- 1Science and Technology Division, Corning Incorporated, Corning, NY, USA
- 2Argonne National Laboratory, Center for Nanoscale Materials, Lemont, IL, USA
Lithium aluminosilicate glass-ceramics have found widespread commercial success in areas such as consumer products, telescope mirrors, fireplace windows, etc. However, there is still much to learn regarding the fundamental mechanisms of crystallization, especially related to the evolution of viscosity as a function of the crystallization (ceramming) process. In this study, the impact of phase assemblage and microstructure on the viscosity was investigated using high-temperature X-ray diffraction (HTXRD), beam bending viscometry (BBV), and transmission electron microscopy (TEM). Results from this study provide a first direct observation of viscosity evolution as a function of ceramming time and temperature. Sharp viscosity increases due to phase separation, nucleation, and phase transformation are noticed through BBV measurement. A near-net shape ceramming can be achieved in TiO2-containing compositions by keeping the glass at a high viscosity (>109 Pa s) throughout the whole thermal treatment.
Introduction
There have been increasing uses of glass-ceramics in areas such as consumer, biomedical, and construction markets since their discovery by Stookey in 1950s (Stookey, 1960a,b; Holand and Beall, 2002; Zanotto, 2010). Lithium aluminosilicate glass-ceramics have gained considerable commercial success due to the combination of low thermal expansion [coefficient of thermal expansion (CTE), close to 0 × 10−7/°C] and high mechanical strength attributed to its two main crystalline phases, β-quartz and β-spodumene (keatite) solid solutions (ss) (Ostertag et al., 1968; Holand and Beall, 2002; Dressler et al., 2011a). Both ZrO2 and TiO2 are commonly used as nucleation agents to produce controlled bulk crystallization in the precursor glass (Henderson, 1979; Holand and Beall, 2002; Zanotto, 2010). Although small in concentration, nucleation agents lead to the formation of nuclei for crystal growth (Holand and Beall, 2002). Fine-grained microstructures composed of small crystals (0.1–1.0 μm) uniformly distributed in a residual glassy phase are usually observed in lithium aluminosilicate glass-ceramics, which is important to achieve a desired mechanical strength (Beall and Doman, 1987; Beall and Pinckney, 1999; Guedes et al., 2001; Holand and Beall, 2002).
The crystallization process and its associated microstructural evolution are generally studied by differential scanning calorimetry (DSC), X-ray diffraction (XRD), scanning electron microscopy (SEM), or transmission electron microscopy (TEM). Thermal analysis serves as a rapid and convenient technique for the study of the kinetics of chemical reactions and crystallization of glass (Matusita and Sakka, 1980). It has been used to study the crystallization kinetics of various glass systems including the lithium aluminosilicate (Ray and Day, 1990; Barbieri et al., 1997a; Kim et al., 2004; Fernandes et al., 2008; Wang et al., 2010; Lilensten et al., 2014). Recently, high-temperature X-ray diffraction (HTXRD) and anomalous small-angle X-ray scattering (ASAXS) have been utilized for the in situ observation of the phase transition and structural changes during high-temperature heat treatment (Misture, 2003; Holand et al., 2006; Sinton et al., 2008; Dressler et al., 2011b; Bocker et al., 2013; Lilensten et al., 2014; Raghuwanshi et al., 2014; Kleebusch et al., 2016). Despite the comprehensive understanding of the crystallization mechanism and microstructural changes, the viscosity evolution during the crystallization process remained unclear. However, the control of glass viscosity is critical to achieve a near-net-shape ceramming in the manufacturing of glass-ceramic products (Holand and Beall, 2011).
The objective of this work is to investigate the crystallization, microstructure, and viscosity evolutions in lithium aluminosilicate glass. Both HTXRD and TEM were used to characterize structural evolution in the glass-ceramics during ceramming, while beam bending viscometry (BBV) was used to measure the changes in glass viscosity.
Experimental
Base glass composition (wt%) of 67 SiO2, 25 Al2O3, 5.0 Li2O, 1.5 MgO, and 1.5 ZnO was prepared by melting a mixture of analytical grade raw materials in platinum crucibles at 1600°C. TiO2 (0–10 wt%) was added on top of the base glass composition to investigate the glass stability with different nucleation agent levels. Table 1 listed the glass compositions for this work. Glasses were poured onto a steel plate to form into patties and then annealed at 600°C to remove thermal stress.
Crystalline phases formed in the annealed glasses were detected using XRD. Data were collected from 5° to 80° (2θ) using a Bruker D4 Endeavor equipped with a LynxEye™ (Bruker Corporation, Billerica, MA, USA) silicon strip detector. The XRD pattern was analyzed using the PDF-4 database and Jade.
Differential scanning calorimetry was used to determine the non-isothermal kinetic parameters for crystallization. About 40 mg of fine glass powders (1–10 μm) was used for the DSC analysis, which was performed with a Netzsch DSC 404 F1 Pegasus. The powders were contained in a platinum cup and heated at 10°C/min.
High-temperature XRD was used as an isothermal technique to determine the kinetic parameters for crystallization. In situ HTXRD study was carried out on a PANalytical MPD XRD system equipped with an Anton Paar HTK1200N high-temperature furnace and an X’Celerator multiple strip detector. A polished glass disk (15 mm in diameter × 1 mm thick) was used for the analysis. A rapid heating rate, 20°C/min, from RT to crystallization temperature was followed by continuous data collection at an isothermal hold temperature. XRD diffraction traces in the 2θ range of 10–70° were collected every 10 min.
The viscosity–temperature behavior of glass was analyzed to investigate the viscosity evolution during ceramming. Glass viscosity below the softening point, in the range of 108–1013 Pa s, was measured using BBV using a three-point bend, BBV-1000 beam bending viscometer (Orton Ceramics, Westerville, OH, USA) according to the American Society for Testing and Materials (ASTM) standard (ASTM International, 2013). Briefly, a load was applied on a glass beam (2.5 mm × 2.5 mm × 55 mm) in a three-point beam bending. To avoid over deformation due to low viscosity in the nucleation stage, the measurement of glass viscosity in the crystal growth stage was conducted on beams pre-nucleated at 750°C for 2 h.
High-resolution scanning transmission electron microscopy (HRSTEM) was used to observe the detailed crystal morphology and to determine the elemental partitioning in the cerammed samples using energy dispersive X-ray (EDX) elemental mapping. Electron-transparent thin sections of less than 100 nm were prepared using focused ion beam (FIB) and observed under TEM with 200-kV accelerating voltage.
Results
Green (Non-Cerammed) Glass
The addition of TiO2 has a substantial impact on the glass stability. When TiO2 concentrations were below 7.0 wt%, no phase separation or devitrification was observed in glass. However, when more TiO2 (7.0 wt% or above) was added to the base glass, phase separation (composition #6) and significant devitrification (composition #7) were observed in the annealed glasses. XRD analysis in Figure 1 confirmed the amorphous nature of compositions #1–5. In contrast, a small amount of β-quartz ss was detected in composition #6, while a completely devitrified sample was obtained in #7. Compositions #1 (no TiO2) and #5 (5.0 wt% TiO2) with no phase separation or devitrification were selected for further studies in this work.
Thermal Analysis
Figure 2 shows the DSC analysis of the two compositions (#1 and 5) of interest. The addition of TiO2 to the lithium aluminosilicate glass results in pronounced exothermal reactions in DSC while none in TiO2-free glass. No detectable exothermal peaks were observed in TiO2-free glass (#1) below 950°C, while three exothermal peaks were observed in TiO2-containing glass (#5), indicative of the marked crystallization due to addition of a nucleation agent.
Phase Assemblage
Figure 3 shows the phase assemblage for two glasses at different thermal treatment steps. Based on the DSC analysis, both glasses were first heated at 750°C for 2 h for nucleation and then at 1050°C for 4 h for crystal growth. No crystalline phases were detected in TiO2-free glass (composition #1) at the nucleation step (Figure 3A), while β-quartz ss was determined in composition #5 added with 5 mol% TiO2 (Figure 3C), in good agreement with the DSC results. The concentration of β-quartz ss increased with increasing holding time at 750°C for composition #5 (Figure 3C). A fully crystallized body was obtained in both glass compositions after the crystal growth stage (1050°C for 4 h). For composition #1, the conversion from β-quartz ss to β-spodumene ss did not complete until after 2 h hold at 1050°C (Figure 3B), while in TiO2-containing composition #5, the conversion completed after 0.5 h (Figure 3D). The major phase was β-spodumene ss for the two compositions, while a small amount of tieilite (Al2O3⋅TiO2) was detected in composition #5. It is worth noting that a high number of cracks were formed in composition #1 due to the lack of volume crystallization, while a crack-free part was achieved in composition #5.
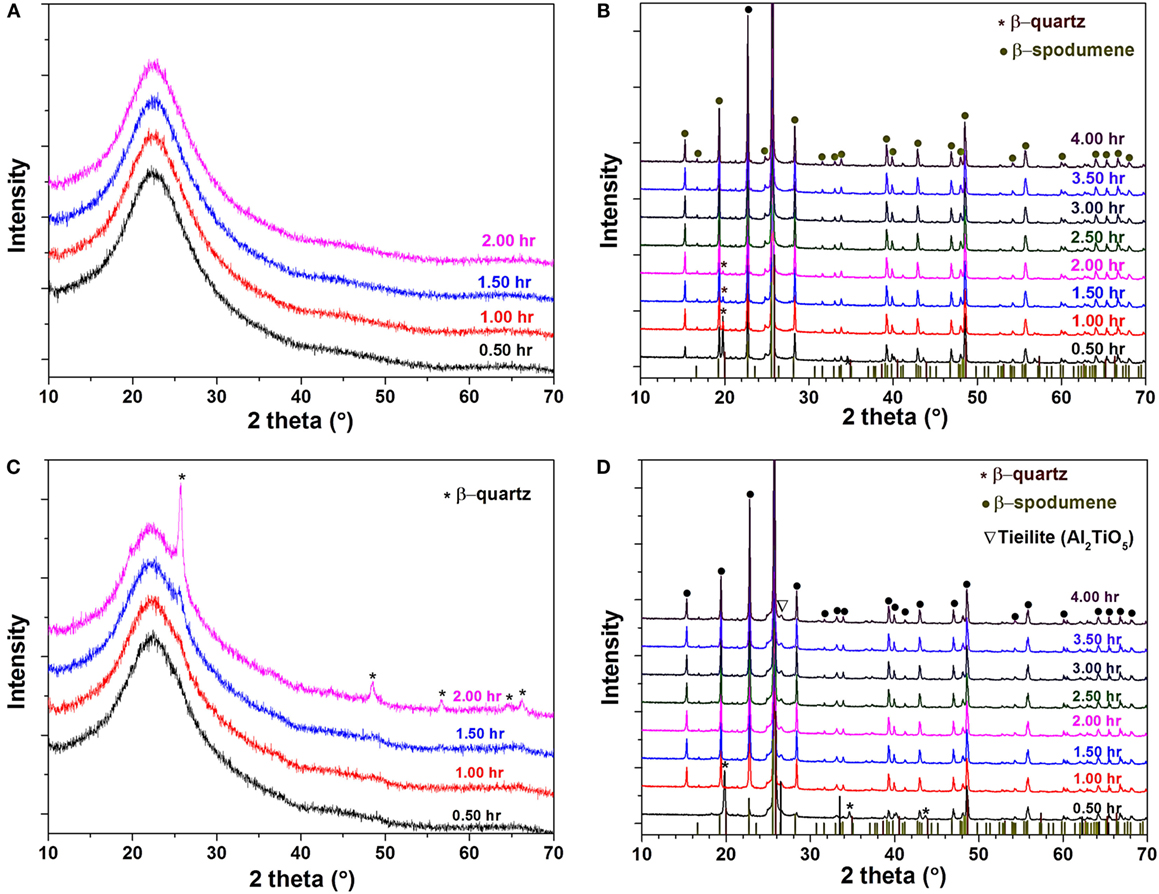
Figure 3. High-temperature X-ray diffraction patterns of glass composition #1: (A) after nucleation at 750°C for 0.5–2.0 h and (B) after crystal growth at 1050°C for 0.5–4.0 h; and of composition #5: (C) after nucleation at 750°C for 0.5–2.0 h and (D) after crystal growth at 1050°C for 0.5–4.0 h.
Microstructure
Transmission electron microscopy images in Figure 4 show the microstructural changes of composition #5 during the nucleation hold at 750°C. For the green glass, no clear feature was observed even at high magnification (Figure 4A). After heat treatment at 750°C for 1 h, droplet-like phase was observed in the sample (Figure 4B). The size of the droplets was in the range of 5–10 nm. At a low magnification, β-quartz ss phase (50–80 nm) was observed to form in the glass matrix (Figure 4C), which agrees with the XRD results. A higher density of β-quartz grains was visible after holding at 750°C for 4 h (Figure 4D). The size of the crystal grew slightly falling in the range of 50–100 nm.
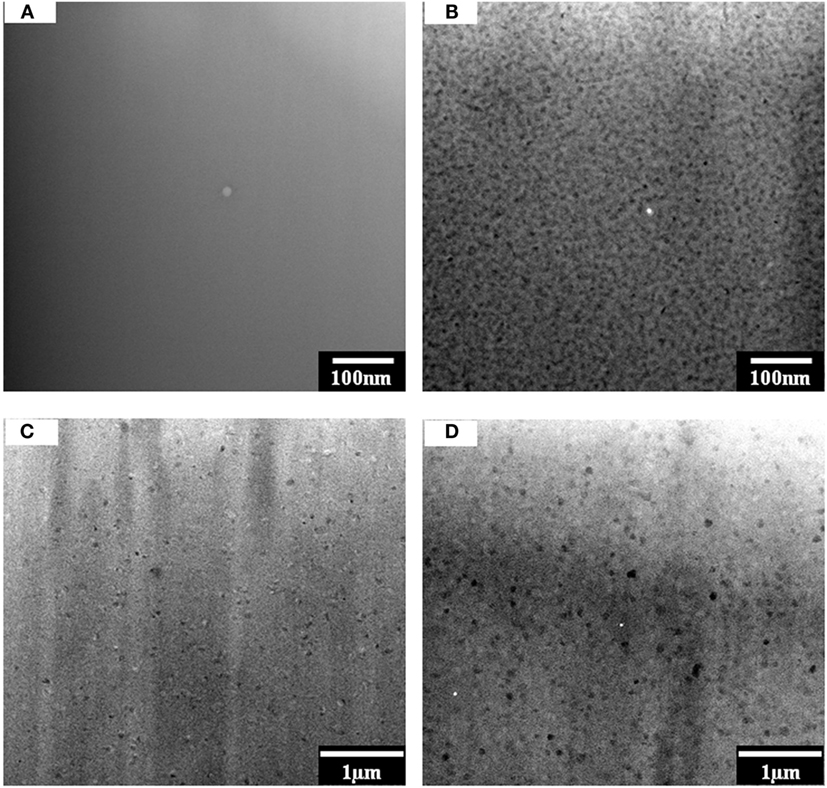
Figure 4. TEM of nucleated glass composition #5: (A) green glass; (B) after holding at 750°C for 1.0 h showing phase separation; (C) low magnification showing the formation of β-quartz ss after at 750°C for 1.0 h; (D) more β-quartz ss after holding at 750°C for 4.0 h.
A highly crystallized body composed of large grains, and small droplet-like crystals was observed after final heat treatment at 1050°C for 4 h (Figure 5A). Combined with XRD results (Figure 3D), the large grains were determined to be β-spodumene ss and small droplets tieilite. The size of β-spodumene grains was in the range of 0.3–1.0 μm while tieilite 30–50 nm (Figure 5B). Elemental mapping confirmed that the droplets were rich in Al and Ti (Figures 5C,D).
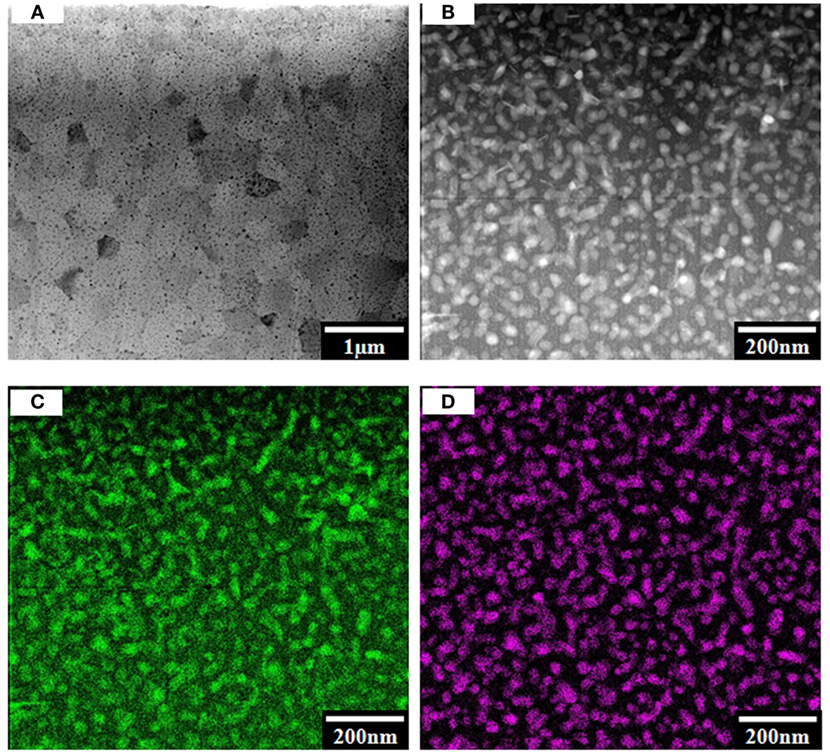
Figure 5. TEM images showing microstructure and elemental mapping of composition #5 after holding at 1050°C for 4.0 h: (A) overview of microstructure; (B) high-magnification image showing the fine crystals; (C) Al map of (B); (D) Ti map of (B).
Viscosity Evolution
Figure 6 shows the viscosity evolution for the two selected glasses as a function of ceramming cycle. For composition #1 without nucleation agent, the viscosity decreased monotonically with increasing temperatures, consistent with most glass compositions. The measurement was stopped at 875°C due to its low viscosity, close to 108 Pa s, which was below the measurement limit for the BBV method. The viscosity remained almost unchanged during the holding at 750°C, corresponding well with the HTXRD study which shows little to no structural change in this glass (Figure 3A). At the end of ceramming cycle, the glass cracked into pieces due to the lack of effective bulk nucleation.
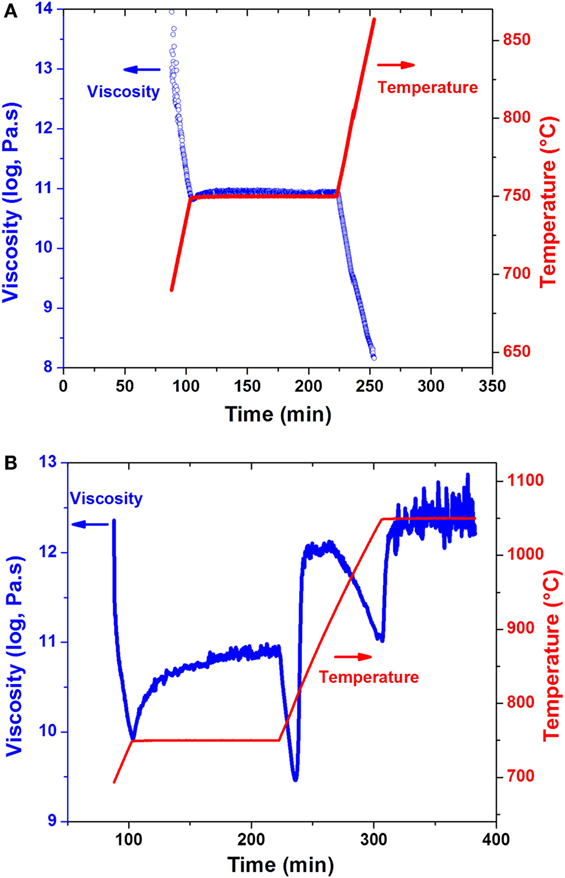
Figure 6. Viscosity–time–temperature plots of glass compositions (A) #1 and (B) #5 as a function of heat treatment.
In contrast, the change of viscosity for the TiO2-containing glass (composition #5) as a function of ceramming temperature was much more complicated than the monotonic decrease of viscosity in TiO2-free glass (Figure 6B). The viscosity plot could be divided into three stages. At stage I, an increase of one order of magnitude in viscosity was observed during the hold at 750°C, suggesting either phase separation or nucleation at this stage. At stage II, an abrupt decrease of viscosity with increasing temperature was observed after the initial nucleation hold until 824°C, at which temperature a sharp increase in viscosity was noticed. The temperature for the jump in viscosity was exactly the onset temperature for the largest exothermal peak in DSC (Figure 2), which was confirmed by XRD to be the formation of β-quartz (Figure 3C). At this stage, the major phase in composition #5 was β-quartz ss with very little amount of glassy phase, suggesting a high viscosity during ceramming. Once the reaction completed, the viscosity of composition #5 started to decrease with increasing temperature due to the lack of new crystalline phase formation and the relatively stable structure. At stage III, β-quartz ss was converted to β-spodumene ss and no further structural change during the holding at 1050°C, as evidenced by the small variation in viscosity and the unchanged phase assemblage in HTXRD (Figure 3D). Overall, the viscosity of the TiO2-containing glass was kept above 109.5 Pa s during the whole ceramming cycle, which contributed to a near-net-shape ceramming of composition #5 without cracking or visible deformation.
Discussion
Numerous investigations have been conducted to understand the crystallization process in Li2O–Al2O3–SiO2 glass-ceramic family due to their increased commercial applications. Nucleation agents, either single or a combination of several, including TiO2, ZrO2, P2O5, and Ta2O5 have been used to produce a bulk nucleation owing to a liquid–liquid phase separation (Barbieri et al., 1997b; Guedes et al., 2001; Wurth et al., 2009; Ananthanarayanan et al., 2010; Li et al., 2010; Nemati et al., 2010; Höche et al., 2011; Höche et al., 2012; Montedo et al., 2012; Chavoutier et al., 2014; Kleebusch et al., 2016; Soares and Zanotto, 2016). Although minor in concentration, nucleation agents are critical to the final microstructure and properties of glass-ceramics. This work studies the crystallization, and microstructural and viscosity evolutions in Li2O–Al2O3–SiO2 glass. It is found that TiO2 impacts the properties of both green glass and glass-ceramic. In green glass, a higher concentration of TiO2 results in a high tendency for devitrification and a less thermal stability (Figures 1 and 2). Therefore, the level of TiO2 must be controlled within a proper range to obtain a stable glass without devitrification for a glass–ceramic composition.
Both the phase assemblage and microstructure of lithium aluminosilicate glass-ceramics are influenced by the presence of TiO2. When a proper amount of TiO2 was added to the Li2O–Al2O3–SiO2 glass, phase separation was developed at the nucleation stage, which led to the formation of β-quartz ss (Figures 3 and 4). This is consistent with previous reports that the addition of TiO2 produced a TiO2-rich phase and serves as a nucleation phase for the formation of β-quartz ss (Doherty et al., 1967; Barbieri et al., 1997b,c; Nordmann and Cheng, 1997; Bhattacharyya et al., 2009). In contract, the TiO2-free glass remained amorphous at this nucleation stage (Figure 3).
The viscosity of the system exhibits a strong dependence on the phase assemblage and structure of each composition. The monotonic decrease of viscosity as a function of ceramming cycle for TiO2-free composition #1 agrees well with the HTXRD and DSC analysis, both of which show no evidence of new phase formation within the tested temperature range. In contract, three distinct stages were observed in the viscosity of TiO2-containing composition #5 (Figure 6), corresponding well with the thermal events determined by DSC (Figure 2), phase assemblage evolution by HTXRD (Figure 3), and microstructural change by TEM (Figures 4 and 5). At stage I, the increasing viscosity at the nucleation hold was mainly attributed to β-quartz ss formation; at stage II, the abrupt increase in viscosity was a direct consequence of the formation of high concentration of β-quartz ss phase; and at stage III, the conversion β-quartz to β-spodumene ss was completed, which produced a fully crystallized glass-ceramic with little glassy phase and a high-viscosity cerammed body. The increase of viscosity due to crystallization was also observed in a lithium aluminosilicate glass containing ZrO2 and TiO2 as nucleation agents. A higher Tg was reported in the nucleated glass than in the precursor glass due to the presence of β-quartz phase (Wurth et al., 2009). The formation of an alumina-rich layer surrounding a ZrTiO4-rich core was reported to account for the increase of viscosity (Raghuwanshi et al., 2014). However, no direct analysis of the viscosity evolution in this system has been conducted. The increase in glass viscosity due to the nucleation in TiO2-containing lithium aluminosilicate glass has been investigated in a previous study using a fiber elongation method, although the measurement was done on a post-treated sample not an in situ measurement during ceramming (Kim et al., 2004). The viscosity of the nucleated composite was decided by the concentration of the crystalline phase and the residual glass composition. The relationship between viscosity and the presence of the crystalline phase was described using the following equation (Kim et al., 2004):
where ηapp is the apparent viscosity of the crystallized glass, ηg the viscosity of the precursor glass, and α the volume fraction of crystalline phase. Based on Eq. 1, a higher concentration of β-quartz ss phase led to a high viscosity in the crystallized glass, which was in agreement with the TEM observation and viscosity measurement (Figures 4 and 6). Additionally, with the formation of β-quartz ss (Li2O⋅Al2O3⋅4SiO2), a highly siliceous residual glass was developed, which increased the viscosity of the system. The finding in our in situ viscosity measurement at the nucleation step was consistent with the previous reports. Our in situ measurement throughout the full ceramming cycle established the viscosity–temperature–time relationship for the first time.
Conclusion
An investigation on the evolutions of crystallization, microstructure, and viscosity in lithium aluminosilicate glass was conducted in this work. The addition of a nucleation agent (TiO2) was found to have a marked impact on the properties in both the glass and glass-ceramics. At the nucleation stage, phase separation and formation of β-quartz ss phase were observed, resulting in an increase of one order of magnitude in viscosity in TiO2-containing glass. In contrast, the viscosity in TiO2-free glass showed a monotonic decrease in viscosity when cerammed using the same cycle. Further viscosity variation due to crystallization and crystal growth was also observed in TiO2-containing glass. The glass viscosity demonstrated a strong dependence on the phase assemblage and structural changes during ceramming as supported by HTXRD, DSC, and TEM analysis. Last but not the least, the addition of TiO2 helped achieve a near-net shape ceramming due to the high glass viscosity throughout the whole thermal treatment, which was critical for the manufacturing of products with complicated three-dimensional shapes.
Author Contributions
All authors conducted experiments and contributed to the writing of the draft.
Conflict of Interest Statement
The authors declare that the research was conducted in the absence of any commercial or financial relationships that could be construed as a potential conflict of interest.
References
Ananthanarayanan, A., Kothiyal, G. P., Montagne, L., and Revel, B. (2010). MAS-NMR studies of lithium aluminum silicate (LAS) glasses and glass-ceramics having different Li2O/Al2O3 ratio. J. Solid State Chem. 183, 120–127. doi: 10.1016/j.jssc.2009.10.006
ASTM International. (2013). “Standard test method for annealing point and strain point of glass by beam bending,” in ASTM C598-93 (West Conshohocken, PA).
Barbieri, L., Leonelli, C., Manfredini, T., Siligardi, C., and Corradi, A. B. (1997a). Nucleation and crystallization of a lithium aluminosilicate glass. J. Am. Ceram. Soc. 80, 3077–3083. doi:10.1111/j.1151-2916.1997.tb03235.x
Barbieri, L., Corradi, A. B., Leonelli, C., Siligardi, C., Manfredini, T., and Pellacani, G. C. (1997b). Effect of TiO2 addition on the properties of complex aluminosilicate glasses and glass-ceramics. Mater. Res. Bull. 32, 637–648. doi:10.1016/S0025-5408(97)00029-9
Barbieri, L., Leonelli, C., Manfredini, T., Siligardi, C., Corradi, A. B., Mustarelli, P., et al. (1997c). Nucleation and crystallization of a lithium aluminosilicate glass. J. Am. Ceram. Soc. 80, 3077–3083. doi:10.1111/j.1151-2916.1997.tb03235.x
Beall, G. H., and Doman, R. C. (1987). “Glass-ceramics,” in Encyclopedia of Physical Science and Technology, ed. R. A. Meyer. (New York: Academic Press), Vol. 6, 294–307.
Beall, G. H., and Pinckney, L. R. (1999). Nanophase glass-ceramics. J. Am. Ceram. Soc. 82, 5–16. doi:10.1111/j.1151-2916.1999.tb01716.x
Bhattacharyya, S., Höche, T., Jinschek, J. R., Avramov, I., Wurth, R., Mu¨ller, M., et al. (2009). Direct evidence of Al-rich layers around nanosized ZrTiO4 in glass: putting the role of nucleation agents in perspective. Cryst. Growth Des. 10, 379–385. doi:10.1021/cg9009898
Bocker, C., Rüssel, C., and Avramov, I. (2013). Transparent nano crystalline glass-ceramics by interface controlled crystallization. Int. J. Appl. Glass Sci. 4, 174–181. doi:10.1111/ijag.12033
Chavoutier, M., Caurant, D., Majérus, O., Boulesteix, R., Loiseau, P., Jousseaume, C., et al. (2014). Effect of TiO2 content on the crystallization and the color of (ZrO2, TiO2)-doped Li2O–Al2O3–SiO2 glasses. J. Non-Cryst. Solids 384, 15–24. doi:10.1016/j.jnoncrysol.2013.03.034
Doherty, P., Lee, D., and Davis, R. (1967). Direct observation of the crystallization of Li2O-Al2O3-SiO2 glasses containing TiO2. J. Am. Ceram. Soc. 50, 77–81. doi:10.1111/j.1151-2916.1967.tb15043.x
Dressler, M., Rüdinger, B., and Deubener, J. (2011a). An in situ high-temperature X-ray diffraction study of early-stage crystallization in lithium aluminosilicate glass–ceramics. J. Am. Ceram. Soc. 94, 1421–1426. doi:10.1111/j.1551-2916.2010.04252.x
Dressler, M., Rüdinger, B., and Deubener, J. (2011b). An in situ high-temperature X-ray diffraction study of early-stage crystallization in lithium alumosilicate glass–ceramics. J. Am. Ceram. Soc. 94, 1421–1426. doi:10.1111/j.1551-2916.2010.04252.x
Fernandes, H. R., Tulyaganov, D. U., Goel, I. K., and Ferreira, J. M. F. (2008). Crystallization process and some properties of Li2O-SiO2 glass-ceramics doped with Al2O3 and K2O. J. Am. Ceram. Soc. 91, 3698–3703. doi:10.1111/j.1551-2916.2008.02724.x
Guedes, M., Ferro, A. C., and Ferreira, J. M. F. (2001). Nucleation and crystal growth in commercial LAS compositions. J. Eur. Ceram. Soc. 21, 1187–1194. doi:10.1016/S0955-2219(00)00333-2
Henderson, D. (1979). Experimental analysis of non-isothermal transformations involving nucleation and growth. J. Therm. Anal. 15, 325–331. doi:10.1007/BF01903656
Höche, T., Mäder, M., Bhattacharyya, S., Henderson, G. S., Gemming, T., Wurth, R., et al. (2011). ZrTiO4 crystallisation in nanosized liquid–liquid phase-separation droplets in glass – a quantitative XANES study. CrystEngComm 13, 2550–2556. doi:10.1039/c0ce00716a
Holand, W., Apel, E., van ‘t Hoen, C., and Rheinberger, V. (2006). Studies of crystal phase formations in high-strength lithium disilicate glass-ceramics. J. Non-Cryst. Solids 352, 4041–4050. doi:10.1016/j.jnoncrysol.2006.06.039
Holand, W., and Beall, G. (2011). Glass-Ceramic Technology, 2nd Edn. Hoboken, NJ: John Wiley & Sons, Inc.
Höche, T., Patzig, C., Gemming, T., Wurth, R., Rüssel, C., and Avramov, I. (2012). Temporal evolution of diffusion barriers surrounding ZrTiO4 nuclei in lithia aluminosilicate glass-ceramics. Cryst. Growth Des. 12, 1556–1563. doi:10.1021/cg2016148
Kim, K. D., Lee, S. H., and Ahn, H. K. (2004). Observation of nucleation effect on crystallization in lithium aluminosilicate glass by viscosity measurement. J. Non-Cryst. Solids 336, 195–201. doi:10.1016/j.jnoncrysol.2004.01.001
Kleebusch, E., Patzig, C., Höche, T., and Rüssel, C. (2016). Effect of the concentrations of nucleating agents ZrO2 and TiO2 on the crystallization of Li2O–Al2O3–SiO2 glass: an X-ray diffraction and TEM investigation. J. Sci. Mater. 51, 10127–10138. doi:10.1007/s10853-016-0241-9
Li, Y., Liang, K., Xu, B., and Cao, J. (2010). Crystallization mechanism and microstructure evolution of Li2O–Al2O3–SiO2 glass-ceramics with Ta2O5 as nucleating agent. J. Therm. Anal. Calorim. 101, 941–948. doi:10.1007/s10973-009-0598-y
Lilensten, L., Fu, Q., Wheaton, B. R., Credle, A. J., Stewart, R. L., and Kohli, J. T. (2014). Kinetic study on lithium-aluminosilicate (LAS) glass-ceramics containing MgO and ZnO. Ceram. Int. 40, 11657–11661. doi:10.1016/j.ceramint.2014.03.171
Matusita, K., and Sakka, S. (1980). Kinetic study on crystallization of glass by differential thermal analysis-criterion on application of Kissinger plot. J. Non-Cryst. Solids 38–39, 741–746. doi:10.1016/0022-3093(80)90525-6
Misture, S. T. (2003). Large-volume atmosphere-controlled high-temperature X-ray diffraction furnace. Meas. Sci. Technol. 14, 1091. doi:10.1088/0957-0233/14/7/326
Montedo, O. R. K., Hotza, D., de Oliveira, A. P. N., Meszaros, R., Travitzky, N., and Greil, P. (2012). Crystallisation kinetics of β-spodumene-based glass ceramic. Adv. Mater. Sci. Eng. 2012, 8. doi:10.1155/2012/525428
Nemati, A., Goharian, P., Shabanian, M., and Afshar, A. (2010). Effects of nucleation agent particle size on properties, crystallisation and microstructure of glass-ceramics in TiO2-ZrO2-Li2O-CaO-Al2O3-SiO2 system. Adv. Appl. Ceram. 109, 318–323. doi:10.1179/174367609X422063
Nordmann, A., and Cheng, Y.-B. (1997). Crystallization behaviour and microstructural evolution of a Li2O–Al2O3–SiO2 glass derived from spodumene mineral. J. Sci. Mater. 32, 83–89. doi:10.1023/A:1018519030791
Ostertag, W., Fischer, G. R., and Williams, J. P. (1968). Thermal expansion of synthetic β-spodumene and β-spodumene–silica solid solutions. J. Am. Ceram. Soc. 51, 651–654. doi:10.1111/j.1151-2916.1968.tb12638.x
Raghuwanshi, V. S., Rüssel, C., and Hoell, A. (2014). Crystallization of ZrTiO4 nanocrystals in lithium-alumino-silicate glass ceramics: anomalous small-angle X-ray scattering investigation. Cryst. Growth Des. 14, 2838–2845. doi:10.1021/cg5001232
Ray, C. S., and Day, D. E. (1990). Determining the nucleation rate curve for lithium disilicate glass by differential thermal-analysis. J. Am. Ceram. Soc. 73, 439–442. doi:10.1111/j.1151-2916.1990.tb06532.x
Stookey, S. D. (1960a). Method of Making Ceramics and Product Thereof. US Patent Vol. 2920971. Corning Glass Works, USA.
Sinton, C. W., Crawford, A., Misture, S., Seeger, J., Wondraczek, L., and Deubener, J. (2008). High temperature reactions between soda-lime-silica glass and lithium aluminosilicate glass-ceramics. Glass Technol. Eur. J. Glass Sci. Technol. A 49, 133–138.
Soares, V. O., and Zanotto, E. D. (2016). Effect of P2O5 on the nonisothermal sinter-crystallization process of a lithium aluminum silicate glass. Int. J. Appl. Ceram. Technol. 13, 948–955. doi:10.1111/ijac.12540
Wang, M.-C., Li, W.-L., Cheng, C.-W., Chang, K.-M., Chen, Y.-F., and Hsi, C.-S. (2010). The phase transformation and crystallization kinetics of (1-x)Li2O-xNa2O-Al2O3-4SiO2 glasses. Materials Chemistry and Physics 123, 203–209. doi:10.1016/j.matchemphys.2010.03.083
Wurth, R., Munoz, F., Müller, M., and Rüssel, C. (2009). Crystal growth in a multicomponent lithia aluminosilicate glass. Mater. Chem. Phys. 116, 433–437. doi:10.1016/j.matchemphys.2009.04.010
Keywords: lithium aluminosilicate glass-ceramics, crystallization, viscosity, microstructure, phase assemblage
Citation: Fu Q, Wheaton BR, Geisinger KL, Credle AJ and Wang J (2016) Crystallization, Microstructure, and Viscosity Evolutions in Lithium Aluminosilicate Glass-Ceramics. Front. Mater. 3:49. doi: 10.3389/fmats.2016.00049
Received: 23 July 2016; Accepted: 17 October 2016;
Published: 08 November 2016
Edited by:
Wolfram Höland, Ivoclar Vivadent, LiechtensteinReviewed by:
William LaCourse, Alfred University, USADaqin Chen, Hangzhou Dianzi University, China
Christian Rüssel, Jena University, Germany
Copyright: © 2016 Fu, Wheaton, Geisinger, Credle and Wang. This is an open-access article distributed under the terms of the Creative Commons Attribution License (CC BY). The use, distribution or reproduction in other forums is permitted, provided the original author(s) or licensor are credited and that the original publication in this journal is cited, in accordance with accepted academic practice. No use, distribution or reproduction is permitted which does not comply with these terms.
*Correspondence: Qiang Fu, ZnVxMkBjb3JuaW5nLmNvbQ==