Emergent models for artificial light-harvesting
- 1Cavendish Laboratory, University of Cambridge, Cambridge, UK
- 2Computational Science Laboratory, Microsoft Research, Cambridge, UK
Introduction and Background
Photovoltaic (PV) solar energy conversion is one of the most promising emerging renewable energy technologies and is likely to play an ever-increasing role in the future of electricity generation. The potential of photovoltaics is considered to be much larger than the current energy demand, which is equivalent to ≈13,000 millions tons of oil (BP, 2014). Together with other renewable energy sources, photovoltaics could thus considerably aid the decarbonization of the energy sector in the near future, an objective, which is (should be) seriously sought after to meet the IPCC 2°C target1. Indeed, PV energy conversion is an ever-increasing research field having an impact on other sectors: technological (e.g., dealing with energy storage and distribution issues) and economical (e.g., evaluating the costs of PV devices compared to conventional energy technologies).
The typical working principle of a photovoltaic cell is quite simple, and well understood in terms of doped inorganic semiconductors (Wurfel, 2009). Photovoltaic technology has significantly moved forward since the first silicon (Si) solar cell having an efficiency of ≈4% was invented in 1950s in Bell Laboratories, following the breakthrough of the p–n junction developed by Shockley, Brattain, and Bardeen (Chapin et al., 1954). Since then, research on PV has led to single-junction and multi-junction solar cells with record efficiencies in controlled (laboratory) environments of ≈29% (Green et al., 2014) and 46% (Fraunhofer-ISE, 2014), respectively. However, the efficiency of the most popular technologies in the commercial market – which belong to the so called first generation of solar cells, mainly based on crystalline Si – is in the 10–18% range and around 25% in laboratory. A second generation of cells, mainly aimed at reducing the fabrication costs, is based on thin film architectures, where light is absorbed and charge generated in a solid thin layer of semiconductor (e.g., CdTe, CIGS), with efficiencies restrained to around 22%. Beyond these technologies, a variety of other PV designs has emerged, mainly driven by the progress on materials research and the need of low-cost manufacture devices, organic photovoltaic (OPV) being one of the most interesting solutions. The latest scientific developments in this field have taken various directions (using different concepts, materials, and geometries), all having the potential to result in high-efficiency PV devices. Most current approaches, which have been termed third generation PV [see e.g., Green (2003)], explore schemes beyond the guidelines given by Shockley and Queisser (1961), which are based on the assumption that each photon absorbed above the band gap of the semiconductor photovoltaic material generates a single electron-hole pair. These methods include solar cells exploiting multiple-exciton generation in both inorganic and organic materials, hot carriers collection, up- and down-conversions.
Reviewing the extensive literature about the past and present PV schemes is beyond the scope of this Opinion, many excellent reviews having already succeeded in this (challenging) task. Here, we will illustrate and highlight our point of view on an emergent strategy for PV and artificial light-harvesting in general. This strategy holds onto an interdisciplinary framework encompassing biology and quantum mechanics. Ideas, which have been developed within this nascent trend, are inspired by the recent discovery of quantum effects in biological systems (Mohseni et al., 2014), and aim to model and reproduce the highly efficient reactions often exhibited by these systems using the tools of quantum mechanics and nanotechnology platforms. Novel theoretical proposals, for instance (Scully, 2010; Blankenship et al., 2011; Scully et al., 2011; Creatore et al., 2013; Zhang et al., 2015), have underlined the relevance of effects of quantum coherence to enhance the performance of photocells whose working principle is inspired by the architecture of biological light-harvesting complexes (LHCs).
Emergent Schemes for Artificial Light-Harvesting
Mimicking photosynthesis is perhaps the most intuitive scheme for building photovoltaic devices: as photovoltaic solar cells convert sunlight into electricity, biological LHCs use photosynthesis to convert sunlight into chemical energy necessary for sustaining their life. Biological photosynthesis begins with an ultrafast sequence of photophysical events, known as light reactions, which occur via a diverse range of nanoscaled pigment–protein complexes (PPCs) found in photosynthetic plants, algae, and bacteria (Blankenship, 2008). There reactions proceed through the creation of molecular excited states (excitons) by the absorption of solar photons, then followed by intra- and/or inter-PPC-exciton energy transfer (EET); finally, the excited state energy reaches a reaction center (RC) where it is used to initiate charge separation, by releasing an energetic electron for the later stages of the photosynthetic chemistry, i.e., to start chemical reactions to obtain carbohydrates. Intriguingly, the efficiency of these light reactions can often be close to 100%, suggesting a carefully optimization of the deleterious processes (decay and charge recombination, excited state trapping), which plague the attempts to carry out similar processes in artificial solar cells.
Recent experimental and theoretical studies demonstrate that quantum-mechanical effects (e.g., coherent quantum dynamics) play a previously unexpected and relevant role in biological EET (Mohseni et al., 2014). Quantum effects have been shown to survive even at ambient temperatures and are possibly beneficial to the observed very high efficiencies exhibited by these biological structures. Consequently, there has been an ever-increasing interest in understanding and modeling the nanoscaled structures and the related pigment–pigment interactions, which drive the natural light reactions so efficiently. Mimicking the physics of the photosynthetic PPCs could be extremely valuable for the future design of more efficient artificial light-harvesting structures based on the exploitation of quantum effects.
A powerful idea for recognizing the role played by quantum effects in the light reactions, one based on the fundamental principles of quantum mechanics, has recently been proposed by Scully (2010), a pioneer of theoretical quantum optics. By modeling the light reactions (photon absorption/emission, excitonic energy transfer) as a cyclic working process, analogous to a thermodynamic Carnot cycle, Scully has presented a formalism, which allows the performance of the light reactions to be analyzed in a way similar to standard thermodynamics heat engines. Within this framework, the solar photonic energy is described in terms of a “hot” thermal bath that is then converted through photon absorption to low-entropy useful work, i.e., the production of high energy electrons later used for the subsequent photosynthetic chemistry. The relevance of the quantum heat engine (QHE) approach is exemplified by the famous calculation of Shockley and Queisser (1961) who used similar thermodynamical considerations to determine the fundamental maximum efficiency of the single band-gap (junction) semiconductor solar cells (Wurfel, 2009). More specifically, Scully has shown that in photocells consisting of elements that support quantum coherence, it is possible to suppress the emission process. Spontaneous emission of photons before the absorbed energy can be used to perform any work is indeed the most important as well as unavoidable loss mechanisms in optical energy extraction. Under open-circuit conditions, i.e., when no work current is extracted, the maximum voltage that can be obtained is determined by the detailed balance of absorption and spontaneous emission. Hence, breaking the detailed balance by quenching the spontaneous emission may lead to enhanced power extraction and current generation in photocells (Scully, 2010).
An appealing direction to explore will thus combine the utility and elegance of the quantum heat engine framework and the nanoscale architecture of the highly efficient photosynthetic complexes. As an example developed along these lines, we have recently presented a scheme of a photocell inspired by the molecular elements of biological RCs (Creatore et al., 2013). In photosynthesis, the RC consists of a “special pair” of photon-absorbing pigments (donors), which are coupled through an electron-accepting molecule to a work extraction stage.
The scheme we propose harnesses well understood and experimentally observed quantum effects, such as the formation of delocalized excited (exciton) states resulting from the long-range dipole–dipole interaction (excitonic coupling) between closely spaced pigments. In our RC-inspired photocell, such dipolar interaction between the pair or light-absorbing donor molecules leads to the formation of new optically excitable states and quantum interference effects, which can enhance the photocell performance relative to a “classical” scheme in which these effects are not present (Figure 1, left panel). In particular, we have shown how dipolar couplings and related quantum interference effects can be tailored to increase the efficiency of charge transfer at the acceptor molecule, thus leading to enhanced light-to-current generation and power extraction at the reaction center. Although not a model of an actual RC, this proposal shows what could be achieved with the same resources, i.e., a pair of absorbers, an electron acceptor, and the ability to “build” the electronic structure, the quantum states, and their interactions. A recent follow-up (Zhang et al., 2015) (proposed by the Group of S. Kais and one of the groundbreakers of quantum biology, G. S. Engel) of our model has highlighted the originality of the QHE strategy for realizing PV devices based on photosynthesis, finding even greater efficiency gains in coherent trimer structures. The relevance of a new class of bio-inspired quantum nanotechnology, however, is not just restrained to reproduce/enhance efficient operations observed in nature. Higgins et al. (2014), for instance, have shown theoretically that well-established quantum control techniques can be used to achieve an enhanced photon absorption (a phenomenon called “superabsorption”) in simple ring nanostructures reminiscent of photosynthetic LHCs (Figure 1, right panel), whereas these natural systems themselves are not designed to exploit this effect. Superabsorption, which is the absorptive analog of quantum superradiance, has a range of applications including photon detection in the context of optical sensors and light-based power transmissions (Higgins et al., 2014).
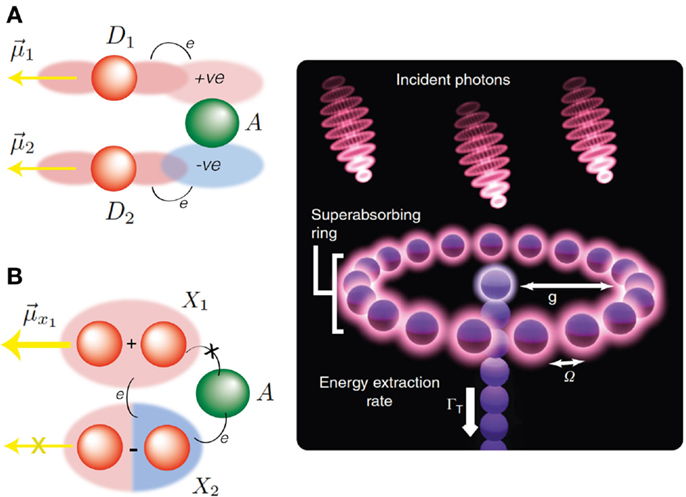
Figure 1. Left panel: the photosynthetic reaction center modeled in Creatore et al. (2013), consisting of two donor molecules (red spheres) and one acceptor molecule (green sphere). In (A), the donor D1 and D2 are identical and uncoupled and both able to absorb and re-emit light (see the dipole moments μ1 and μ2). In (B), the dipolar excitonic coupling between D1 and D2 leads to new excitable donor states (X1 and X2) resulting from the quantum superposition of the original donor states: X1 is a bright state with optical dipole moment , while X2 is a dark state, which cannot absorb or emit photons. Under a variety of realistic and experimentally realizable parameters, the presence of a dark state lowers the role of deleterious radiative recombination processes, while increases the efficiency of charge transfer at the acceptor A. This leads to an enhanced current and power generation at the RC, compared to the uncoupled/classical scheme of (A); see Creatore et al. (2013) for details. Right panel: a collection of N dipoles arranged in a ring structure reminiscent of the photosynthetic light-harvesting complex LH1. By using environmental quantum control techniques, Higgins et al. (2014) have shown that it is possible to achieve a regime (superabsorption), in which the N dipoles absorb incident photons with a rate proportional to N2, this effect being the absorptive analog of quantum superradiance. This model could be relevant to applications to solar energy harvesting, where the excitation induced by the absorption of solar photons are efficiently transferred from the ring structure to a central core absorber and then to a center converting such excitations into stored energy; see Higgins et al. (2014) for details. [Copied with permission from the original paper: Nat. Commun. 5, Article number: 4705 (2014).]
These bio-inspired schemes are potentially realizable in artificial molecular light-harvesting systems through existing organic synthesis routes. A fundamental breakthrough in this direction has been recently achieved by Hayes et al. (2013), who have engineered an artificial synthetic system (consisting of a series of rigid synthetic heterodimers) supporting the persistent electronic coherences observed in biological systems and believed to be beneficial for the process of energy transfer. Another direction for the realization of these models, one based on solid-state nanotechnology architectures, is now viable due to the increasing level of experimental control over nano-optical systems gained in recent years, and involves the use of solid-state chromophores such as quantum dots, superconducting qubits, plasmonic nanostructures, and possibly hybrid combinations of the above. From the theoretical/computational point of view, advanced simulation techniques to complement the Hamiltonian/microscopic approaches (based on setting up and solving quantum optical master equations) used in the aforementioned proposals, are required. These techniques are useful to precisely assess the optical geometry and the stability of the engineered quantum states, including the role of environmental degrees of freedom (using, e.g., time-dependent density matrix renormalization group methods) and details of additional states, e.g., charge-transfer states (using, e.g., tight binding models).
Conclusion
Innovative ideas are needed to satisfy the ever-growing interest and expectations on solar energy conversion. In this Opinion, we have presented the prospects of an emergent novel strategy to harvest solar energy based on replicating mechanisms developed in nature, and realizable using the theoretical and experimental tools of quantum mechanics. Although at a nascent stage, this framework is attracting an increasing attention and we think that the proposals here discussed suggest an encouraging trend for biologically inspired PV. Further, the technological capabilities are now fully developed for translating the theoretical schemes in experimental proofs of concept/prototypes. Besides, we believe that results and solutions established along these new lines will be beneficial to the evolving and interconnected fields of non-equilibrium (Bustamante et al., 2005) and quantum thermodynamics (Gemmer et al., 2004; Kosloff, 2013) as well. Biological processes usually take place at the micro- and nano-scale and evolve in out-of-equilibrium conditions – energy transfer and the motion of molecular motors being paradigmatic examples. The same scenario applies to nanoscale devices, which, through precise quantum-mechanical control, could be employed to unravel the thermodynamic relations between the non-equilibrium evolution of molecular quantum states and the enhanced (or reduced) working efficiency of biological or biologically inspired processes harnessing these states.
Conflict of Interest Statement
The authors declare that the research was conducted in the absence of any commercial or financial relationships that could be construed as a potential conflict of interest.
Footnote
- ^This target calls for a maximum global mean temperature rise of 2°C compared to the temperature of pre-industrial times.
References
Blankenship, R. E., Tiede, D. M., Barber, J., Brudvig, G. W., Fleming, G., Ghirardi, M., et al. (2011). Comparing photosynthetic and photovoltaic efficiencies and recognizing the potential for improvement. Science 332, 805–809. doi: 10.1126/science.1200165
Pubmed Abstract | Pubmed Full Text | CrossRef Full Text | Google Scholar
BP. (2014). Statistical Review of World Energy 2014. Available at: http://www.bp.com/en/global/corporate/about-bp/energy-economics/statistical-review-of-world-energy.html
Bustamante, C., Liphardt, J., and Ritort, F. (2005). The nonequilibrium thermodynamics of small systems. Phys. Today 58, 43–48. doi:10.1063/1.2012462
Chapin, D. M., Fuller, C. S., and Pearson, G. L. (1954). A new silicon p-n junction photocell for converting solar radiation into electrical power. J. Appl. Phys. 25, 676–677. doi:10.1063/1.1721711
Creatore, C., Parker, M. A., Emmott, S., and Chin, A. W. (2013). Efficient biologically inspired photocell enhanced by delocalized quantum states. Phys. Rev. Lett. 111, 253601. doi:10.1103/PhysRevLett.111.253601
Pubmed Abstract | Pubmed Full Text | CrossRef Full Text | Google Scholar
Fraunhofer-ISE. (2014). New World Record for Solar Cell Efficiency at 46%. Available at http://www.ise.fraunhofer.de/en/press-and-media/press-releases/press-releases-2014/new-world-record-for-solar-cell-efficiency-at-46-percent
Green, M. A. (2003). Third Generation Photovoltaics – Advanced Solar Energy Conversion. Berlin: Springer.
Green, M. A., Emery, K., Hishikawa, Y., Warta, W., and Dunlop, E. D. (2014). Solar cell efficiency tables (version 44). Prog. Photovolt. Res. Appl. 22, 701–710. doi:10.1002/pip.2452
Hayes, D., Griffin, G. B., and Engel, G. S. (2013). Engineering coherence among excited states in synthetic heterodimer systems. Science 340, 1431–1434. doi:10.1126/science.1233828
Pubmed Abstract | Pubmed Full Text | CrossRef Full Text | Google Scholar
Higgins, K. D. B., Benjamin, S. C., Stace, T. M., Milburn, G. J., Lovett, B. W., and Gauger, E. M. (2014). Superabsorption of light via quantum engineering. Nat. Commun. 5:4705. doi:10.1038/ncomms5705
Pubmed Abstract | Pubmed Full Text | CrossRef Full Text | Google Scholar
Kosloff, R. (2013). Quantum thermodynamics: a dynamical viewpoint. Entropy 15, 2100–2128. doi:10.1021/nl2011164
Pubmed Abstract | Pubmed Full Text | CrossRef Full Text | Google Scholar
Mohseni, M., Omar, Y., Engel, G. S., and Plenio, M. B. (eds) (2014). Quantum Effects in Biology. Cambridge: Cambridge University Press.
Scully, M. (2010). Quantum photocell: using quantum coherence to reduce radiative recombination and increase efficiency. Phys. Rev. Lett. 104, 207701. doi:10.1103/PhysRevLett.104.207701
Pubmed Abstract | Pubmed Full Text | CrossRef Full Text | Google Scholar
Scully, M. O., Chapin, K. R., Dorfman, K. E., Kim, M. B., and Svidzinsky, A. (2011). Quantum heat engine power can be increased by noise-induced coherence. Proc. Natl. Acad. Sci. U.S.A. 108, 15097–15100. doi:10.1073/pnas.1110234108
Pubmed Abstract | Pubmed Full Text | CrossRef Full Text | Google Scholar
Shockley, W., and Queisser, H. J. (1961). Detailed balance limit of efficiency of p-n junction solar cells. J. Appl. Phys. 32, 510–519. doi:10.1002/adma.201202873
Pubmed Abstract | Pubmed Full Text | CrossRef Full Text | Google Scholar
Keywords: solar cells, artificial photosynthesis, quantum effects in biology, quantum coherence, artificial nanostructures
Citation: Creatore C, Chin AW, Parker MA and Emmott S (2015) Emergent models for artificial light-harvesting. Front. Mater. 2:6. doi: 10.3389/fmats.2015.00006
Received: 30 December 2014; Accepted: 19 January 2015;
Published online: 10 February 2015.
Edited by:
Simone Taioli, Bruno Kessler Foundation, ItalyReviewed by:
Michele Casula, Université Pierre et Marie Curie, FranceCopyright: © 2015 Creatore, Chin, Parker and Emmott. This is an open-access article distributed under the terms of the Creative Commons Attribution License (CC BY). The use, distribution or reproduction in other forums is permitted, provided the original author(s) or licensor are credited and that the original publication in this journal is cited, in accordance with accepted academic practice. No use, distribution or reproduction is permitted which does not comply with these terms.
*Correspondence: cc619@cam.ac.uk; ac307@cam.ac.uk