Therapeutic ion-releasing bioactive glass ionomer cements with improved mechanical strength and radiopacity
- 1Otto Schott Institute of Materials Research, Friedrich Schiller University Jena, Jena, Germany
- 2Craniofacial Development and Stem Cell Biology, King’s College London, London, UK
- 3Unit of Dental Physical Sciences, Oral Growth and Development, Barts and The London School of Medicine and Dentistry, Queen Mary University of London, London, UK
Bioactive glasses (BG) are used to regenerate bone, as they degrade and release therapeutic ions. Glass ionomer cements (GIC) are used in dentistry, can be delivered by injection, and set in situ by a reaction between an acid-degradable glass and a polymeric acid. Our aim was to combine the advantages of BG and GIC, and we investigated the use of alkali-free BG (SiO2–CaO–CaF2–MgO) with 0–50% of calcium replaced by strontium, as the beneficial effects of strontium on bone formation are well documented. When mixing BG and poly(vinyl phosphonic-co-acrylic acid), ions were released fast (up to 90% within 15 min at pH 1), which resulted in GIC setting, as followed by infrared spectroscopy. GIC mixed well and set to hard cements (compressive strength up to 35 MPa), staying hard when in contact with aqueous solution. This is in contrast to GIC prepared with poly(acrylic acid), which were shown previously to become soft in contact with water. Strontium release from GIC increased linearly with strontium for calcium substitution, allowing for tailoring of strontium release depending on clinical requirements. Furthermore, strontium substitution increased GIC radiopacity. GIC passed ISO10993 cytotoxicity test, making them promising candidates for use as injectable bone cements.
Introduction
In 2010, about 5.5% of the EU population were diagnosed with osteoporosis, with 22% of women aged 50 and above (Hernlund et al., 2013). In osteoporosis, bone mass and density are reduced, owing to osteoclasts resorbing too much bone and osteoblasts not forming enough new bone, resulting in an increased risk of fractures. Incidences of clinical vertebral fractures in the EU have been reported to range from 170 to 470 per 100,000 (Hernlund et al., 2013), and about a third of these fractures can be persistently painful after non-operative care (Agency for Healthcare Research and Quality, 2010). Vertebroplasty and kyphoplasty are common procedures which restore fractured vertebrae by injecting a bone cement such as poly(methyl methacrylate) (PMMA) (Lewis, 2006), and the aim is to relieve pain while restoring dimensions and strength of the vertebrae. Glass ionomer cements (GIC) have the potential to overcome some of the limitations of acrylic cements, such as shrinkage during polymerization and a highly exothermic setting reaction, as they set by an acid–base reaction between a polymeric acid [such as poly(acrylic acid), PAA] and a degradable glass (Wilson, 1996). Furthermore, GIC form a chemical bond to bone (Wilson et al., 1983), which is in contrast to acrylic cements, where mechanical stability is achieved by mechanical interlocking only.
Several commercial dental GIC contain strontium in the glass component (Stamboulis et al., 2004), and the release of strontium ions has been shown to enhance tooth remineralization (Thuy et al., 2008) and promote antibacterial properties (Guida et al., 2003; Brauer et al., 2013), particularly in synergy with fluoride ions (Dabsie et al., 2009). Furthermore, strontium ions are known to enhance osteoblastic bone formation and reduce osteoclastic bone resorption (Marie et al., 2001), and they are used for the treatment of osteoporosis (Marie, 2005). Strontium-containing bioactive glasses (BG) have been shown to release strontium ions when in contact with aqueous solutions (Fredholm et al., 2012), and they have been suggested to combine the benefits of strontium ions with those of BG (bioactivity, apatite formation, controlled release of therapeutic ions, and delivery versatility) (Gentleman et al., 2010; Autefage et al., 2015).
Dental GIC contain aluminum ions, which play an important role in both glass degradation (hydrolysis of Si–O–Al bonds) and in stability of the cements (cross-linking of PAA chains by Al3+); however, as they are neurotoxic (Joshi, 1990) and negatively affect bone mineralization (Cournot-Witmer et al., 1981; Boyce et al., 1982; Blades et al., 1998), dental GIC are not ideal for orthopedic applications. Efforts have been made to replace aluminum with iron (Hurrell-Gillingham et al., 2006) or zinc (Darling and Hill, 1994); however, these also have drawbacks and particularly too high a zinc release has been shown to result in cytotoxic reactions (Brauer et al., 2011a). Materials based on magnesium-containing BG can therefore provide a promising alternative.
In previous studies, GIC based on magnesium-containing BG and PAA have been shown to be promising candidates for use as bone cements (Brauer et al., 2011a, 2013) and to show antibacterial properties (Brauer et al., 2013). However, their mechanical properties and hydrolytic stability were poor compared to GIC using aluminum- or zinc-containing glasses (Brauer et al., 2011a). Our hypothesis was that a polyelectrolyte with a larger concentration of functional groups would allow for formation of more hydrolytically and mechanically stable GIC, and here we therefore prepare GIC with poly(vinylphosphonic-co-acrylic acid) (PVPA–PAA) rather than PAA.
Our aim was to characterize the setting behavior, mechanical properties, ion release, and in vitro cell compatibility of GIC based on PVPA–PAA and BG (SiO2–CaO–CaF2–MgO) with 0, 2.5, 10, or 50% of Ca replaced by Sr on a molar base.
Materials and Methods
Glass Synthesis and Characterization
Bioactive glasses (SiO2–CaO–CaF2–MgO) where either no calcium (Sr0) or 2.5, 10, or 50% of calcium (Sr2.5, Sr10, Sr50) were replaced by strontium on a molar base (Table 1) were prepared using a standard melt-quench route as described previously (Brauer et al., 2013). Glasses were mixed with isopropanol and ground for 50 min in an agate planetary mill (Pulverisette, Fritsch GmbH, Idar-Oberstein, Germany) and then sieved using a 40 μm analytical sieve. The amorphous state of the glasses was investigated using powder X-ray diffraction (XRD; D5000, Siemens) using CuKα radiation and then measured with a step time of 31 s and step width of 0.02° in the range of 10°–60° 2Θ. Thermal properties of the glasses were analyzed using differential thermal analysis (DTA; DTA-50, Shimadzu; heating rate, 10 K min−1). Glass composition was analyzed using scanning electron microscopy with energy-dispersive X-ray spectroscopy (EDX) with fluoride (Field Emission JEOL 7001 F, EDAX Trident EDX; Kα; 25 keV).
Glass Dissolution
Glass solubility was analyzed in a hydrochloric acid/potassium chloride (HCl/KCl) buffer, which was obtained by mixing 134 mL of 0.2 mol L−1 hydrochloric acid solution (Titrisol, Merck, p.a.) with 50 mL of 0.2 mol L−1 potassium chloride (VEB Jenapharm Laborchemie Apolda, p.a.) solution, filling to a total volume of 200 mL and storing at 37°C. Seventy-five milligrams of glass powder was immersed in 50 mL buffer solution and stored at 37°C for 5, 15, 60, 360, or 1440 min. Afterward, solution and remaining powder were separated by filtering using medium porosity filter paper (5 μm particle retention, VWR). The remaining powder was analyzed using attenuated total reflectance Fourier transform infrared spectroscopy (ATR-FTIR; Alpha, Bruker Daltonic GmbH, Bremen, Germany) and the solutions were analyzed using inductively coupled plasma optical emission spectroscopy (ICP-OES; Varian Liberty 150, Agilent Technologies, Böblingen, Germany). Data represent mean ± standard deviation (SD).
Cement Formation
Before mixing cements, glass powders were annealed by heating to and holding for 10 min at 50 K below glass transition before being allowed to cool to room temperature. Cements were prepared using poly(vinyl phosphonic-co-acrylic acid) (PVPA–PAA; 40 wt% solution; weight average chain length between 40 and 70 kD according to manufacturer), which was provided by First Scientific Dental GmbH (Elmshorn, Germany). Cements were obtained by mixing glass powder and polymer solution in a weight ratio of 2:1, giving the cement into PTFE molds (4 mm in diameter; 7 mm in height) and allowing to set inside the mold for 60 min at 37°C.
Cement Setting Behavior and Mechanical Properties
Cement setting was followed by ATR-FTIR, by placing the freshly mixed cement onto the diamond window of the spectrometer and obtaining a spectrum every 5 min for up to 60 min. FTIR spectra of the untreated glasses and PVPA–PAA solution were measured for comparison.
Mechanical properties of the cements were measured on specimens which (after setting at 37°C for 60 min) were removed from the mold and kept for 23 h either in deionized water or at 100% relative humidity (100% RH) at 37°C. For storing at 100% RH, specimens together with some damp tissue were placed inside a closed container; physical contact between specimen and tissue was avoided. Specimens without treatment in deionized water or at 100% RH (thus set for 60 min at 37°C inside the mold only) were analyzed as controls. Compressive strength and Young’s modulus were measured using a hydraulic testing machine (Zwick 1445, Zwick GmbH, Ulm, Germany) with a 10 kN load cell at a test velocity of 1 mm min−1. Per composition, 10 separate specimens were tested. Results were analyzed using one-way analysis of variance (ANOVA; Origin 8.5.0, OriginLab Corp., Northampton, MA, USA) followed by Bonferroni post hoc test; p ≤ 0.05 was considered significant. Results are presented as mean ± confidence interval.
Radiopacity
Glass ionomer cement radiopacity was measured according to the specifications laid out in BS EN ISO 9917-1:2007 standard for water-based dental cements. One-millimeter-thick GIC disks 10 mm in diameter were prepared by packing the cement mixture into Teflon molds of the same dimensions. GICs were allowed to set in the molds for 1 h while clamped between two acetate sheets. Thereafter, the disks were stored at 37°C and 100% RH. After 24 h, the samples were removed from the molds and radiographed, along with a six-step aluminum step wedge (0.5 to 5.0 mm), using a digital X-ray unit. The samples and the step wedge were irradiated at 65 kV from a distance of 10 cm. Exposure time was 0.4 s. Exact height of the cement disks was measured using a micrometer, and measured radiopacity was normalized to a specimen height of 1.0 mm. Measurements were performed in triplicates and results are presented as mean ± SD.
Cell Culture, Cement Cytotoxicity (ISO 10993), and Ion Release
MC3T3-E1 mouse osteoblasts were obtained from the European Collection of Cell Cultures (Salisbury, UK) and cultured under standard conditions (37°C, 5% CO2/95% air, 100% RH) in Alpha Minimum Essential Medium (αMEM) supplemented with 10% (v/v) fetal bovine serum (FBS) and 2 mM l-glutamine (all from Invitrogen, Paisley, UK).
To create conditioned media for cytotoxicity testing (ISO 10993:5), 10 mm diameter, 1 mm thick cement disks, organo-tin stabilized poly(vinyl chloride) (PVC) sheet (positive control), and non-toxic PVC (noDOP®) tubing (negative control) were prepared. Positive and negative control samples were provided by Raumedic (Münchberg, Germany) and sterilized in 70% ethanol for 1 h. Cement samples were sterilized under UV light for 2 h on each side.
Conditioned media were created by soaking cement samples or positive and negative control samples in αMEM for 7 days at 37°C. A material surface area to culture medium volume ratio of 3 cm2 mL−1 was maintained as directed in ISO10993:5. Cells were seeded at 20,000 cells/cm2 in 96-well plates in basal medium and allowed to attach for 24 h. Culture medium was then exchanged with conditioned medium supplemented with 10% FBS and 2 mM l-glutamine. Conditioned medium was added to cells either neat or diluted by factors of 2, 4, 8, or 16 with basal medium and allowed to incubate for 24 h. Cell metabolic activity was determined by MTT (3-(4,5-Dimethylthiazol-2-yl)-2,5-diphenyltetrazolium bromide; Sigma-Aldrich, Dorset, UK) assay, as previously described (Gentleman et al., 2010). Briefly, 20 μL of a 5 mg/mL solution of MTT in phosphate buffered saline was added to each well and plates were returned to the incubator for 4 h. Culture medium was then removed, the formazan product dissolved in dimethyl sulfoxide, and the solution’s absorbance was read on a colorimetric plate reader at 592 nm. Data represent mean (± SD) of three separate samples for each composition or control and are normalized to negative controls. Statistical analysis of MTT activity was carried out by ANOVA followed by post hoc Tukey test and was limited to comparisons carried out with the non-diluted conditioned medium. Differences were considered significant if p < 0.05. Ions released into the cell culture medium were analyzed using ICP-OES (calcium, strontium, magnesium, and silicon) and fluoride-selective electrode (fluoride). For ICP-OES analysis, solutions were diluted by a factor 1:20 and acidified using 69% nitric acid before analysis. Fluoride concentrations were measured using a fluoride ion selective electrode (Elit 8221, Nico2000 Ltd.) with a AgCl reference electrode.
Results
Glass Characterization
All glasses were amorphous according to powder XRD results, with XRD patterns displaying the typical amorphous halos (Figure 1A). Significant amounts of fluoride were lost during melting (Brauer et al., 2011b), resulting in overall changes in the glass compositions as presented in Table 2. DTA curves (Figure 1B) and summarized thermal properties (Table 3) show comparable thermal behavior for glasses Sr0, Sr2.5, and Sr10 but lower glass transition and higher crystallization temperatures for Sr50.
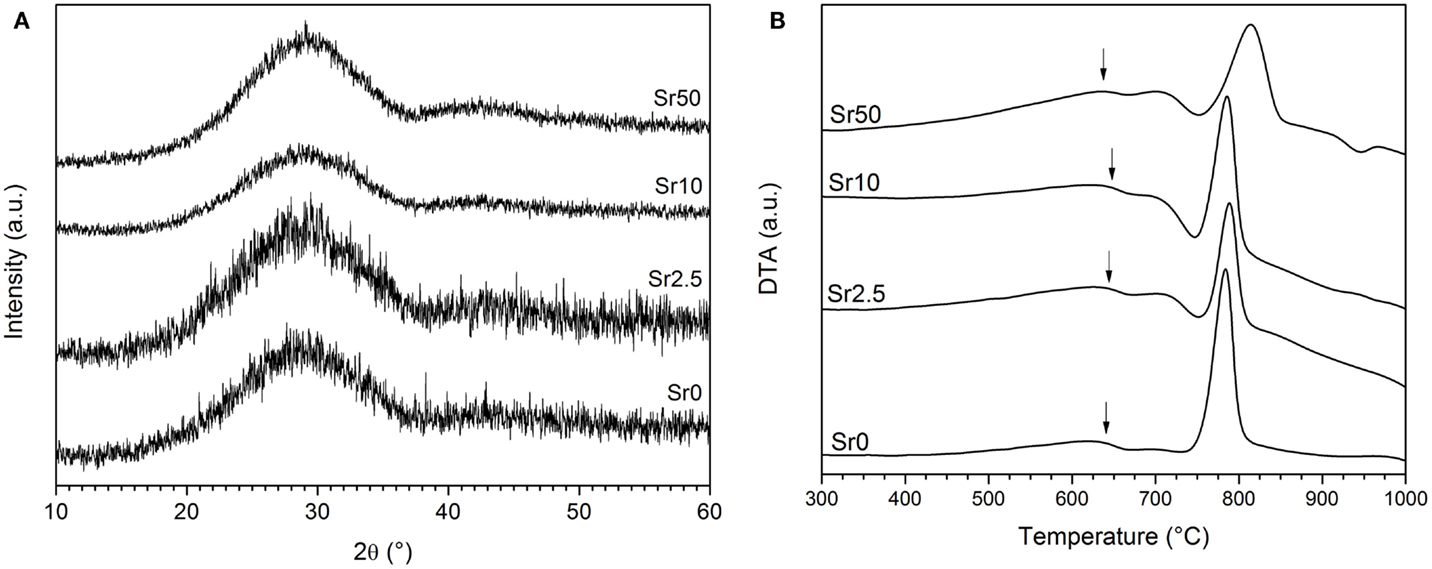
Figure 1. (A) XRD patterns of the glasses, showing the typical amorphous halo. (B) DTA traces of the glasses, showing glass transition temperatures (Tg) highlighted by arrows and crystallization exotherms.
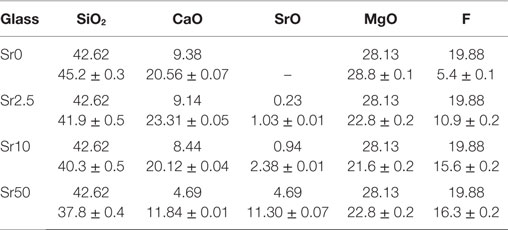
Table 2. EDX results compared to nominal glass composition (mol%; fluoride content presented as F; Ca and Sr presented as oxides only).
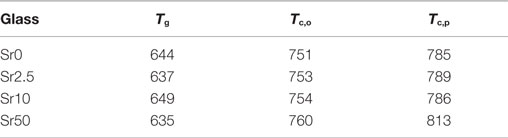
Table 3. DTA results: glass transition (Tg), crystallization onset (Tc,o), and crystallization peak (Tc,p) temperatures (°C), error is ±5 K.
Glass Dissolution
Experiments were performed in a buffer solution set to pH 1, as this was the pH of the PVPA–PAA solution. Upon immersion of glass powders, a fast pH rise was observed (Figure 2A). A pH of 1.15 was reached within the first few minutes and pH 1.2 at 6 h, where it remained for the duration of the experiment (24 h). This was accompanied by a release of ions (Figure 2B), where a very fast release was observed within the first minutes of the experiments, and concentrations increased more slowly afterward. Absolute concentrations shown in Figure 2B correspond to a release of about 90% of calcium and 75% of strontium and magnesium ions within the first hour, reaching 100 and 90%, respectively, at 24 h. Release of silicon ions ranged from about 10% at 5 min to 20% at 24 h. This ion release caused structural changes observed by FTIR, where the non-bridging oxygen band at 930 cm−1 had already disappeared at an immersion time of 5 min, and no pronounced changes in FTIR spectra were observed afterwards (Figure 2C). Release of Mg2+ and silicon ions did not vary much with strontium for calcium substitution in the glass. By contrast, concentrations of Ca2+ ions decreased with strontium for calcium substitution in the glass (owing to lower amounts of Ca2+ ions present in the glass) and concentrations of Sr2+ ions increased linearly (R2 = 0.999; Figure 2D).
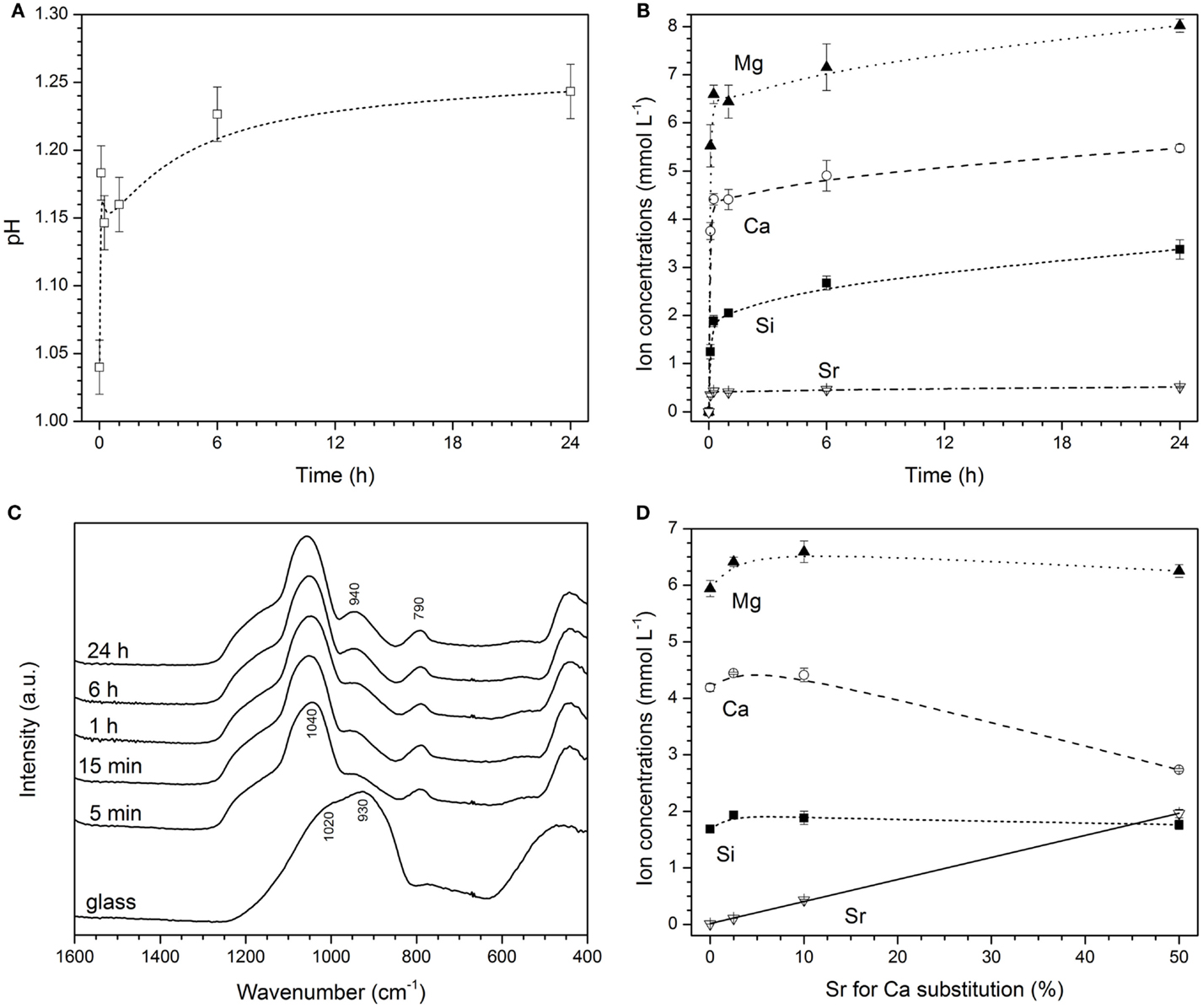
Figure 2. Changes in (A) pH, (B) concentrations of ions in HCl/KCl buffer, and (C) FTIR spectra with immersion time of glass Sr10. (D) Changes in concentrations of ions with strontium for calcium substitution in the glass for glasses immersed in HCl/KCl buffer for 15 min (solid line: linear regression; R2 = 0.999).
Cement Formation and Properties
Cements from PVPA–PAA and glasses Sr0 and Sr2.5 showed very good mixing properties; however, with increasing strontium for calcium substitution, the glasses became more reactive and working times shorter, with glass Sr50 setting very quickly. All glasses, when mixed with PVPA-PAA solution, set to hard and brittle cements within 1 h at 37°C. Cements stayed hard when stored in water or at 100% RH.
Poly(vinylphosphonic-co-acrylic acid) solution showed pronounced bands at about 1715, 1640, 1170, 995, and 930 cm−1, with additional low-intensity bands at 1455 and 1415 cm−1 (Figure 3). Upon mixing with the glass powder, the band at 1715 cm−1 disappeared, new bands appeared at 1560, 1050, and 977 cm−1, and the two bands at 1455 and 1415 cm−1 increased in intensity. The band at 1715 cm−1 can be assigned to the symmetric stretch of the carboxylic –C=O band (Socrates, 2004). Upon reaction with the glass and formation of the polysalt matrix, i.e., a change from mostly carboxyl (–COOH) to carboxylate groups (–COO−), the –C=O band disappeared, owing to the π electrons in the carboxylate group being delocalized. A similar effect is observed with the –P=O stretch vibration band at 1170 cm−1 (Socrates, 2004), which also disappeared once the salt matrix of the GIC has formed. The band at 1642 cm−1 may be related to –C=O or possibly –P=O bonds, connected by hydrogen bonds (Socrates, 2004), and the intensity also decreased upon salt formation. The band at 1050 cm−1 is most likely related to P–O vibrations in the salt matrix, while the high intensity band formed at 1560 cm−1 and the double band at 1455 and 1415 cm−1 are related to asymmetric and symmetric –COO− stretch bands, respectively (Socrates, 2004).
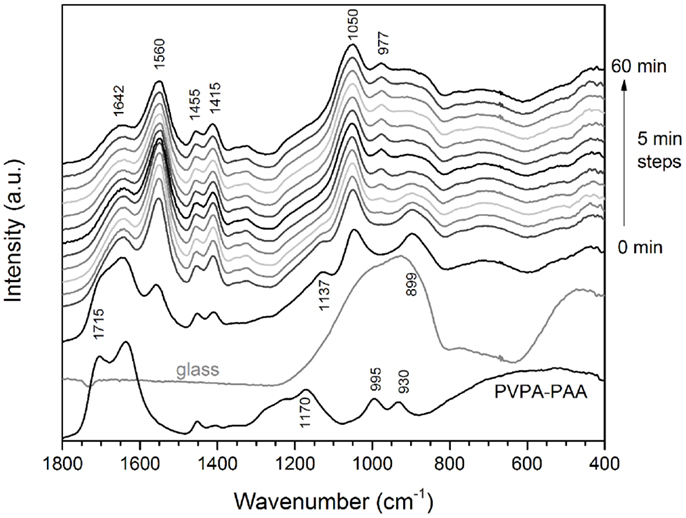
Figure 3. Changes in FTIR spectra with setting time for cements made using glass Sr10. Spectra of PVPA–PAA solution and the untreated glass are given for comparison.
Cements gave average compressive strength of at least 20 MPa and Young’s moduli of 4 GPa and above (Figures 4A,B, respectively). There seemed to be no clear trend in mechanical properties (average compressive strength or Young’s modulus) with glass composition; but both compressive strength and Young’s modulus seemed to increase with storage time in water and particularly at 100% RH. Maximum compressive strength was between 38 and 39 MPa for cements made from Sr0 and Sr2.5 at 24 h at 100% RH, while maximum compressive strength obtained for cements from glasses Sr10 and Sr50 were between 30 and 35 MPa (Figure 4C). Maximum Young’s moduli showed no clear trend but seemed to decrease with increasing strontium for calcium substitution in the glass (Figure 4D).
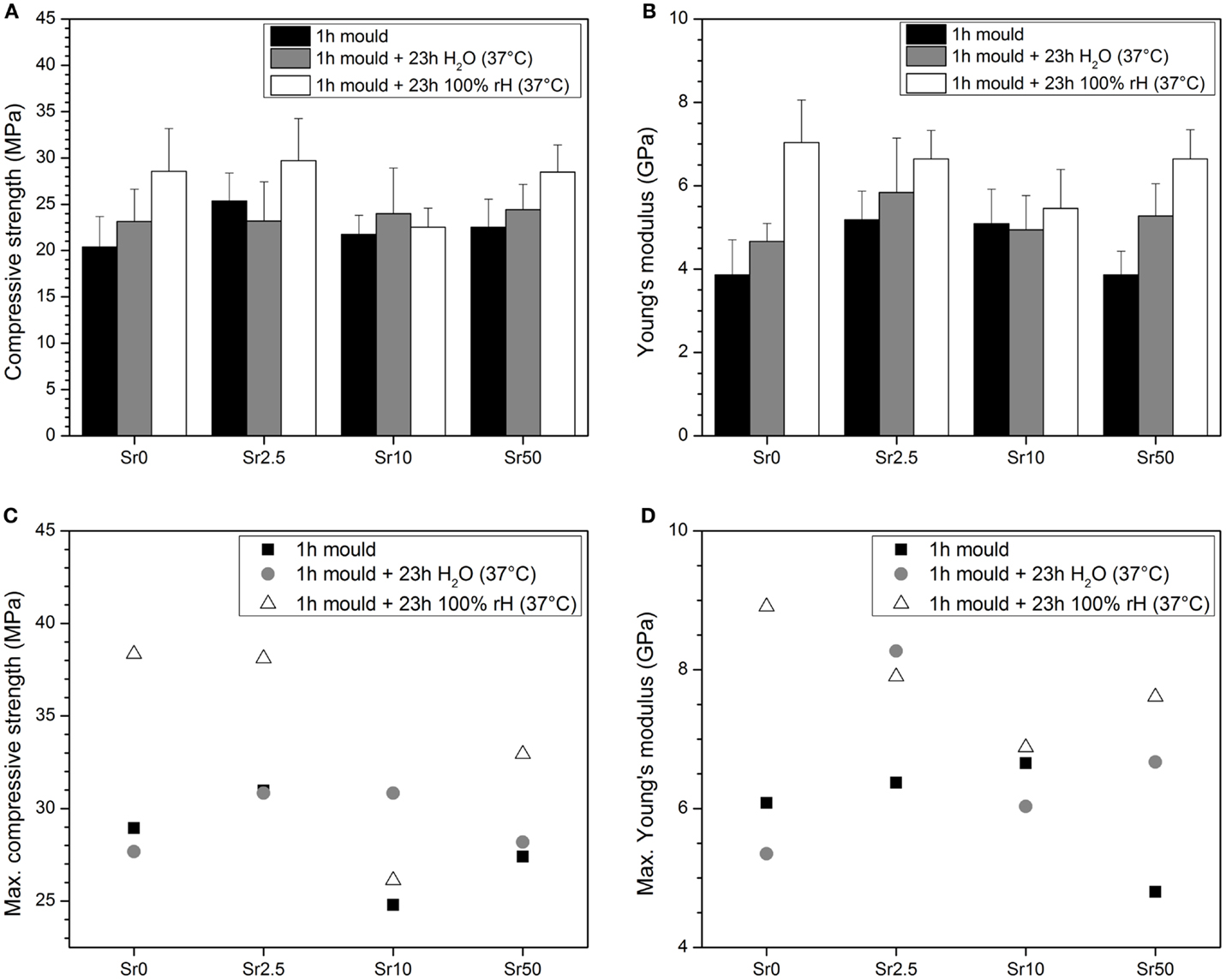
Figure 4. Mean (A) compressive strength and (B) elastic modulus and maximum (C) compressive strength and (D) elastic modulus obtained from compression tests on cement samples.
Radiopacity increased with increasing strontium for calcium substitution in the glass (Figure 5A), showing a linear increase for substitutions from 2.5 to 50% (R2 = 0.999; Figure 5B). Cements from glass Sr10 showed radiopacity comparable to that of aluminum, while radiopacity of cements from glasses Sr0 and Sr2.5 was lower and that of Sr50 well above it (225% of that of aluminum). The radiopacity of cement from glass Sr0 was off scale, i.e., lower than the first step of the step wedge (0.5 mm Al), and it is therefore not included in Figure 5B.
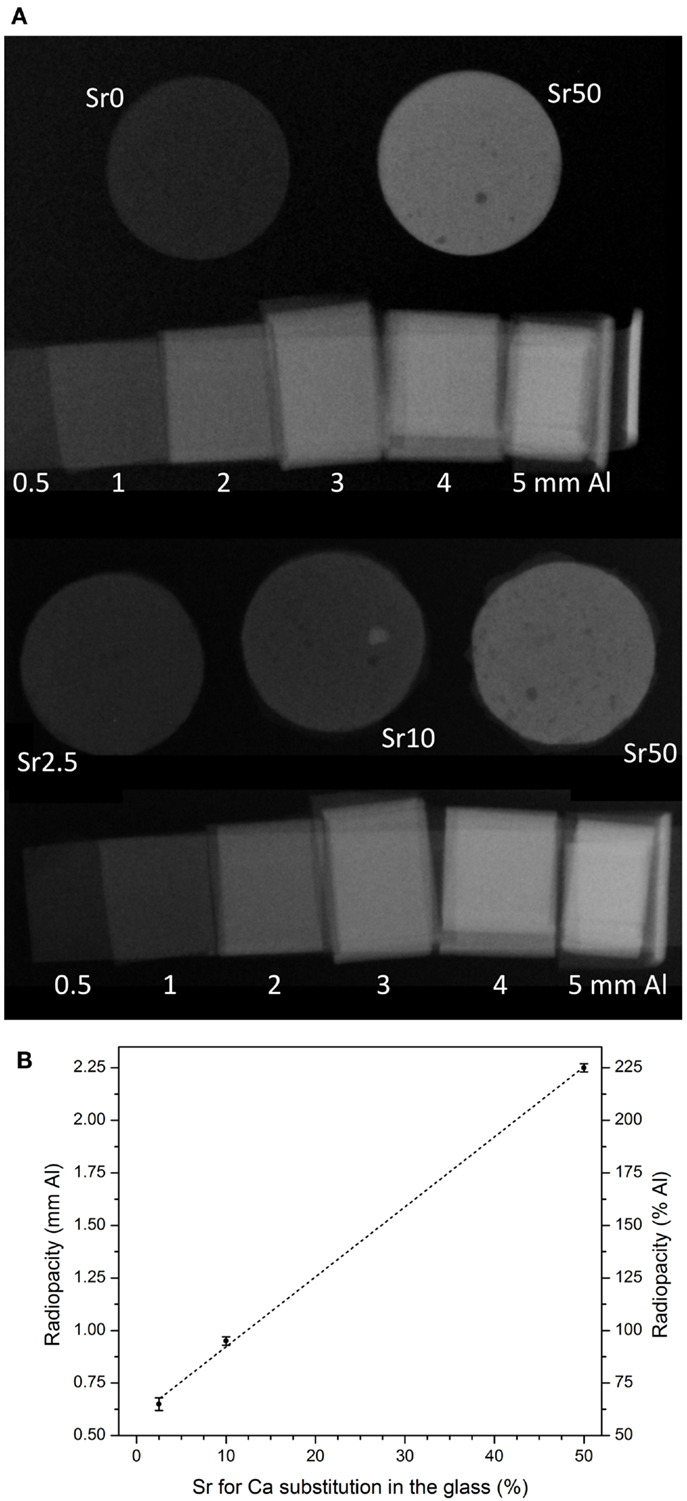
Figure 5. (A) Radiographic images of cement disks and aluminum step wedge and (B) radiopacity of cement disks (1 mm in height) vs. strontium for calcium substitution in the glass. Dashed line is linear regression (R2 = 0.999).
Cytotoxicity and Ion Release into Cell Culture Medium
MC3T3-E1 cells exposed to conditioned medium soaked with the negative control had normal morphologies, whereas those cultured with conditioned medium soaked with the positive control had abnormal rounded morphologies and often detached from the culture surface (Figures 6A,B, respectively). Quantification of cell metabolic activity by MTT assay demonstrated that cells exposed to conditioned medium soaked with the positive control had significantly lower metabolic activity than those exposed to conditioned medium soaked with the negative control (non-diluted, p < 0.001) (Figure 7A). MC3T3-E1 exposed to medium soaked with cements made from glasses Sr0 to Sr10 had normal morphologies (Figures 6C–E) and their metabolic activities were never significantly lower than that of the negative control. Cells exposed to medium soaked with cement from glass Sr50 contained cells with normal morphologies but also some rounded cells (Figure 6F); however, their metabolic activity was not significantly different from that of the negative control (p > 0.05).
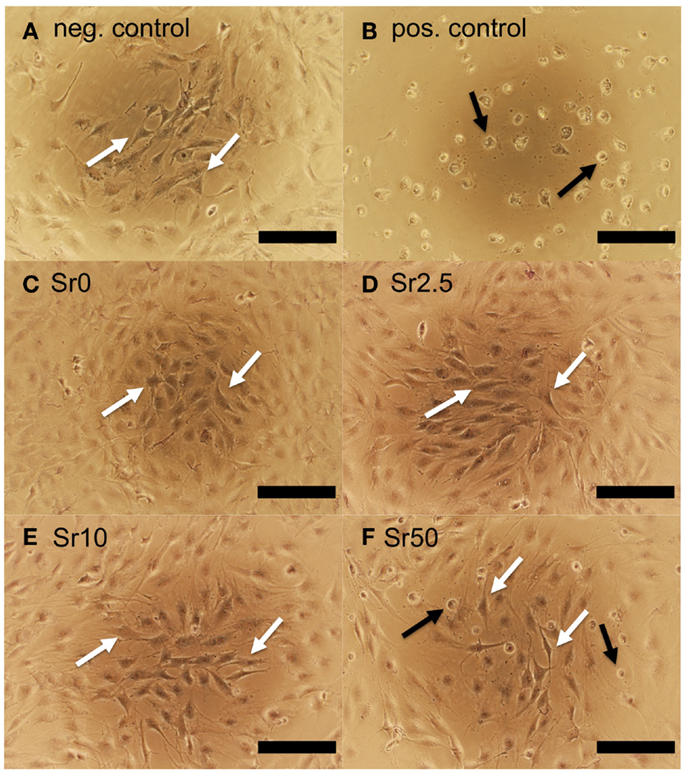
Figure 6. MC3T3-E1 cells cultured for 24 h in conditioned medium soaked with (A) negative and (B) positive control and (C–F) cements prepared with glasses Sr0 to Sr50. Examples of cells with normal morphologies are indicated with white arrows; cells with poorly spread morphologies are indicated with black arrows (scale bar is 200 μm).
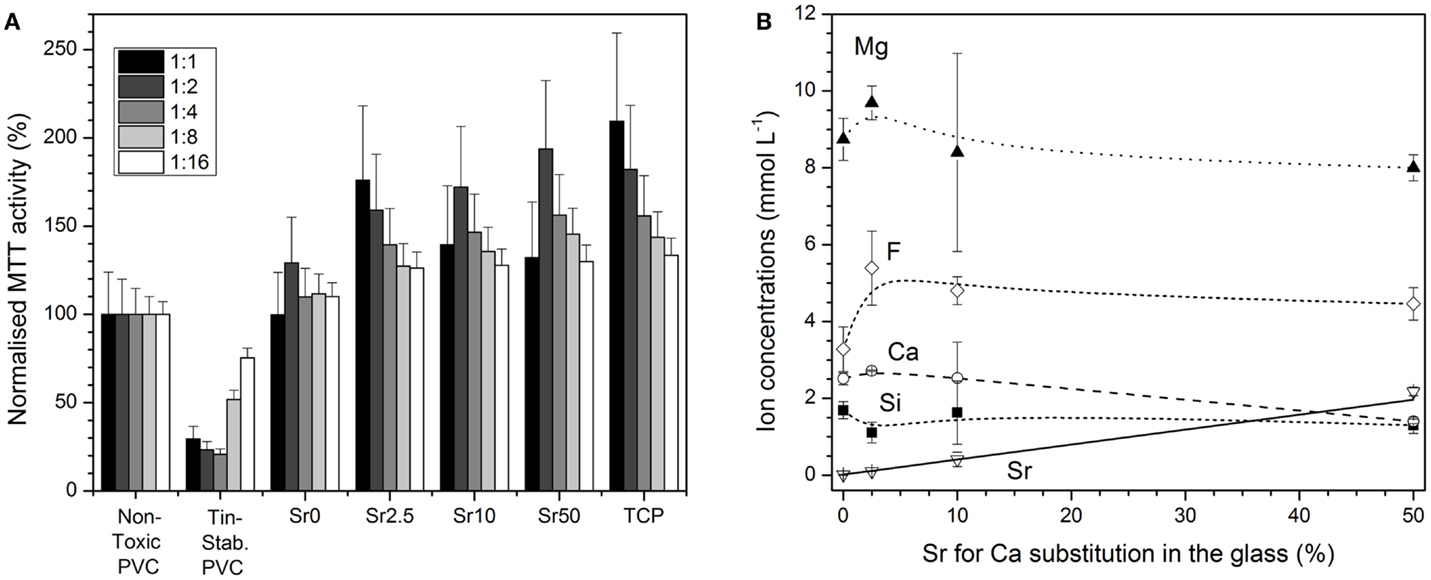
Figure 7. (A) Cytotoxicity assessment (ISO10993:5): Normalized MTT activity of MC3T3-E1 cells cultured for 24 h in conditioned medium (undiluted and diluted by factors 1:2 to 1:16) soaked with negative or positive control or cements made from glasses Sr0 to Sr50. (B) Concentrations of ions in cell culture medium vs. strontium for calcium substitution in the glass (solid line: linear regression; R2 = 0.999).
Concentrations of Mg2+, Ca2+, F−, and silicon ions in cell culture medium soaked with cements from glasses Sr0 to Sr50 did not vary greatly with glass composition. The only exception were the concentrations of Sr2+ ions, which increased linearly (R2 = 0.999) with strontium for calcium substitution in the glass (Figure 7B).
Discussion
Bioactive glasses are successfully used to regenerate bone; they degrade in physiological solutions and release ions to stimulate bone formation. When in contact with aqueous solutions, particularly at low pH (Bingel et al., 2015), BGs show a fast release of modifier ions (Hoppe et al., 2011; Brauer, 2015), including sodium, calcium, strontium, or magnesium ions (Fredholm et al., 2012; Bingel et al., 2015; Blochberger et al., 2015). This ability of BG to release ions can also be exploited for preparing GIC. The glasses in the present study were shown to release ions fast when in contact with a solution of a pH comparable to that of the PVPA–PAA solution used (Figure 2B), with the majority of ions being released during the first 5 min of immersion. Over the remaining time of the experiment (up to 24 h) ionic concentrations kept increasing, but much more slowly. When following changes in glass structure during immersion by FTIR (Figure 2C), it became obvious that indeed after the initial dramatic changes over the first 5 min (where the non-bridging oxygen band disappeared nearly completely) no pronounced structural changes seemed to occur.
Dental GICs set by a neutralization reaction between a polymeric acid and an acid-degradable (fluoro–) aluminosilicate glass (Wilson, 1996). In aluminosilicate glasses, aluminum, an intermediate element according to Dietzel’s rules (Dietzel, 1941), has been shown to be present in fourfold coordination mostly, forming Si–O–Al bonds and groups, which are charge-balanced by modifier cations (Neuville et al., 2006). These Si–O–Al bonds are readily hydrolyzed at low pH (Griffin and Hill, 1999), allowing for rapid glass degradation and ion release, which enables the GIC to set by formation of ionic bridges between carboxylate groups by metal cations such as Al3+. As aluminum ions are known to be neurotoxic (Joshi, 1990) and negatively affect bone mineralization (Cournot-Witmer et al., 1981; Boyce et al., 1982; Blades et al., 1998), magnesium ions have been studied as a possible alternative.
This makes the use of magnesium-containing BG of particular interest here, as it has been suggested that MgO acts as an intermediate oxide in BG, partially entering the silicate network (Watts et al., 2010). This would mean that some magnesium is present as Si–O–Mg bonds, which may be acid hydrolyzable in analogy to Si–O–Al bonds in dental GIC (Griffin and Hill, 1999). And although recent results showed that there is no pronounced difference in the release patterns of Ca2+ and Mg2+ ions from BGs (Blochberger et al., 2015), Mg2+ ions with their smaller ionic radius compared to calcium ions [Mg2+ 0.072 nm; Ca2+ 0.100 nm; both sixfold coordination (Shannon, 1976)] and thus higher field strength may be of interest for effective cross-linking of the carboxylate groups in the GIC matrix.
Cement setting and subsequent mechanical properties are influenced by a range of (to some extent interconnected) factors. Ion release from the glass has a strong influence on cement setting, and working and setting time are likely to depend on how fast ions are actually released from the glass. Ion field strength is likely to affect both cement setting and mechanical properties, with small cations of high charge, e.g., Al3+, resulting in better setting and improved mechanical properties compared to, e.g., alkaline earth metal cations. The polymeric acid component has also been shown to affect setting and mechanical properties, with molecular weight being one factor here and other factors including the type and number of functional groups per monomer unit (Hill et al., 1989; Griffin and Hill, 1998). These functional groups were observed in FTIR spectra (Figure 3), and the spectra allowed for following the reaction between PVPA–PAA and the glass, particularly by the decrease in intensity of carboxyl and phosphonic acid groups, and the increase in intensity of the corresponding salt bands.
In the present study, we did not see a pronounced influence of glass composition on GIC mechanical properties (Figure 4), but maximum Young’s moduli seemed to decrease with increasing strontium for calcium substitution in the glass (Figure 4D). This may be explained by the larger ionic radius of Sr2+ compared to Ca2+, which can be expected to result in it being a slightly less effective ion for cross-linking of the polycarboxylate chains. It was also noticeable that the glass with the highest strontium for calcium substitution showed a shorter working time and poorer mixing properties than glasses with lower substitutions. Indeed, it was very difficult to obtain a homogeneously mixed cement of glass Sr50 unless the glass powder was annealed first to reduce its reactivity. For all other glass compositions, it was possible to obtain cements from both annealed and non-annealed glass powders, although annealing resulted in increased working times and easier mixing. This influence of strontium for calcium substitution is likely to be related to the ion release from the glass. Relative ion release, i.e., the percentage of ions released from a glass, has been shown to increase with strontium for calcium substitution in BG (Fredholm et al., 2012) owing to a lower oxygen density, i.e., an expanded silicate network, in the glass (Fredholm et al., 2010; Du and Xiang, 2012), caused by the larger ionic radius of Sr2+ ions compared to Ca2+ ions (Martin et al., 2012). The same effect is likely to have influenced not only ion release and subsequent cement setting in the present study but also the lower Tg and crystallization temperature (Lotfibakhshaiesh et al., 2010) observed for glass Sr50 (Table 1). The effect of annealing on working times may possibly be explained by the removal of surface stresses introduced during the grinding process.
In our previous study, we showed that GIC prepared from the same glass compositions but using PAA (rather than PVPA–PAA) as the polymeric acid exhibited poor hydrolytic stability (Brauer et al., 2011a, 2013), with the cements becoming soft when in contact with water. In the present study, all cements stayed hard during storage in either water or at 100% relative humidity. This difference was probably caused by differences in the functional groups of the polymers, as PAA only has one carboxyl group per repeating unit, while PVPA–PAA has a phosphonic acid group, i.e., a diprotic acid group, in addition to the carboxyl group (Figure 8). This provides for more protons to attack the glass but also, once deprotonated, for more ionic cross-links to form between metal cations and the polymeric acid.
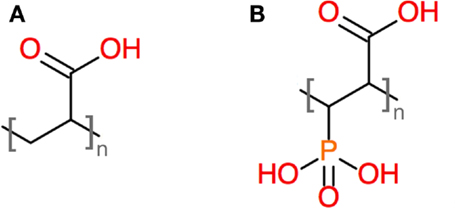
Figure 8. Structures of repeating units of (A) PAA and (B) a copolymer of acrylic acid and vinylphosphonic acid.
The radiopacity of implants is often increased in order to enable or facilitate visualization of the material during medical imaging such as radiography or fluoroscopy, and radiopacifiers such as barium sulfate are commonly added to cement systems including acrylic cements (Jasper et al., 2002). Density and atomic number are two main factors influencing the radiopacity of a material, and if high atomic number elements are present in a material, it can be expected to possess an inherent radiopacity. Incorporation of strontium into calcium phosphate cements has been shown to increase their radiopacity (Schumacher and Gelinsky, 2015), and in a similar effect, increasing strontium for calcium substitution in the glass, and thus the presence of increasing amounts of strontium ions in the GIC, made the GIC in the present study increasingly radiopaque (Figure 5).
Comparing the radiopacity of these GICs to that of bone slices of the same thickness is complicated owing to the lack of available literature. Radiographic images of alveolar sockets and surrounding bone, however, showed radiographic densities of about 1.6 mm Al (Fernandes Gomes et al., 2006). While no exact thickness of the bone was given, the thickness was more than 1 mm (i.e., more than the thickness of our GIC specimens here), as the radiographs showed the entire jawbone. This suggests that the radiopacity of the GIC prepared with Sr50 is well above that of bone and that even a lower strontium for calcium substitution (e.g., 30–40%) might be sufficient to obtain radiopaque cements, which would show in a radiograph.
If implant materials are used to fill bone defects, their role as void filler can be combined with a role as a release device for therapeutic agents. For inorganic implant materials, such as BG or GIC, an easy way of achieving this is by incorporating ions which can be released into the body and perform their therapeutic action there (Hoppe et al., 2011). Key is here to keep ionic concentrations in the range where therapeutic benefits are obtained but no toxic effects are observed. Therefore, caution should be exercised when substituting reportedly therapeutic ions into BG or GIC. Although zinc, for example, plays a number of essential biological roles in the body, particularly in bone formation, toxicity can result if the concentrations released are too high (Brauer et al., 2011a).
Glass ionomer cement studied here released various ions originating from the BG, but did not show any cytotoxic effects according to ISO 10993:5 (Figure 7). Instead, they performed at least as well as the non-toxic PVC control. GICs released about 1.5–2 mM of silicon ions (Figure 7B), originating from degradation of the BG silicate matrix. Ionic dissolution products from BG have been shown to increase osteoblast proliferation via gene upregulation (Xynos et al., 2000), and silicon ions are thought to play a key role here (Hoppe et al., 2011). The great benefit is that supplementation with growth factors is not necessary when using BG, owing to the stimulatory effects of silicon and also calcium ions (Jones, 2013). If BGs are incorporated into GIC, comparable benefits through ion release may possibly be observed.
Apart from adding radiopacity to the cements, the presence of strontium in the glass allowed for the release of strontium ions (Figure 7B), which, when released from BG, showed anabolic and anticatabolic effects in vitro (Gentleman et al., 2010). And, indeed, strontium ions are the active component in a drug for the treatment of osteoporosis (Marie, 2005; Bonnelye et al., 2008). In the present study, strontium concentrations in cell culture medium were between 0.1 (Sr2.5) and 2.2 mM (Sr50), which corresponds to a concentration range from 9 to 192 ppm. Concentrations in a similar range were recently shown to stimulate osteoblasts (25 ppm) and inhibit osteoclasts (80 ppm) (Gentleman et al., 2010). Strontium ions released from GIC have also previously been shown to have antibacterial properties and reduce cell counts of Streptococcus faecalis or Staphylococcus aureus when present in concentrations between 0.16 and 2.5 mM (Brauer et al., 2013).
The benefits of fluoride ions in preventing dental caries are well documented (Featherstone, 2000), and, indeed, the release of fluoride ions is one of the advantages of using dental GIC (Ewoldsen and Demke, 2001). However, fluoride ions also affect bone health. As high concentrations of fluoride, traditionally administered orally as sodium fluoride, are not effective in preventing fractures (Aaseth et al., 2004), its use for treating osteoporosis was dismissed for several years. Recently, however, the use of low fluoride concentrations, in combination with other therapeutic agents, is being reexamined (Reid et al., 2007). In addition, synergistic effects of fluoride and strontium ions in promoting antibacterial activity have been suggested (Dabsie et al., 2009). Cell culture studies on fluoride-releasing BGs showed that low concentrations of fluoride released from the glass (between 1.5 and 1.8 ppm) enhanced cell attachment and proliferation, while initial high release of fluoride ions (between 10 and 35 ppm) followed by much reduced release (0.3 to 2 ppm) through formation of a protective fluoroapatite surface layer on the BGs showed significantly increased alkaline phosphatase (ALP) activity, a marker for mineralization, indicating that fluoride-containing BGs can direct cell differentiation (Gentleman et al., 2013). In the present study, fluoride concentrations in the culture medium were between 3 and 6 mM (corresponding to 60–100 ppm), indicating that lower fluoride concentrations in the glass component (and subsequently in the GIC) may be advantageous.
The GICs studied here also released magnesium ions, and concentrations in undiluted cell culture medium ranged from about 8 to 10 mM. Magnesium ions present in and released from BGs have been shown to reduce apatite precipitation (Diba et al., 2012; Blochberger et al., 2015), but some beneficial effects on osteoblast proliferation, differentiation, and ALP activity have been suggested (Hoppe et al., 2011).
Taken together, our results show that it is possible to prepare mechanically and hydrolytically stable GICs from BGs free of aluminum or zinc ions, which allow for the release of reportedly therapeutic ions. Further experiments are necessary, however, to investigate if the release of fluoride, strontium, silicon, or magnesium ions from GIC does indeed have comparable beneficial effects as those demonstrated in previous studies on BG.
Conflict of Interest Statement
The authors declare that the research was conducted in the absence of any commercial or financial relationships that could be construed as a potential conflict of interest.
Acknowledgments
The authors wish to thank Mrs. Brunhilde Dreßler, Institute of Geography, University Jena, for her help and support with ICP-OES measurements and Dr. Christian Bocker, Otto Schott Institute, for EDX analyses. PVPA–PAA solution was kindly provided by Ade Akinmade, First Scientific Dental Materials, Elmshorn, Germany. DB gratefully acknowledges funding from the Carl Zeiss Foundation, Germany. EG acknowledges funding from the Wellcome Trust, UK, for a Research Career Development Fellowship.
References
Aaseth, J., Shimshi, M., Gabrilove, J. L., and Birketvedt, G. S. (2004). Fluoride: a toxic or therapeutic agent in the treatment of osteoporosis? J. Trace Elem. Exp. Med. 17, 83–92. doi: 10.1002/jtra.10051
Agency for Healthcare Research and Quality. (2010). Department of Research & Scientific Affairs, American Academy of Orthopaedic Surgeons. Rosemont, IL: Based on data from the HCUP Nationwide Inpatient Sample.
Autefage, H., Gentleman, E., Littmann, E., Hedegaard, M. A. B., von Erlach, T., O’Donnell, M., et al. (2015). Sparse feature selection methods identify unexpected global cellular response to strontium-containing materials. Proc. Natl. Acad. Sci. U.S.A. 112, 4280–4285. doi:10.1073/pnas.1419799112
Bingel, L., Groh, D., Karpukhina, N., and Brauer, D. S. (2015). Influence of dissolution medium pH on ion release and apatite formation of Bioglass® 45S5. Mater. Lett. 143, 279–282. doi:10.1016/j.matlet.2014.12.124
Blades, M. C., Moore, D. P., Revell, P. A., and Hill, R. (1998). In vivo skeletal response and biomechanical assessment of two novel polyalkenoate cements following femoral implantation in the female New Zealand white rabbit. J. Mater. Sci. Mater. Med. 9, 701–706.
Blochberger, M., Hupa, L., and Brauer, D. S. (2015). Influence of zinc and magnesium substitution on ion release from Bioglass® 45S5 at physiological and acidic pH. Biomed. Glasses 1, 93–107. doi:10.1515/bglass-2015-0009
Bonnelye, E., Chabadel, A., Saltel, F., and Jurdic, P. (2008). Dual effect of strontium ranelate: stimulation of osteoblast differentiation and inhibition of osteoclast formation and resorption in vitro. Bone 42, 129–138. doi:10.1016/j.bone.2007.08.043
Boyce, B. F., Elder, H. Y., Elliot, H. L., Fogelman, I., Fell, G. S., Junor, B. J., et al. (1982). Hypercalcemic osteomalacia due to aluminum toxicity. Lancet 2, 1009–1013. doi:10.1016/S0140-6736(82)90049-6
Brauer, D. S. (2015). Bioactive glasses – structure and properties. Angew. Chem. Int. Ed. Engl. 54, 4160–4181. doi:10.1002/anie.201405310
Brauer, D. S., Gentleman, E., Farrar, D. F., Stevens, M. M., and Hill, R. G. (2011a). Benefits and drawbacks of zinc in glass ionomer bone cements. Biomed. Mater. 6, 045007. doi:10.1088/1748-6041/6/4/045007
Brauer, D. S., Mneimne, M., and Hill, R. G. (2011b). Fluoride-containing bioactive glasses: fluoride loss during melting and ion release in tris buffer solution. J. Non Cryst. Solids 357, 3328–3333. doi:10.1016/j.jnoncrysol.2011.05.031
Brauer, D. S., Karpukhina, N., Kedia, G., Bhat, A., Law, R. V., Radecka, I., et al. (2013). Bactericidal strontium-releasing injectable bone cements based on bioactive glasses. J. R. Soc. Interface 10, 20120647. doi:10.1098/rsif.2012.0647
Cournot-Witmer, G., Zingraff, J., Plachot, J. J., Escaig, F., Lefèvre, R., Boumati, P., et al. (1981). Aluminum localization in bone from hemodialyzed patients – relationship to matrix mineralization. Kidney Int. 20, 375–385. doi:10.1038/ki.1981.149
Dabsie, F., Gregoire, G., Sixou, M., and Sharrock, P. (2009). Does strontium play a role in the cariostatic activity of glass ionomer? Strontium diffusion and antibacterial activity. J. Dent. 37, 554–559. doi:10.1016/j.jdent.2009.03.013
Darling, M., and Hill, R. (1994). Novel polyalkenoate (glass-ionomer) dental cements based on zinc silicate-glasses. Biomaterials 15, 299–306. doi:10.1016/0142-9612(94)90055-8
Diba, M., Tapia, F., Boccaccini, A. R., and Strobel, L. A. (2012). Magnesium-containing bioactive glasses for biomedical applications. Int. J. Appl. Glass Sci. 3, 221–253. doi:10.1111/j.2041-1294.2012.00095.x
Dietzel, A. (1941). Structural chemistry of glass. Naturwissenschaften 29, 537–547. doi:10.1007/BF01513796
Du, J. C., and Xiang, Y. (2012). Effect of strontium substitution on the structure, ionic diffusion and dynamic properties of 45S5 bioactive glasses. J. Non Cryst. Solids 358, 1059–1071. doi:10.1016/j.jnoncrysol.2011.12.114
Ewoldsen, N., and Demke, R. S. (2001). A review of orthodontic cements and adhesives. Am. J. Orthod. Dentofacial Orthop. 120, 45–48. doi:10.1067/mod.2001.117207
Featherstone, J. D. B. (2000). The science and practice of caries prevention. J. Am. Dent. Assoc. 131, 887–899. doi:10.14219/jada.archive.2000.0307
Fernandes Gomes, M., Pinheiro de Abreu, P., Cantarelli Morosolli, A. R., Marotta Araujo, M., and das Gracas Vilela Goulart, M. (2006). Densitometric analysis of the autogenous demineralized dentin matrix on the dental socket wound healing process in humans. Braz. Oral Res. 20, 324–330.
Fredholm, Y. C., Karpukhina, N., Brauer, D. S., Jones, J. R., Law, R. V., and Hill, R. G. (2012). Influence of strontium for calcium substitution in bioactive glasses on degradation, ion release and apatite formation. J. R. Soc. Interface 9, 880–889. doi:10.1098/rsif.2011.0387
Fredholm, Y. C., Karpukhina, N., Law, R. V., and Hill, R. G. (2010). Strontium containing bioactive glasses: glass structure and physical properties. J. Non Cryst. Solids 356, 2546–2551. doi:10.1016/j.jnoncrysol.2010.06.078
Gentleman, E., Fredholm, Y. C., Jell, G., Lotfibakhshaiesh, N., O’Donnell, M. D., Hill, R. G., et al. (2010). The effects of strontium-substituted bioactive glasses on osteoblasts and osteoclasts in vitro. Biomaterials 31, 3244–3252. doi:10.1016/j.biomaterials.2010.01.121
Gentleman, E., Stevens, M. M., Hill, R. G., and Brauer, D. S. (2013). Surface properties and ion release from fluoride-containing bioactive glasses promote osteoblast differentiation and mineralization in vitro. Acta Biomater. 9, 5771–5779. doi:10.1016/j.actbio.2012.10.043
Griffin, S., and Hill, R. (1998). Influence of poly(acrylic acid) molar mass on the fracture properties of glass polyalkenoate cements. J. Sci. Mater. 33, 5383–5396. doi:10.1023/A:1004498217028
Griffin, S. G., and Hill, R. G. (1999). Influence of glass composition on the properties of glass polyalkenoate cements. Part I: influence of aluminium to silicon ratio. Biomaterials 20, 1579–1586. doi:10.1016/S0142-9612(99)00058-7
Guida, A., Towler, M. R., Wall, J. G., Hill, R. G., and Eramo, S. (2003). Preliminary work on the antibacterial effect of strontium in glass ionomer cements. J. Mater. Sci. Lett. 22, 1401–1403. doi:10.1023/A:1025794927195
Hernlund, E., Svedbom, A., Ivergard, M., Compston, J., Cooper, C., Stenmark, J., et al. (2013). Osteoporosis in the European Union: medical management, epidemiology and economic burden. A report prepared in collaboration with the international osteoporosis foundation (IOF) and the European federation of pharmaceutical industry associations (EFPIA). Arch. Osteoporos. 8, 136. doi:10.1007/s11657-013-0136-1
Hill, R. G., Wilson, A. D., and Warrens, C. P. (1989). The influence of poly(acrylic acid) molecular weight on the fracture toughness of glass-ionomer cements. J. Sci. Mater. 24, 363–371. doi:10.1007/BF00660982
Hoppe, A., Güldal, N. S., and Boccaccini, A. R. (2011). A review of the biological response to ionic dissolution products from bioactive glasses and glass-ceramics. Biomaterials 32, 2757–2774. doi:10.1016/j.biomaterials.2011.01.004
Hurrell-Gillingham, K., Reaney, I. M., Brook, I., and Hatton, P. V. (2006). In vitro biocompatibility of a novel Fe2O3 based glass ionomer cement. J. Dent. 34, 533–538. doi:10.1016/j.jdent.2005.07.011
Jasper, L. E., Deramond, H., Mathis, J. M., and Belkoff, S. M. (2002). Material properties of various cements for use with vertebroplasty. J. Mater. Sci. Mater. Med. 13, 1–5. doi:10.1023/A:1013170610826
Jones, J. R. (2013). Review of bioactive glass: from Hench to hybrids. Acta Biomater. 9, 4457–4486. doi:10.1016/j.actbio.2012.08.023
Joshi, J. G. (1990). Aluminum, a neurotoxin which affects diverse metabolic reactions. Biofactors 2, 163–169.
Lewis, G. (2006). Injectable bone cements for use in vertebroplasty and kyphoplasty: state-of-the-art review. J. Biomed. Mater. Res. Part B Appl. Biomater. 76B, 456–468. doi:10.1002/jbm.b.30398
Lotfibakhshaiesh, N., Brauer, D. S., and Hill, R. G. (2010). Bioactive glass engineered coatings for Ti6Al4V alloys: influence of strontium substitution for calcium on sintering behaviour. J. Non Cryst. Solids 356, 2583–2590. doi:10.1016/j.jnoncrysol.2010.05.017
Marie, P. J. (2005). Strontium as therapy for osteoporosis. Curr. Opin. Pharmacol. 5, 633–636. doi:10.1016/j.coph.2005.05.005
Marie, P. J., Ammann, P., Boivin, G., and Rey, C. (2001). Mechanisms of action and therapeutic potential of strontium in bone. Calcif. Tissue Int. 69, 121–129. doi:10.1007/s002230010055
Martin, R. A., Twyman, H. L., Rees, G. J., Barney, E. R., Moss, R. M., Smith, J. M., et al. (2012). An examination of the calcium and strontium site distribution in bioactive glasses through isomorphic neutron diffraction, X-ray diffraction, EXAFS and multinuclear solid state NMR. J. Mater. Chem. 22, 22212–22223. doi:10.1039/c2jm33058j
Neuville, D. R., Cormier, L., and Massiot, D. (2006). Al coordination and speciation in calcium aluminosilicate glasses: effects of composition determined by Al-27 MQ-MAS NMR and Raman spectroscopy. Chem. Geol. 229, 173–185. doi:10.1016/j.chemgeo.2006.01.019
Reid, I. R., Cundy, T., Grey, A. B., Horne, A., Clearwater, J., Ames, R., et al. (2007). Addition of monofluorophosphate to estrogen therapy in postmenopausal osteoporosis: a randomized controlled trial. J. Clin. Endocrinol. Metab. 92, 2446–2452. doi:10.1210/jc.2006-2264
Schumacher, M., and Gelinsky, M. (2015). Strontium modified calcium phosphate cements – approaches towards targeted stimulation of bone turnover. J. Mater. Chem. B 3, 4626–4640. doi:10.1039/C5TB00654F
Shannon, R. D. (1976). Revised effective ionic radii and systematic studies of interatomic distances in halides and chalcogenides. Acta Crystallogr. Sect. A A32, 751–767. doi:10.1107/S0567739476001551
Socrates, G. (2004). Infrared and Raman Characteristic Group Frequencies. Tables and Charts. Chichester, NY: John Wiley & Sons.
Stamboulis, A., Law, R. V., and Hill, R. G. (2004). Characterisation of commercial ionomer glasses using magic angle nuclear magnetic resonance (MAS-NMR). Biomaterials 25, 3907–3913. doi:10.1016/j.biomaterials.2003.10.074
Thuy, T. T., Nakagaki, H., Kato, K., Phan, A. H., Inukai, J., Tsuboi, S., et al. (2008). Effect of strontium in combination with fluoride on enamel remineralization in vitro. Arch. Oral Biol. 53, 1017–1022. doi:10.1016/j.archoralbio.2008.06.005
Watts, S. J., O’Donnell, M. D., Law, R. V., and Hill, R. G. (2010). Influence of magnesia on the structure and properties of bioactive glasses. J. Non Cryst. Solids 356, 517–524. doi:10.1016/j.jnoncrysol.2009.04.074
Wilson, A. D. (1996). A hard decade’s work: steps in the invention of the glass-ionomer cement. J. Dent. Res. 75, 1723–1727. doi:10.1177/00220345960750100301
Wilson, A. D., Prosser, H. J., and Powis, D. M. (1983). Mechanism of adhesion of poly-electrolyte cements to hydroxyapatite. J. Dent. Res. 62, 590–592. doi:10.1177/00220345830620051801
Xynos, I. D., Edgar, A. J., Buttery, L. D. K., Hench, L. L., and Polak, J. M. (2000). Ionic products of bioactive glass dissolution increase proliferation of human osteoblasts and induce insulin-like growth factor II mRNA expression and protein synthesis. Biochem. Biophys. Res. Commun. 276, 461–465. doi:10.1006/bbrc.2000.3503
Keywords: bone cement, strontium, compressive strength, bioactive glass, bone filler
Citation: Fuchs M, Gentleman E, Shahid S, Hill RG and Brauer DS (2015) Therapeutic ion-releasing bioactive glass ionomer cements with improved mechanical strength and radiopacity. Front. Mater. 2:63. doi: 10.3389/fmats.2015.00063
Received: 27 July 2015; Accepted: 18 September 2015;
Published: 14 October 2015
Edited by:
Wolfram Höland, Ivoclar Vivadent AG, LiechtensteinReviewed by:
Ahmed El-Fiqi, Dankook University, South KoreaJin Nakamura, National Institute for Materials Science, Japan
Copyright: © 2015 Fuchs, Gentleman, Shahid, Hill and Brauer. This is an open-access article distributed under the terms of the Creative Commons Attribution License (CC BY). The use, distribution or reproduction in other forums is permitted, provided the original author(s) or licensor are credited and that the original publication in this journal is cited, in accordance with accepted academic practice. No use, distribution or reproduction is permitted which does not comply with these terms.
*Correspondence: Delia S. Brauer, Otto Schott Institute of Materials Research, Friedrich Schiller University Jena, Fraunhoferstr. 6, Jena 07743, Germany, delia.brauer@uni-jena.de