Phys. Rev. B 81, 144115. doi:10.1103/PhysRevB.81.144115
de Sousa Pereira, S. M., Martins, M. A., Trindade, T., Watson, I. M., Zhu, D., and Humphreys, C. J. (2008). Controlled integration of nanocrystals in inverted hexagonal nano-pits at the surface of light-emitting heterostructures. Adv. Mater. 20, 1038–1043. doi:10.1002/adma.200701739
Fang, Z., Solovyev, I., and Terakura, K. (2000). Phase diagram of tetragonal manganites. Phys. Rev. Lett. 84, 3169–3172. doi:10.1103/PhysRevLett.84.3169
Farag, N., Bobeth, M., Pompe, W., Romanov, A. E., and Speck, J. S. (2005). Modeling of twinning in epitaxial (001)-oriented La0.67Sr0.33MnO3 thin films. J. Appl. Phys. 97, 113516. doi:10.1063/1.1914950
Fitting, L., Thiel, S., Schmehl, A., Mannhart, J., and Muller, D. A. (2006). Subtleties in ADF imaging and spatially resolved EELS: a case study of low-angle grain boundaries in SrTiO3. Ultramicroscopy 106, 1053–1061. doi:10.1016/j.ultramic.2006.04.019
Garcia, V., Bibes, M., Bocher, L., Valencia, S., Kronast, F., Crassous, A., et al. (2010). Ferroelectric control of spin polarization. Science 327, 1106–1110. doi:10.1126/science.1184028
Gibert, M., Abellán, P., Benedetti, A., Puig, T., Sandiumenge, F., García, A., et al. (2010). Self-organized Ce1-xGdxO2-y nanowire networks with very fast coarsening driven by attractive elastic interactions. Small 6, 2716–2724. doi:10.1002/smll.201001237
Glazer, A. M. (1972). The classification of tilted octahedra in perovskites. Acta Cryst. B 28, 3384–3392. doi:10.1107/S0567740872007976
Gozar, A., Logvenov, G., Fitting Kourkoutis, L., Bollinger, A. T., Giannuzzi, L. A., Muller, D. A., et al. (2008). High-temperature interface superconductivity between metallic and insulating copper oxides. Nature 455, 782–785. doi:10.1038/nature07293
Hamann, D. R., Muller, D. A., and Hwang, H. Y. (2006). Lattice-polarization effects on electron-gas charge densities in ionic superlattices. Phys. Rev. B 73, 195403. doi:10.1103/PhysRevB.73.195403
Haun, M. J., Furman, E., Halemane, T. R., and Cross, L. E. (1989). Thermodynamic theory of the lead zirconate-titanate solid solution system, part IV: tilting of the oxygen octahedra. Ferroelectrics 99, 55–62. doi:10.1080/00150198908221436
Howard, J., and Stokes, H. T. (1998). Group-theoretical analysis of octahedral tilting in perovskites. Acta Cryst. B 54, 782–789. doi:10.1107/S0108768198004200
Huijben, M., Martin, L. W., Chu, Y. H., Holcomb, M. B., Yu, P., Rjinders, G., et al. (2008). Critical thickness and orbital ordering in ultrathin La0.7Sr0.3MnO3. Phys. Rev. B 78, 094413. doi:10.1103/PhysRevB.78.094413
Hwang, H. Y., Iwasa, Y., Kawasaki, M., Keimer, B., Nagaosa, N., and Tokura, Y. (2012). Emergent phenomena at oxide interfaces. Nat. Mater. 11, 103–113. doi:10.1038/nmat3223
Jia, C. L., Mi, S. B., Faley, M., Poppe, U., Schubert, J., and Urban, K. (2009). Oxygen octahedron reconstruction in the SrTiO3/LaAlO3 heterointerfaces investigated using aberration-corrected ultrahigh-resolution transmission electron microscopy. Phys. Rev. B 79, 081405. doi:10.1103/PhysRevB.79.081405
Kim, Y., Han, H., Kim, Y., Lee, W., Alexe, M., Baik, S., et al. (2010). Untrahigh density array of epitaxial nanoislands on conducting substrates. Nano Lett. 10, 2141–2146. doi:10.1021/nl100819d
Konstantinovic, Z., Sandiumenge, F., Santiso, J., Balcells, L. L., and Martínez, B. (2013). Self-assembled pit arrays as templates for the integration of Au nanocrystals in oxide surfaces. Nanoscale 5, 1001–1008. doi:10.1039/c2nr33181k
Lee, J.-S., Arena, D. A., Yu, P., Nelson, C. S., Fan, R., Kinane, C. J., et al. (2010). Hidden magnetic configuration in epitaxial La1-xSrxMnO3 films. Phys. Rev. Lett. 105, 257204. doi:10.1103/PhysRevLett.105.257204
Liliental-Weber, Z., Chen, Y., Ruvimov, S., and Washburn, J. (1997). Formation mechanism of nanotubes in GaN. Phys. Rev. Lett. 79, 2835–2838. doi:10.1103/PhysRevLett.79.2835
Matthews, J. W. (1975). Defects associated with the accommodation of misfit between crystals. J. Vac. Sci. Technol. 12, 126–133. doi:10.1116/1.568741
Megaw, H. D., and Darlington, C. N. W. (1975). Geometrical and Structural relations in the rhombohedral perovskites. Acta Cryst. A 31, 161–173. doi:10.1107/S0567739475000332
Moon, E. J., Balachandran, P. V., Kirby, B. J., Keavney, D. J., Sichel-Tissot, R. J., Schlepütz, C. M., et al. (2014). Effect of interfacial octahedral behavior in ultrathin manganite films. Nano Lett. 14, 2509–2514. doi:10.1021/nl500235f
Nakagawa, N., Hwang, H. Y., and Muller, D. A. (2006). Why some interfaces cannot be sharp. Nat. Mater. 5, 204–209. doi:10.1038/nmat1569
Ohtomo, A., and Hwang, H. Y. (2004). A high-mobility electron gas at the LaAlO3/SrTiO3 heterointerface. Nature 427, 423–426. doi:10.1038/nature02308
Okamoto, S., Millis, A. J., and Spaldin, N. (2006). Lattice relaxation in oxide heterostructures: LaTiO3/SrTiO3 superlattices. Phys. Rev. Lett. 97, 056802. doi:10.1103/PhysRevLett.97.056802
Pesquera, D., Herranz, G., Barla, A., Pellegrin, E., Bondino, F., Magnano, E., et al. (2012). Surface symmetry-breaking and strain effects on orbital occupancy in transition metal perovskite epitaxial films. Nat. Commun. 3:1189. doi:10.1038/ncomms2189
Pomar, A., Santiso, J., Sandiumenge, F., Roqueta, J., Bozzo, B., Frontera, C., et al. (2014). Growth kinetics engineered magnetoresistance response in La0.7Sr0.3MnO3 thin films. Appl. Phys. Lett. 104, 152406. doi:10.1063/1.4871984
Reyren, N., Thiel, S., Caviglia, A. D., Fitting Kourkoutis, L., Hammerl, G., Richter, C., et al. (2007). Superconducting interfaces between insulating oxides. Science 317, 1196–1199. doi:10.1126/science.1146006
Riedl, T. H., Gemming, T. H., Dörr, K., Luysberg, M., and Wetzig, K. (2009). Mn valency at La0.7Sr0.3MnO3/SrTiO3(001) thin film interfaces. Microsc. Microanal. 15, 213–221. doi:10.1017/S1431927609090229
Rondinelli, J. M., May, S. J., and Freeland, J. W. (2012). Control of octahedral connectivity in perovskite oxide heterostructures: an emerging route to multifunctional materials discovery. MRS Bull. 37, 261–270. doi:10.1557/mrs.2012.49
Rondinelli, J. M., and Spaldin, N. A. (2011). Structure and properties of functional oxide thin films: insights from electronic-structure calculations. Adv. Mater. 23, 3363–3381. doi:10.1002/adma.201101152
Sandiumenge, F., Puig, T., Rabier, J., Plain, J., and Obradors, X. (2000). Optimization of flux pinning in bulk melt textured 1-2-3 superconductors: bringing dislocations under control. Adv. Mat. 12, 375–381. doi:10.1002/(SICI)1521-4095(200003)12:5<375::AID-ADMA375>3.0.CO;2-9
Sandiumenge, F., Santiso, J., Balcells, L. L., Konstantinovic, Z., Roqueta, J., Pomar, A., et al. (2013). Competing misfit relaxation mechanisms in epitaxial correlated oxides. Phys. Rev. Lett. 110, 107206. doi:10.1103/PhysRevLett.110.107206
Santiso, J., Balcells, L. L., Konstantinovic, Z., Roqueta, J., Ferrer, P., Pomar, A., et al. (2013). Thickness evolution of the twin structure and shear strain in LSMO films. CrystEngComm. 15, 3908–3918. doi:10.1039/c3ce40085a
Suntivich, J., Gasteiger, H. A., Yabuuchi, N., Goodenough, J. B., and Shao-Horn, Y. (2011a). Design principles for oxygen-reduction activity on perovskite oxide catalysts for fuel cells and metal-air batteries. Nat. Chem. 3, 546–550. doi:10.1038/nchem.1069
Suntivich, J., May, K. J., Gasteiger, H. A., Goodenough, J. B., and Shao-Horn, Y. A. (2011b). Perovskite oxide optimized for oxygen evolution catalysis from molecular orbital principles. Science 334, 1383–1385. doi:10.1126/science.1212858
Szot, K., Speier, W., Bihlmayer, G., and Waser, R. (2006). Switching the electrical resistance of individual dislocations in single-crystalline SrTiO3. Nat. Mater. 5, 312–320. doi:10.1038/nmat1614
Tebano, A., Aruta, C., Sanna, S., Medaglia, P. G., Balestrino, G., Sidorenko, A. A., et al. (2008). Evidence of orbital reconstruction at interfaces in ultrahin La0.67Sr0.33MnO3 film. Phys. Rev. Lett. 100, 137401. doi:10.1103/PhysRevLett.100.137401
Tokura, Y., and Nagaosa, N. (2000). Orbital physics in transition-metal oxides. Science 288, 462–468. doi:10.1126/science.288.5465.462
Urushibara, Y., Morimoto, Y., Arima, T., Asamitsu, A., Kido, G., and Tokura, Y. (1995). Insulator-metal transition and giant magnetoresistance in La1-xSrxMnO3. Phys. Rev. B 51, 14103. doi:10.1103/PhysRevB.51.14103
Willmott, P. R., Pauli, S. A., Herger, R., Schlepütz, C. M., Martoccia, D., Patterson, B. D., et al. (2007). Structural basis for the conducting interface between LaAlO3 and SrTiO3. Phys. Rev. Lett. 99, 155502. doi:10.1103/PhysRevLett.99.155502
Keywords: framework structures, strongly correlated electron oxides, perovskite, epitaxy, self-organization, strain, polar discontinuity, functional properties
Citation: Sandiumenge F, Bagués N and Santiso J (2014) Interfaces and nanostructures of functional oxide octahedral framework structures. Front. Mater. 1:13. doi: 10.3389/fmats.2014.00013
Received: 27 June 2014; Accepted: 07 August 2014;
Published online: 21 August 2014.
Edited by:
P. Davide Cozzoli, University of Salento, ItalyCopyright: © 2014 Sandiumenge, Bagués and Santiso. This is an open-access article distributed under the terms of the Creative Commons Attribution License (CC BY). The use, distribution or reproduction in other forums is permitted, provided the original author(s) or licensor are credited and that the original publication in this journal is cited, in accordance with accepted academic practice. No use, distribution or reproduction is permitted which does not comply with these terms.
*Correspondence: Felip Sandiumenge, Institut de Ciència de Materials de Barcelona, (ICMAB-CSIC), Campus de la UAB, Bellaterra, Barcelona 08193, Spain e-mail:ZmVsaXBAaWNtYWIuZXM=