- Department of Biomedical Engineering, Yale University, New Haven, CT, United States
Stroke is among the leading causes of death and disability worldwide, 85% of which are ischemic. Current stroke therapies are limited by a narrow effective therapeutic time and fail to effectively complete the recovery of the damaged area. Magnetic resonance imaging of the subventricular zone (SVZ) following infarct/stroke has allowed visualization of new axonal connections and projections being formed, while new immature neurons migrate from the SVZ to the peri-infarct area. Such studies suggest that the SVZ is a primary source of regenerative cells for the repair and regeneration of stroke-damaged neurons and tissue. Therefore, the development of tissue engineered scaffolds that serve as a bioreplicative SVZ niche would support the survival of multiple cell types that reside in the SVZ. Essential to replication of the human SVZ microenvironment is the establishment of microvasculature that regulates both the healthy and stroke-injured blood–brain barrier, which is dysregulated poststroke. In order to reproduce this niche, understanding how cells interact in this environment is critical, in particular neural stem cells, endothelial cells, pericytes, ependymal cells, and microglia. Remodeling and repair of the matrix-rich SVZ niche by endogenous reparative mechanisms may then support functional recovery when enhanced by an artificial niche that supports the survival and proliferation of migrating vascular and neuronal cells. Critical considerations to mimic this area include an understanding of resident cell types, delivery method, and the use of biocompatible materials. Controlling stem cell survival, differentiation, and migration are key factors to consider when transplanting stem cells. Here, we discuss the role of the SVZ architecture and resident cells in the promotion and enhancement of endogenous repair mechanisms. We elucidate the interplay between the extracellular matrix composition and cell interactions prior to and following stroke. Finally, we review current cell and neuronal niche biomimetic materials that allow for a tissue-engineered approach in order to promote structural and functional restoration of neural circuitry. By creating an artificial mimetic SVZ, tissue engineers can strive to facilitate tissue regeneration and functional recovery.
Stroke: Impact and Background
Stroke is among the most prominent public health issues in the world, ranking among the top five leading causes of death and disability and impacting 1 in 6 people worldwide (Marlier et al., 2015). In the US alone, someone suffers a stroke every 4 s (Mozaffarian et al., 2016). Both the incidence and prevalence of stroke have been correlated to an aging population as well as socioeconomic burden (Boisserand et al., 2016), causing trends in incidence and severity of stroke outcomes to vary significantly between states and countries. Regardless of age or socioeconomic variables, most stroke patients present impaired motor and sensory function and output, though the severity of impairment may differ. The only current therapy for ischemic stroke being administration of recombinant tissue plasminogen activator (rtPA). While the treatment of rtPA therapy can be beneficial, it is currently constrained to just 2–5% of stroke patients, as rtPA is only effective if administered 4.5 h following symptom onset (Ruan et al., 2015). For patients who are unable to meet the criteria for rtPA therapy, intracranial hemorrhage often causes permanent neurological functional deficits (Ruan et al., 2015). A need for therapies that can be administered beyond a short therapeutic window remains, in addition to those that have the potential to restore the damaged tissue cavity that is formed by hypoxic conditions following stroke.
Responsible for approximately 85% of strokes (Boisserand et al., 2016), ischemia occurs when there is a blockade of a cerebral artery by a foreign clot or mass. This prevents sufficient blood flow through macrovessels and the invested microvasculature, thereby limiting oxygen exchange into the surrounding tissue. Reduction of blood supply under 15–20% of baseline levels contributes to tissue necrosis and irreversible damage (Sommer, 2017). Tissue necrosis and apoptosis can also be caused by ischemia-induced complications, including calcium overload, oxidative stress, and ATP dependence (Moskowitz et al., 2010). Vascular occlusion disrupts the cells and proteins that maintain the tightly regulated barrier at the vascular–tissue interface, known as the blood–brain barrier (BBB). The BBB contains endothelial cells (ECs), pericytes (PCs), a basement membrane, and astrocytic feet. Damage to one or all of these components causes increased permeability and subsequent edema and neuroinflammation. Furthermore, soluble factors including tumor necrosis factor alpha (TNF-α), thrombin, hemoglobin, and iron sulfate can be released into the local area through diffusion of soluble blood and blood plasma constituents, which may cause cytotoxic effects to the milieu (Nour et al., 2013). The volume of necrotic lesion core resulting from cellular and tissue damage is directly correlated to the extent of motor impairment experienced by the patient. The final infarct size, or area of dead tissue due to hypoxia, varies from patient to patient, contributing to the heterogeneity and complexity of this disorder (Alexander et al., 2010).
In an effort to achieve neurological function after stroke, neurogenesis creates new neurons (neuroblasts) in two germinal regions in the adult mammalian brain: the subventricular zone (SVZ) of the lateral ventricles (LV) and the subgranular zone (SGZ) in the hippocampus (Alvarez-Buylla and García-Verdugo, 2002). Under normal conditions, the adult brain inhibits new axonal sprouting. Recent studies have demonstrated that existing neurons may sprout new connections after the occurrence of ischemic stroke, as seen in Figure 1 (Carmichael, 2006). Axonal sprouting in peri-infarct tissue, or tissue surrounding the lesion core, results in remapping of connections adjacent to the infarct area, allowing for new connections to form up to several millimeters away from the infarct area (Carmichael, 2006). The degree of axonal sprouting depends on the carefully regulated induction of growth-promoting and inhibitory genes (Carmichael et al., 2001, 2005). Proteins involved in axonal growth including growth-associated protein 43, have been well studied, characterizing the precise network of molecules required for the growth cone phosphorylation cascade and initiation of axonal sprouting (Ng et al., 1988). The “trigger phase” inducing axonal sprouting occurs 1–3 days after stroke (Carmichael and Chesselet, 2002) and is followed by cytoskeletal rearrangement and gene transcription (Carmichael, 2006).
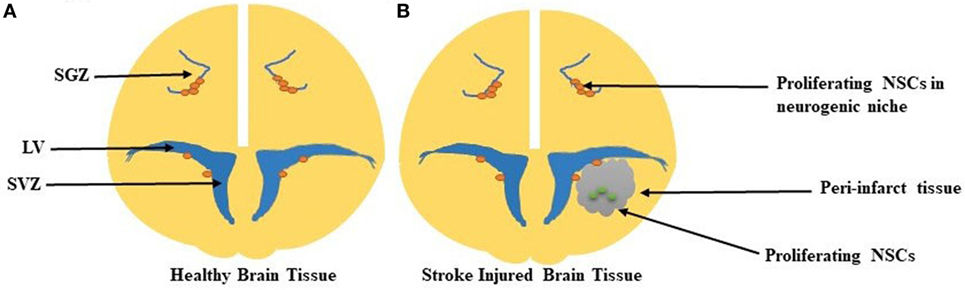
Figure 1. (A) Healthy brain tissue with proliferating neural stem cells (NSCs) in the neurogenic niches of both the subgranular zone (SGZ) and subventricular zone (SVZ). The SVZ lines the lateral wall of the lateral ventricle (LV). (B) In the stroke-injured brain, the peri-infarct tissue, which contains the ischemic core, contains proliferating NSCs in this damaged parenchyma.
In addition to neurogenesis, the process of angiogenesis, or development of new blood vessels, is necessary to achieve functional neurological recovery following stroke (Ruan et al., 2015). The peri-infarct area is hypoxic, triggering angiogenesis through upregulation of EC-secreted vascular endothelial growth factor (VEGF) within 6–24 h following occlusion. Angiogenesis is followed by a coordinated upregulation of the VEGF-receptor after 48 h (Marti et al., 2000). Due to this signaling cascade, EC proliferation and sprouting contribute to early and necessary microvessel formation, as the number of new vessels formed in the ischemic penumbra is associated with longer survival for ischemic stroke patients (Krupinski et al., 1994). Many factors beyond VEGF, such as fibroblast growth factor (FGF) and brain-derived neurotrophic factor (BDNF) regulate angiogenesis. These function to promote vasodilation and increase circulation and tissue oxygenation as initial steps for repair following ischemic damage (Ruan et al., 2015).
Angiogenesis and neurogenesis are coupled processes critical for functional and structural recovery of the brain infarct region following stroke. Neural stem cells (NSCs), cells with the capacity to differentiate into cells of all glial and neuronal lineages, are able to populate central nervous system (CNS) regions. NSCs are natively resident in the SVZ niche and secrete growth factors and chemokines that promote proliferation and expansion of the NSC pool. Regenerative capacity is maintained by switching from asymmetric division, a process allowing stem cells to self-renew, to symmetric division, a process enabling cells to expand in number after injury. Following NSC proliferation, neuroblasts (cells differentiated from NSCs that are committed to neuronal fate) migrate to the ischemic region and boundary of the infract region. This migration is characterized by cellular interactions between immature neuroblasts and blood vessels, suggesting that angiogenesis and neurogenesis are time-dependent and codependent upon one another (Ruan et al., 2015).
Goal of Tissue Engineering Strategies
In order to improve behavioral recovery following stroke, the goal of regenerative medicine is to successfully transplant stem or progenitor cells that have the ability to differentiate into neurons and integrate with the host microenvironment, improving both neurological and behavioral outcomes. Ideally, the act of transplantation and cell survival would enhance the endogenous repair processes, including axonal sprouting, neurogenesis, brain plasticity, and motor remapping. Integrated neural progenitor cells have the potential to replace and re-build impacted circuitry and reduce lesion size, in conjunction with the fine-coordinated events of angiogenesis and neurogenesis. This repair includes recruitment of vascular cells for increased neovascularization and vascular stabilization (Bliss et al., 2007; Vishwakarma et al., 2014).
Neural stem cells enact cell proliferation and recruitment through the production and secretion of growth factors that are correlated with recovery following ischemia, including endothelial growth factor (EGF) and FGF-2 (Greenberg and Jin, 2006; Vishwakarma et al., 2014). Some growth factors such as VEGF have a short-term impact, having demonstrated an increase in vascularity of infarcted tissue following stroke (Greenberg and Jin, 2006), and presenting upregulation in rat middle cerebral artery occlusion (MCAO) models after 3 h (Marti et al., 2000). Both insulin-growth factor-1 (IGF-1) and BDNF have also demonstrated promising results as acute therapies, beginning administration after 30 and 15 min post MCAO, respectively, and primarily attributed to reduction in infarct volume (Greenberg and Jin, 2006). Growth factor presentation in various forms is integral to promoting repair and functional recovery following stroke.
Tissue engineering constructs for the goal of neural regeneration and repair of the stroke region require that cell survival and activity be sustained and, often, enhanced for appropriate integration of exogenous transplanted cells into the host tissue. The successful use of biomaterials that recreate the SVZ native niche should support cell survival, proliferation, growth factor production, and protein secretion as necessary for appropriate cellular delivery and engraftment into the damaged area. Combining tissue engineering and cell transplantation advances can directly influence the differentiation and survival of neural progenitor and stem cells in vitro and in vivo (Ghuman and Modo, 2016).
Objective of Review
While we have come to understand many of the cellular and structural interactions that occur during stroke recovery, we remain unable to harness this knowledge to enhance therapeutic recovery. To date, there remains no effective treatment to foster recovery following ischemic stroke, though cell therapy has emerged as a promising approach to encourage functional replacement of the damaged neurons. As a therapeutic approach, transplanted NSCs have the potential to differentiate into numerous phenotypes required for neurogenesis in response to biological cues, and may integrate with the host environment to recover the effects of neurodegeneration. By incorporating factors that enhance both neurogenesis and angiogenesis through cell therapy, a neuro-restorative therapy can be created to promote re-growth in the neurogenic niche (Delcroix et al., 2010).
Here, we discuss attributes to an effective cell-based therapy, focusing on the resident SVZ niche cell types that are critical for both tissue homeostasis and response to injury. We elucidate many of the microenvironmental factors defined by the cytoarchitecture and extracellular matrix (ECM) that can influence the success of tissue engineered constructs for neural progenitor cell transplantation. Assessing how the SVZ is damaged poststroke, we consider the use of natural and synthetic biomaterials in facilitating recovery of the damaged ischemic core by creating a biomimetic microenvironment.
SVZ Structure and Biology
Cytoarchitecture
The SVZ is one of the two niches in the adult brain where neurons can regenerate, the other being the SGZ of the hippocampus (Alvarez-Buylla and García-Verdugo, 2002). This highly organized microenvironment begins to form during embryonic development with the generation of excitatory neurons. New excitatory neurons are generated in the ventricular zone that faces the LV, an area adjacent to the SVZ. Upon migration toward the brain surface during the developmental period, neurons that are born earlier are in deeper layers while neurons born later remain superficial (Tabata et al., 2012). The SVZ is highly vascularized and NSCs are in close contact with the rich vascular system, as evidenced by close interactions between NSCs (type-B), ependymal cells, and ECs. Direct contact between each of these cells and NSCs promote NSC self-renewal and neurogenesis in the SVZ, seen in Figure 2 (Doetsch, 2003).
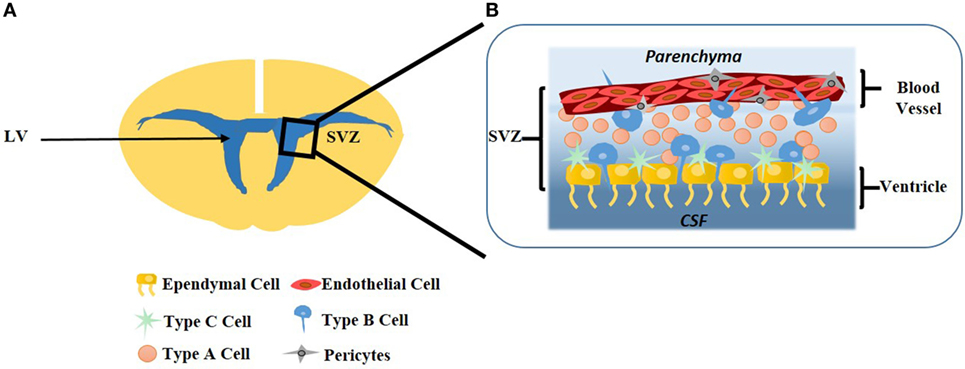
Figure 2. (A) The subventricular (SVZ) area is most prominent on the lateral wall of the lateral ventricle (LV). (B) Lining the ventricle is a layer of ependymal cells which form a single-cell layer. Type-C neural stem cells (NSCs) are transit-amplifying cells lining the ependymal cells. Type-B NSCs interact with the ependymal cells as well as the endothelial cells (ECs), where ECs form the vasculature of the blood–brain barrier. Type-A NSCs, known as neuroblasts, have the ability to migrate to the olfactory bulb. Pericytes surround ECs of the capillaries which facilitates communication with the surrounding microenvironment.
The microvasculature of the SVZ contains a more expansive vascular network than other areas of the brain, including non-neurogenic niches and the SGZ. While the microvasculature of the SVZ maintains vascular integrity to support the BBB, there are regions of the SVZ blood vessels where contact with astrocytic endfeet and microvasculature support cells, particularly PCs, is absent. The intermittent absence of astrocytic and PC contact with the vessel allows for transit-amplifying NSCs to directly contact the endothelium, enabling rapid signaling, and molecular exchange between the two cells (Girouard and Iadecola, 2006). The unique SVZ microvasculature suggests that a modified BBB in this niche exists (Goldberg and Hirschi, 2009).
Extrinsic factors that directly influence cells of the SVZ originate from blood vessels (Shen et al., 2008), the ependymal layer (Lim et al., 2000), and cerebrospinal fluid (CSF). In particular, the CSF is a major supplier of both proteins and small molecules responsible for signaling this niche (Falcão et al., 2012). The choroid plexus determines the composition of CSF in order to directly delivery these proteins and molecules to the SVZ and impact the behavior of neural progenitor cells (Lim et al., 2000; Shen et al., 2008). Secreted CSF is important in maintaining normal brain function as well as response to neuropathological conditions, where changes in CSF to the SVZ may alter the brain parenchyma metabolism.
The interactions between both ECs and PCs with NSCs, as well as with each other, are critical for maintaining vessel integrity and niche cell maintenance. The connection between type-B cells and ECs is characterized by short, thick processes extended from the body of NSCs in the ventricular zone. These processes allow cells to anchor to the basement membrane and migrate rapidly along the vessel length, enabling interactions critical for both NSC self-renewal and cell differentiation (Gonzalez-Cano et al., 2016). Clustering progenitor cells in close contact with cerebral ECs are supported in their proliferation and response to ischemia, though the process by which EC mediate this support has yet to be elucidated (Stolp and Molnár, 2015). Like ECs, PCs also intricately interact with type-B cells, where NSCs both project and ensheath PCs of the SVZ capillaries (Lacar et al., 2012). While abundant in large numbers within the SVZ, PC distribution along the length of brain microvessels varies, which is not surprising since the vascular endothelial tissue is extremely heterogeneous. Type-B cells control capillary tone in the SVZ due to the contractile nature of PCs (Goldberg and Hirschi, 2009). As a result, the interactions between vascular cells and NSCs are critical to the SVZ functioning as a neurogenic network.
Cell Types
Stem Cells
The goal of stem cell-based therapy is to replace dying and dead cells in the brain in order to restore damaged neural circuitry. There is a broad pool of stem cells with the potential to be used as therapeutic agents with the goal of de novo neuron and glial cell generation in following stroke-induced neurodegeneration (Lindvall and Kokaia, 2010). The use of NSCs, mesenchymal stem cells (MSCs), embryonic stem cells (ESCs), and induced pluripotent stem cells (IPSCs) are summarized in Table 1.
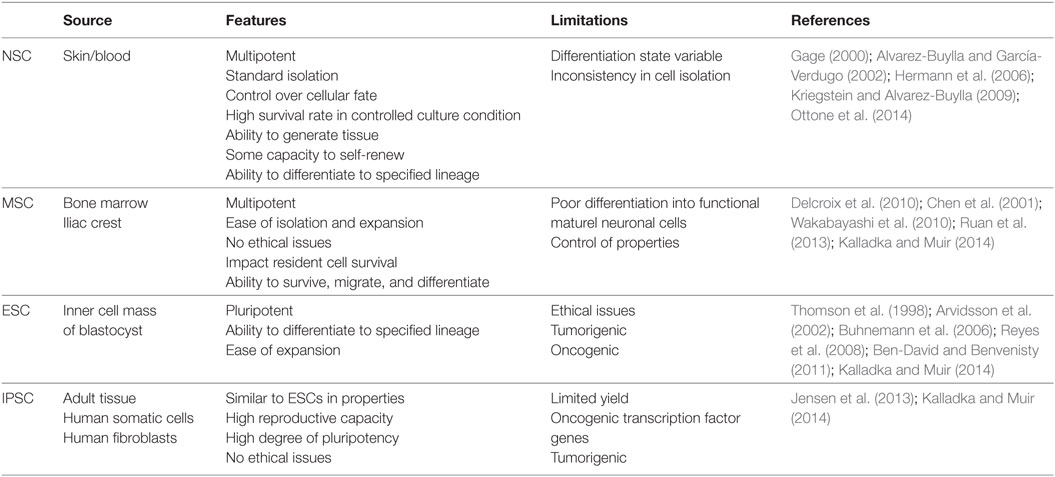
Table 1. A summary of neural stem cell (NSC), mesenchymal stem cell (MSC), embryonic stem cell (ESC), and induced pluripotent stem cell (IPSC) isolation sources, advantages, and limitations.
Neural Stem Cells
In the adult brain, NSCs can be subdivided into 3 types: (1) type-B quiescent stem cells, (2) type-C cells, which are transit-amplifying progenitors derived from activated type-B cells, and (3) type-A neuroblasts, which are formed from amplifying type-C cells that ultimately migrate to the olfactory bulb where they differentiate into interneurons (Ottone et al., 2014). Type-B cells, commonly referred to as SVZ astrocytes, are in close proximity to the vasculature and interact with ECs directly. Type-B cells are characterized both by their distinct morphology of long projections with specialized endfeet, as well as their expression of glial-fibrillary acidic protein (GFAP) (Kriegstein and Alvarez-Buylla, 2009). Because the interactions between quiescent type-B NSCs and ECs are key to the stability and remodeling of the SVZ, their relationship remains a highly active area of investigation (Alvarez-Buylla and García-Verdugo, 2002; Ottone et al., 2014).
Neural stem cells are able to generate neural tissue that possesses some capacity for self-renewal, as well as the ability to undergo asymmetric cell division to produce cells other than themselves, including neurons or glial cells (Gage, 2000). They can give rise to neurons, astrocytes, and oligodendrocytes in vitro and in vivo and have been positively correlated with replacement or repair to damaged brain tissue in animal models of stroke. NSCs in the adult brain are consistently present in the SVZ and the hippocampus (Hermann et al., 2006). NSC isolation for use in vitro requires excision of these regions of the brain and agitation of the tissue to dissociate cells (Gage, 2000). In vitro, cellular fate has been determined through staining with antibodies specific for neuron-specific class III B-tubulin (TUJ1), a marker specific for identification of NSC derived neurons. GFAP has been used for identification of astrocytes, and galactosylceramide is a marker used to identify oligodendrocytes. In vitro and in vivo observation of NSC differentiation suggests that cell fate to a specific lineage can be induced by withdrawing mitogens or by exposing cells to factors, such as FGF-2, to permit neurogenesis (Gage, 2000; Kriegstein and Alvarez-Buylla, 2009).
Neural stem cells are located within close proximity to the abundant vasculature of the SVZ. More than 50% of NSC nuclei within the murine SVZ are located within 20 µm of blood vessels. Additionally, NSC proliferation occurs close to blood vessels, suggesting that neurogenesis is dependent upon direct and indirect interaction with the vasculature. Endothelial signaling, specifically ephrin-B/EphB, regulates NSC proliferation and niche cell plasticity, while Notch signaling regulates NSC maintenance (Imayoshi et al., 2010; Nomura et al., 2010). These signaling pathways facilitate EC inhibition of NSC differentiation and limit NSC proliferation. ECs of the SVZ vasculature are functionally important for the NSCs lineage by regulating these cell processes (Shen et al., 2008).
To date, the consistency of data acquired from the use of NSCs has been limited, primarily due to inconsistent protocols in cell culture. As one example, mitogens EGF and FGF-2 are inconsistently used between laboratories to expand primitive cells. Many experimental protocols call for the individual use of such growth factors, where others suggest that most effective manipulation requires the combined use of growth factors. In addition, methods of cell isolation vary due to differences in dissection methods, donor age, and cell density within each neural region (Gage, 2000). The variability in NSC cultural protocol and isolation creates an undefined depiction of how to harvest NSCs, ultimately making utility studies and outcomes inconsistent.
Mesenchymal Stem Cells
Mesenchymal stem cells, multipotent adult stem cells, are isolated from the iliac crest or bone marrow and are easily expanded in vitro (Delcroix et al., 2010). MSCs can impact resident cell survival, including astrocytes, by upregulating kinase pathways. MSCs can then migrate to ischemic boundaries in several animal models with temporary or permanent MCAO. However, it remains unclear whether MSCs that differentiate along neuronal lines in culture are intrinsically similar to NSCs, as specific characterization of MSCs over time has resulted which makes comparability and consistency between studies difficult (Kalladka and Muir, 2014).
Mesenchymal stem cells have been utilized in animal models with stroke, demonstrating positive results in terms of repair. Transplantation of rat bone marrow-derived MSCs into a rat MCAO model has resulted in a reduction of infarct size. In this context, MSCs can survive, migrate, differentiate, and support significant recovery in motor and somatosensory behavioral tests in rats with transplanted MSCs upon activation with nerve growth factor (Chen et al., 2001). In fact, even xenotransplantation of human MSCs isolated from bone marrow has enhanced functional recovery of mice following induction of stroke (Ruan et al., 2013). Beyond MCAO injections, MSCs are delivered through various routes. For example, bone marrow MSCs delivered via intraventricular transplantation, carotid artery transplantation, and internal jugular vein injection have migrated to the brain injury area and cortex, surviving in the infarct area following middle MCAO in rats (Ruan et al., 2013). Rats receiving MSC transplantation demonstrated reduction in infarct volume at 7 and 14 days, as well as expression of neurotrophic factors VEGF, EGF, and FGF within 7 days (Wakabayashi et al., 2010). It appears that there is wide potential for MSC delivery to promote functional recovery through activation of endogenous restorative responses in the brain (Wakabayashi et al., 2010).
Embryonic Stem Cells
Embryonic stem cells are pluripotent cells derived from the first stage of embryonic development, containing the potential to replace tissues lost by injury (Reyes et al., 2008; Kalladka and Muir, 2014). However, due to their isolation source, the usage of ESCs presents ethical issues in medical research and as a potential therapeutic strategy (Kalladka and Muir, 2014). Despite these issues, ESCs have demonstrated major advantages for therapeutic uses and are studied intensely for in vitro generation of neuronal cell lines. The use of ESCs in xenotransplantation into the necrotic area of adult rats subjected to stroke demonstrates that murine ESCs improved functional outcome. In these studies, engrafted cells were able to survive within the infarct area up to 12 weeks following transplantation (Arvidsson et al., 2002). The support pluripotent ESCs provide to resident cells make them an attractive candidate for improving functional recovery poststroke.
In addition to promoting survival, ESCs can differentiate into specific neuronal populations, including glial cells, neurons, and astrocytes (Arvidsson et al., 2002). Transplanted and engrafted cells exhibit characteristics of functional neurons and astrocytes, including neuron-specific nuclear protein (NeuN) + and GFAP + expression, respectively. They also resemble mature glial cells with enhanced green fluorescent protein + expression (Buhnemann et al., 2006). Differentiation is successfully controlled through the use of specific media and culture conditions. For example, the use of media containing fetal bovine serum and ESC supplement coincides with upregulation of TUJ1, suggesting the differentiation of ESCs to neuronal cells (Reyes et al., 2008). By tightly controlling experimental parameters and timing in conjunction with growth and inhibitory factors in media and culture conditions, ESCs can be preferentially driven toward specific cell phenotypes (Arvidsson et al., 2002).
The expanded and immediate translation of ESCs to clinical application is somewhat limited because ESCs display tumorigenic properties in vivo and in vitro. For example, ESCs have formed teratomas when injected into immunodeficient mice (Ben-David and Benvenisty, 2011). ESCs also display a high level of telomerase activity, which is directly related to immortality in human cell lines (Thomson et al., 1998). Nonetheless, ESCs have shown positive results when transplanted to the diseased brain or spinal cord, directly or after pre-differentiation or genetic modification in culture. Experimentation utilizing ESC based clinical trials have been targeted for macular degeneration, Parkinson’s disease, and spinal cord injury (Trounson and McDonald, 2015). While it appears that there is potential for the use of ESCs in neural regeneration and repair, additional studies are needed to understand how tumorigenic characteristics of ESCs can be controlled before being considered for therapeutic applications.
Induced Pluripotent Stem Cells
Induced pluripotent stem cells can be reprogramed to an embryonic-like state, subsequently performing similarly to ESCs. IPSCs can be isolated from adult tissue, such as human somatic cells and fibroblasts and have both a high reproductive capacity while maintaining a high degree of pluripotency (Kalladka and Muir, 2014). However, there is a low yield achieved during induced reprogramming in addition to the expression of oncogenic transcription factors (Kalladka and Muir, 2014). Transplanted IPSCs have formed teratomas in rat MCAO models, and unlike ESCs, have demonstrated little effect in terms of functional recovery. Although some studies report improvement in function and reduced infarct volume, as well as differentiated neuronal cells following intracerebral implantation of human fibroblast derived IPSCs, there is limited functional improvement to be reported using stereotactic injections to the brain (Jensen et al., 2013; Kalladka and Muir, 2014). In order to improve the use of IPSCs, parameters such as the optimal timing following stroke, dose of cells, source, culture protocol of cells, and maturation time must be optimized (Jensen et al., 2013).
Endothelial Cells
Endothelial cells comprise the lumen of the brain microvasculature and are critical for regulating tissue homeostasis and facilitating information transfer between neurons and glial cells. During ischemic stroke, microvascular ECs are damaged by oxygen and nutrient deprivation, which results in degradation of tight junctions that produces an increase in BBB permeability. Following stroke, disturbance of the EC framework and local cytokine levels poses danger to the milieu due to ECs’ role in maintaining the BBB integrity when responding to injury (Girouard and Iadecola, 2006).
As previously discussed, angiogenesis and neurogenesis are interdependent processes that intrinsically rely upon each other. In the BBB, the vascular framework is formed through tight junctions, which are responsible for regulating vascular permeability and stabilizing the vessel wall. As the BBB is notably impermeable to large, polar molecules, true biomimetic in vitro models must maintain endothelial specific markers that facilitate vascular integrity and restrict permeability. Experimental use of the immortalized mouse brain EC line, bEnd3 is common because these cells retain the expression of mRNA and proteins that are required for the formation of tight junction proteins. These include zonal occludins-1 and -2 (ZO-1 and ZO-2), and claudin-5 (Fanning et al., 1998; Brown et al., 2007). Although immortalized brain ECs are utilized heavily in vitro, utilizing primary ECs can more closely mimic brain microvasculature. In order to prepare a pure culture, for example from a mouse, a standard protocol typically includes an enzyme-based tissue disruption, selective adhesion to collagen I, a culture in EC medium, and selective survival over non-ECs when cultured with a protein synthesis inhibitor (Welser-Alves et al., 2014). Utilizing brain ECs through benchtop work allows researchers to re-create complex 3-D environments and contribute to our understanding on how they contribute to vascular remodeling and BBB breakdown. In addition to controlling differentiation and promoting quiescence, ECs are critical for healing the neuronal niche following ischemic damage. Specifically, ECs promote NSCs survival through Notch signaling regulated growth factor secretion. Growth factors, including VEGF, can stimulate neurogenesis, demonstrating that the NSCs lie directly adjacent to the vasculature in an interdependent manner, ultimately promoting NSC homeostasis and survival (Shen et al., 2008).
Pericytes
Pericytes are microvascular mural cells that are instrumental to vasculogenesis, the process of new blood vessel formation during embryonic development, and vascular stability. PCs enact their roles in neurovasculature development and integrity maintenance through direct contact with microvascular EC and exchange of soluble factors between the two cells, where the highest density of PCs (EC:PC ratio 1:1) is in neural tissues (Korn et al., 2002; Geevarghese and Herman, 2014). PCs are capable of expressing proteoglycans, specifically chondroitin-sulfate proteoglycan-4, and other ECM proteins. They are known to support the structure of the microvasculature and provide signals necessary for the differentiation of plastic cells within the SVZ (Korn et al., 2002). There is no universal marker to identify PCs, rather they are typically recognized by their coexpression of multiple markers and absence of EC, fibroblast, leukocyte, and smooth muscle cell specific markers. Positive identifying markers for PC include chondroitin-sulfate proteoglycan neuron-glial antigen 2 NG2, platelet-derived growth factor-beta (PDGFβ) receptor, and CD13—a type II membrane metalloprotease (MMP) that is specific for brain PCs (Armulik et al., 2011). PC markers may be downregulated during various stages of development and location within organs, suggesting a heterogeneity among PCs. PC heterogeneity is correlated with specialized function required for vascular homeostasis in specific microenvironments (Girouard and Iadecola, 2006; Armulik et al., 2011).
Pericytes respond to EC-secreted PDGFβ, where PDGFβ receptor is functionally involved in PC recruitment during angiogenesis (Daneman et al., 2010; Armulik et al., 2011). PCs promote neurovasculature maturation and are influenced by transforming growth factor-beta (TGF-β) to regulate cell proliferation, differentiation, and survival. Secretion of TGF-β by ECs is elevated following ischemic stroke, indicating that the injured brain may induce mural cell expansion in order to promote neovascularization and repair (Rustenhoven et al., 2016). Primary rat brain PCs in coculture with mouse brain capillary ECs have exhibited both induction and upregulation of microvascular integrity. This was demonstrated by a decrease in permeability to sodium fluorescein, where the enhancement of integrity was inhibited when TGF-β activity was neutralized via antibody binding. This suggests that brain PCs contribute to BBB maintenance through TGF-β production (Dohgu et al., 2005).
Although PCs are present in the SVZ niche, their role is poorly understood. The contractile nature of PCs is known to regulate blood flow in ex vivo cerebellar brain slices and retina preparations; however, the mechanisms driving regulation of capillary tone and blood flow in the SVZ remain unknown (Armulik et al., 2011). Recent work in mouse models suggests that type-B NSCs send projections that ensheath PCs on SVZ capillaries may indirectly regulating capillary blood by activating purinergic receptors on PCs. Despite their ambiguous role, PCs are known regulators of oxygen and glucose, directly impacting niche cell proliferation and metabolic changes within the environment (Lacar et al., 2012). Continued studies investigating the signaling mechanisms involved in PC regulated blood flow are critical for a better understanding of the neuronal niche.
Ependymal Cells
Ependymal cells are multi-nucleated cells that intricately interact with the apical NSC layer and LV. Ependymal cells have microvilli and motile cilia, wherein cilia create a current of CSF along the LV walls. Malfunction of these cells is correlated with disturbance of CSF flow (Spassky et al., 2005). Ependymal cells are identified through their presentation of surface and intracellular proteins, including β-catenin, N-cadherin, Vimentin, and α-tubulin, in addition to their close proximity, within 5 µm, to vessels (Shen et al., 2008). Ependymal cells, like ECs, secrete soluble factor pigment epithelium-derived factor (PEDF) which potentially promotes NSC self-renewal and plays a role in NSC maintenance in the murine SVZ (Ramirez-Castillejo et al., 2006). The pathways associated with PEDF activation can present a therapeutic advantage by promoting self-renewal of NSCs in many neurodegenerative diseases.
In the SVZ, ependymal cells differ from ECs and NSCs in their role and behavior. For one, ependymal cells are not connected to one another nor their articulating cells via tight junctions (unlike ECs). This enables large molecules to penetrate the ependymal layer through the gap junctions that connect ependymal cells and astrocytes (Shen et al., 2008; Nomura et al., 2010). Ependymal cells exist in a postmitotic state and do not divide after differentiation, unlike NSCs (Spassky et al., 2005). Stroke injury is disruptive to the quiescent state of ependymal cells enforced by canonical Notch signaling, a signaling pathway that is necessary to conserve stem cell maintenance. In turn, neurogenesis is promoted through inhibition of Notch signaling following stroke, allowing ependymal cells to lose ependymal cell features, enter the cell cycle, and give rise to astrocytes and neuroblasts. The ependymal cells lack self-renewal capacity and do not maintain their population after stroke, unlike ECs and NSCs, suggesting their role as a reservoir recruited following injury (Carlen et al., 2009).
Ependymal cells play an important role in maintaining a neurogenic niche. These cells produce bone morphogenic protein (BMP) antagonist, Noggin, where Noggin antagonizes type-B cell BMP signaling and promotes neurogenesis in the SVZ. Type-B cell BMP signaling blocks the neurogenic pathway, instead promoting gliogenesis. Thereby, ependymal cells contribute to the promotion and enhancement of neurogenesis in the SVZ, which is critical to re-building damaged tissue (Lim et al., 2000).
Microglia
Microglia are resident immune cells known to maintain brain homeostasis. These cells monitor the microenvironment by responding to injury through the secretion of cytokines and phagocytosing cellular debris at the site (Weinstein et al., 2010; Ghuman and Modo, 2016). Within minutes following stroke, the brain tissue surrounding the core contains activated microglia that accumulate within the lesioned cavity (Patel et al., 2013). Microglia are detected using Iba-1 and Mac-2, markers for cells of myeloid origin and activated/proliferating resident microglia, respectively. Flow cytometry provides the ability to distinguish between residential and infiltrating microglia and myeloid cells, defined by low and high expression of cell-surface marker CD45, respectively. However, the stability of this marker during pathological state is in question (Lalancette-Hebert et al., 2007).
Microglial activation is triggered by ischemic stroke, where endogenous neuronal–glial interactions are disrupted, leading to upregulation of pro-inflammatory cytokines. CD200, a transmembrane glycoprotein expressed on neurons, and its receptor expressed on myeloid cells, modulate microglial activation under homeostasis, promoting quiescence until the occurrence of injury. Following stroke, CD200 gene transcription in the ischemic hemisphere is decreased (Patel et al., 2013), facilitating alternative activation of microglia. In addition, following injury the quiescence of microglia is also disrupted through a decrease in neurons secreting the cytokine fractalkine (CX3CL1). ATP release from dying neurons, as well as monocyte chemotactic protein-1 secretion from surrounding brain cells, induces chemotaxis and migration of microglia to the ischemic area (Patel et al., 2013).
Following tissue damage, changes in microglia migration patterns, cell-surface protein expressions and functions are observed. Motility of microglia toward injury site is seen within minutes. Microglial polarization promotes the development of two phenotypes; M1 or M2. M1 is a classical pro-inflammatory activation phenotype, increasing pro-inflammatory mediators including TNF-α, IL-6, IL-1, nitric oxide, and proteolytic enzymes including MMP-3 and MMP-9. M2, an alternative activation, releases anti-inflammatory mediators, including IGF-1, TGF-B, IL-10, IL-4, and IL-13 (Patel et al., 2013). The fine-tuned balance of M1 and M2 are offset by stroke. Identifying microglia of each subpopulation can contribute understanding of functional changes poststroke (Patel et al., 2013).
The pro-inflammatory mediators associated with the M1 phenotype can be detrimental to injured brain tissue. MMPs breakdown the ECM and BBB poststroke. This adverse effect is diminished using an MMP knock out mouse model, demonstrating less neuronal injury post ischemia. In addition, TNF-α is rapidly upregulated in the brain following injury within 1 h after induction of ischemia (Lambertsen et al., 2005) and inhibition of this pleiotropic cytokine has been displayed in studies to be neuroprotective (Barone et al., 1997). Microglia thereby plays a detrimental role in the acute phase by exacerbating the inflammatory phase.
Recent evidence suggests that activated microglia may actually provide benefits to the SVZ following stroke by orchestrating brain tissue repair. Following cerebral ischemia in transgenic mice, a resident proliferating microglia population presents an endogenous pool of neurotrophic molecules, including IGF-1, where activated microglial proliferation peaks 48–72 h post injury (Lalancette-Hebert et al., 2007). At 72 h postischemic injury, resident microglia undergo proliferative expansion and neutrophil infiltration is diminished, suggesting that microglia are resolving the acute inflammatory phase of repair and promoting reparative environmental remodeling (Denes et al., 2007). Stimulating microglial proliferation can thereby be explored as a therapeutic tool in order to assess the neuroprotective properties of these resident immune cells.
Role of the ECM
ECM Composition and Proteins
The ECM compromises 20% of the brain tissue volume and presents structural and functional proteins that are necessary for cellular function (Sobel, 1998). Brain-specific ECM varies in density and composition, as well as association with specific neuron types within different areas of the brain. Areas with mature synaptic activity show densely packed areas, known as perineuronal nets, directly impacting neuronal activity in this tight network (Bikbaev et al., 2015). The ECM comprises the basement membranes that support epithelia and vascular ECs. The BBB contains an endothelium basement membrane in addition to a parenchymal basement membrane. Under normal conditions, these two basement membranes are closely in contact. The parenchymal basement membrane is formed by astrocytes and creates a barrier for leukocyte migration into the brain parenchyma. The anatomical location between ECs and astrocytes regulates barrier function, provides physical support and anchoring for cells, and contains ligands that regulate cell processes and signaling through integrin and ECM receptor interactions.
There is a bidirectional relationship between cells and ECM proteins, where ECM proteins, once secreted, interact with cells to both induce and maintain the regulatory properties of this barrier system (Baeten and Akassoglou, 2011). These proteins include collagen, fibronectin, laminin, tenascin, and proteoglycans, interacting closely with ECs, astrocytes, PCs, and microglial, which secrete these proteins poststroke. Many tissue engineering strategies aim to expose cells to an environment within a scaffold that possesses biological ECM-derived molecules to drive cellular function (Delcroix et al., 2010).
The general structure of the ECM includes a variety of macromolecules including proteoglycans and fibrous proteins, each of which are expressed to different degrees in tissues throughout the body. For example, more than 20 distinct collagens have been identified which are expressed differentially in specific tissues based on mechanical requirements of the area. Laminins demonstrate multiple isoforms, synthesized by a variety of cells in a tissue specific manner, expressing explicit α, β, and γ chains. The effect of laminin on adjacent cells is exerted through integrins that recognize laminins, heightening the role of laminin mediating cell–ECM interactions. Laminin 2, specifically, promotes neurite outgrowth from neural cells, where laminin 5 and 10 are seem predominantly in the vascular basement membrane to mediate adhesion of platelets, leukocytes, and ECs. The tenascin family, a group of glycoproteins with strikingly diverse expression patterns, possesses complex domain structures, implying the molecule can interact with multiple ECM proteins. Tenascins are not expressed in normal adult tissues, however, under pathological conditions, including injury induced neovascularization, tenascin-c is expressed. Proteoglycans, heavily glycosylated proteins consisting of a core protein with covalently attached glycosaminoglycan chains, are grouped into several families, characterized by their composition. For example, decorin, a key member of a leucine-rich repeat proteoglycan family, is involved in signal transduction via EGF receptor. It is involved in modulation and differentiation of epithelial and ECs, and also interacts with TGF-β. The heparin sulfate proteoglycans have high affinity bonding to a range of cytokines and growth factors, including FGF and VEGF, implying an important role in pathological processes including the modulation of cell migration, proliferation, and differentiation. MMPs, the main ECM enzymes with proteolytic degradation potential, are responsible for the constant ECM remodeling, facilitating the synthesis and deposition of new ECM proteins and thereby plays an essential role in tissue repair and cell metastasis. At homeostasis, the adult brain has very little MMP expression and activity, though is the microenvironment is rapidly altered when activated by cytokines and growth factors that trigger tissue remodeling (Bosman and Stamenkovic, 2003). Overall, this intrinsically complex ECM system is essential for normal development as well as response to disease and injury, consequently there is an intricate interplay between resident cells and this matrix.
ECM–Stem Cell Interactions
Extracellular matrix components supply a microenvironment that promotes the maintenance of stem cell homeostasis, dictated by signals derived by both ECM–cell interactions and soluble factors. Integrins, a family of heterodimeric transmembrane receptors connecting extracellular environments to intracellular cytoskeletons, are key receptors that facilitate cellular processes including migration and differentiation. Integrins and cell presented matrix receptors mediate a number of interactions by activating downstream signaling. As an example, integrins directly activate downstream signaling through focal adhesion kinase and phosphoinositide 3-kinase, thereby regulating self-renewal and proliferation of cells including hematopoietic stem and progenitor cells. Epithelial cells in the brain express high levels of α6 and/or β1 integrins, which heterodimerize in order to generate a laminin receptor (Marthiens et al., 2010). In particular, α6β1 integrin facilitates cell binding to laminin and is required for NSC adhesion to SVZ blood vessels, where adult SVZ progenitor cells express α6β1 receptor. Blocking this receptor inhibits adhesion to ECs, negatively impacting SVZ progenitor cell proliferation in vivo, and highlights the importance of these integrin mediated interactions (Shen et al., 2008). In addition, integrins can bind directly to laminin, collagen, and fibronectin, and to cell-surface adhesion molecules including intercellular adhesion molecule-1 and vascular cell adhesion molecule-1, both present in the stem cell niche.
In addition to integrins, cadherin molecules mediate both cell–cell adhesions and interactions with cytoskeleton-associated proteins. Scaffold proteins β-catenin and α-catenin can interact with intracellular domains of cadherins, thereby connecting cadherins to the cytoskeletal network and forming stable adherns junctions by clustering cadherin molecules. E-cadherin, expressed by ependymal cells in the SVZ, forms adherns junctions between ependymal cells and NSCs. When eliminating E-cadherin in vitro, NSC self-renewal is reduced as seen in vivo by disrupting E-cadherin through mutations in areas where NSCs specifically reside (Karpowicz et al., 2009). NSCs use both cadherin and integrin to interact with the niche and exploiting the necessity of these adhesion molecules can aid transplantation approaches (Chen et al., 2013).
The ECM can regulate stem cell activity by non-canonical growth factor presentation. It both avidly binds growth factors, regulating their local availability, and functions as a reservoir, making growth factors unavailable and insoluble. By capturing FGF-2 from the milieu, the ECM of the NSC niche in the SVZ promotes growth factor activity in the niche and regulates the neurogenic niche. Thereby, the ECM is extremely dynamic in its response to regulating stem cell maintenance and activity by providing and controlling access to growth factors in the milieu (Gattazzo et al., 2014).
Due to the important role of ECM proteins in cell survival and function, many ECM moieties have been incorporated into engineered scaffolds as a strategy for creating microenvironments that promote neuronal regeneration (Lemons and Condic, 2008). Within biomimetic polymer scaffolds, presentation of laminin, collagen, or fibronectin to MSC in culture demonstrates that laminin, in contrast to fibronectin or collagen, is a strong promoter of neuronal differentiation. Laminin also positively impacts neurite length and the formation of a growth cone, the structure that drives axon extension, through integrin-dependent signaling. Laminin-211 can both increase and decrease neurite extension due to the affinity of integrin binding, demonstrating its ability to drive precise and complex integrin mediated pathways (Qian and Saltzman, 2004; Delcroix et al., 2010). While the entirety of the laminin protein has been used in investigation matrix derived cell function, isolated laminin derived peptides are responsible for specific cell function. Laminin derived peptides, including and Tyr–Ile–Gly–Ser–Arg (YIGSR) and Ile–Lys–Val–Ala–Val (IKVAV) sequences, are responsible for outgrowth of dorsal root ganglion neurons and are widely utilized in a variety of polymeric based scaffolds (Delcroix et al., 2010). The use of a scaffold to guide cell survival and differentiation in vivo includes incorporation of ECM components or derived peptides. As one example, the inclusion of fibronectin derived Arg–Gly–Asp (RGD) to poly-(ethylene glycol) (PEG) hydrogel systems illustrates improved human MSCs cell survival in vitro (Salinas and Anseth, 2008). Human plasma fibronectin and human plasma fibrinogen demonstrate enhanced survival of cells and increased cell metabolic activity in vitro when encapsulating human MSCs in fibronectin or fibrinogen containing hydrogel capsules, most likely due to integrin clustering and activation of extracellular signaling cascades such as the mitogen activated protein kinase cascade (Karoubi et al., 2009). Thereby, the use of ECM proteins can enhance therapeutic cell survival in vivo by triggering native cell pathways.
ECM Following Stroke
Following stroke, basement membranes of the BBB are degraded. New ECM proteins are deposited, either by secretion of ECM proteins, or leakage of plasma proteins into the CNS. Degradation of basement membranes after stroke demonstrates notable changes at later time points after injury, for instance, 12–18 h after MCAO in a rat model, although changes in cell presented matrix receptors occur within a few hours. Degradation occurs through proteolysis, mainly by MMPs and plasmin, where these are upregulated and activated following pathological conditions such as stroke (Baeten and Akassoglou, 2011). MMP-9, synthesized by both macrophages as well as ECs, is present within and at the periphery of infarct within 24 h, thereby creating a significant disturbance to BBB integrity following stroke. These degradation pathways are correlated with increased BBB disruption, as well as secondary inflammation and edema and the promotion of vascular permeability (Romanic et al., 1998).
As an inherent method of tissue repair following stroke, ECM proteins are deposited or penetrate the CNS to recover the structure of the microenvironment. In particular, osteopontin (OPN), a secreted glycosylated phosphoprotein, binds receptors that act on several cell types and is expressed in neurons, macrophages, and astrocytes. Following stroke, OPN is upregulated around the infarct zone, and appears to be beneficial after ischemic insult. In rat pups, OPN injections are correlated with reduction in infarct volume and improvement in functional recovery following ischemia (Baeten and Akassoglou, 2011). Fibrinogen, a major plasma protein involved in blood coagulation, cannot normally penetrate the CNS parenchyma due to the BBB. In stroke, however, fibrinogen can occlude blood vessels and leak in the brain, forming deposits and potentially increasing inflammation. In order to remove fibrin to reestablish blood flow, enzymatic degradation by plasmin as well as MMP-2/9 activity is mechanistically helpful (Baeten and Akassoglou, 2011). Considering the alteration of the ECM composition in response to BBB disruption and the direct impact on neurologic disease are functionally important when considering ECM functions in physiology and disease pathology.
Cell-Based Therapies
Cell Delivery Technologies
Cell therapies have demonstrated some ability to induce spontaneous recovery following stroke and can promote endogenous neurogenesis (Kalladka and Muir, 2014). Current methods of cell therapy include exogenous, autologous, and allogenic cell delivery into experimental stroke models, each presenting their own set of advantages and drawbacks. Exogenous cell therapy, in which cells are derived from a source external to the stroke, has demonstrated impact on cell migration, survival, and differentiation which subsequently promote functional recovery in animal models. However, these transplanted cells may induce an immunogenic response. An alternative to exogenous cell therapy is the use of autologous cells, which avoids the risk of rejection. This therapy presents its own challenges, though, as limited access to these cells often requires in vitro expansion, which may delay treatment. Allogenic cell therapy, or transplanting stem cells from a genetically similar donor, is potentially the most successful method of cell transplantation. The use of allogenic cells presents advantages of reduced immunogenicity, in addition to being readily available. Of note, ongoing research is still required in order to improve patient selection regarding eligibility for allogenic based therapy (Kalladka and Muir, 2014). Stereotaxic implantation, a minimally invasive surgical method relying on exact 3-D coordinate anatomical systems, is challenging due to time constraints, where lesion size varies greatly over time and from patient to patient. Intravenous (IV) delivery, a non-invasive delivery route, can overcome this set back, though there is a lack of a direct pathway of cells to the ischemic area. Although intra-arterial (IA) delivery can deliver cells to the peri-infarct area directly, occlusion of the target artery that persists can create a blockade for a route of delivery while also compromising survival of delivered cells (Kalladka and Muir, 2014). Interestingly, there is a lack of evidence that IA delivery is superior to IV when delivering bone derived MSCs to a mouse stroke model. With no difference in functional or structural outcomes detected (Yang et al., 2013), the ideal route of administration to optimize the effect of cell therapy, in particular that of stem cells, remains a matter of debate.
Stem cells can be used in stroke therapies to dynamically respond to a stroke-damaged tissue, in which stem cells have phenotypic properties that allow direct interaction with the host environment. Engrafted stem cells, unlike terminally differentiated cells, can respond dynamically to temporal and spatial changes of the environment that follow ischemic injury (Kim, 2004; Kalladka and Muir, 2014; Duncan et al., 2015). Delivery of stem cells to a brain-injury site has effectively reduced lesion size and host cell death. Additionally, successfully delivered stem cells have the potential to replace lost circuitry by forming new synaptic contacts. Even the use of stem cell xenografts in animal models has been shown to decrease infarct area, promote expression of the neuronal proteins such as synaptic proteins, and restore synaptic activity. In fact, stem cells can also strengthen existing synapses (Ishibashi et al., 2004; Bliss et al., 2007), promoting enhanced signaling among uninjured neurons. Further, an induction of host brain plasticity has been observed following exogenous cell engraftment. In this context, increased levels of factors (i.e., FGF and BDNF) that induce angiogenesis resulted in successful integration of transplanted cells with the host circuitry, as well as increased neovascularization and recruitment of endogenous progenitors (Bliss et al., 2007; Lindvall and Kokaia, 2010).
The use of cell therapy requires collaboration between neurologists and engineers to create new and effective delivery methods. Cellular behavior manipulation and induction are explored to enhance cell state prior to and after delivery (Lindvall and Kokaia, 2010). The approaches utilized for cell transplantation, including the control of differentiation and proliferation, are necessary to provide insight for ex vivo models that address relevant biological questions regarding SVZ cell interactions. Fundamental considerations include the cell type, cell number, and delivery vehicle and route. Here, we consider the key cell types native to the SVZ and their potential use as cell therapy: stem cells, ECs, ependymal cells, PCs, and microglia, summarized in Table 2.
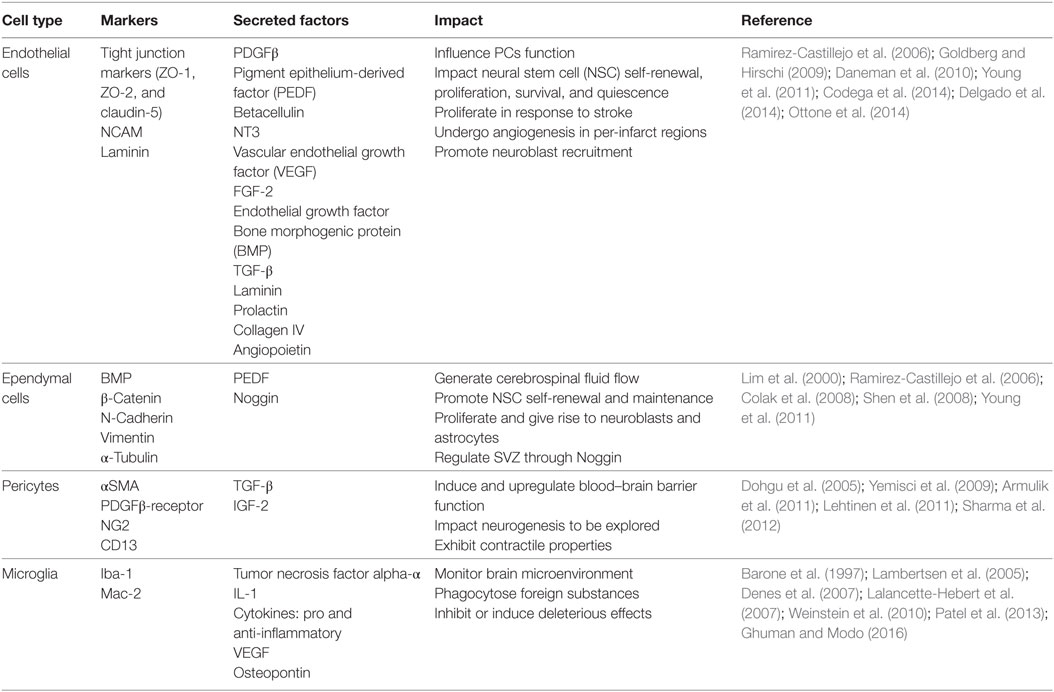
Table 2. Resident cell types of the subventricular zone (SVZ) niche, including endothelial cells, ependymal cells, pericytes (PCs), and microglia, with their respective cellular markers, secreted factors, and impact to other cells present in the niche.
Limitations to Successful Cell Therapy
The major restriction to successful transplantation of neural cells into the stroke-damaged area has been the survival of exogenous cells, which ranges from 1 to 32% (Zhang et al., 2001). Cell survival is compromised by delivery mechanisms that increase distorting force during syringe needle flow such as pressure drop, shearing, and stretching forces (Aguado et al., 2012). Viscosity and composition of delivery fluid contribute to cell distribution in solution and shear forces applied to cells during delivery. Additionally, sedimentation in carrier fluid becomes a limiting factor in successful cell delivery, causing the first partial injection volume to contain more cells than those delivered later. In order to achieve precise cell suspensions and cells with minimal damage, cellular delivery methods are the focus of innovation and optimization in stroke recovery therapeutics (Aguado et al., 2012; Potts et al., 2013).
The BBB and CSF barrier pose limitations to delivering therapeutics to the brain. Established delivery methods can be invasive, causing further damage to the ischemic area. Although systemic, intravenous delivery methods can be non-invasive, they often lead to an accumulation of cells in clearing organs, including kidney and spleen, rather than the brain. Targeted intracerebral cell injection can be applied directly to the peri-infarct region; however, the multiple injections required to deliver adequate numbers of cells can cause further damage to the already injured tissue (Ghuman and Modo, 2016). With minimal damage to an already fragile environment, invasive approaches are unfavorable due to the threat of further disruption of tissue and the BBB. Recent advances take these limitations in current therapeutic strategies into account, focusing on development of minimally invasive and non-damaging injections or delivery routes.
Biomimetic strategies face obstacles when considering clinical translation due to the fact the optimal cell type for transplantation therapy has not been determined. The use of stem cells in particular is impacted by ethical issues, where fetal and embryonic derived cells can create controversy. Generating an abundance of cells that maintain and recapitulate stemness is a challenge that limits the use of other cell types. Just as it has been seen with direct implantation and delivery of stem cells, many restrictions impact the success of these new therapies. First, the stroke-injured brain may compartmentalize areas of damaged tissue, creating a physical barrier to therapeutic deliveries. Stroke-injured brains also contain varying degrees of necrotic, avascular tissue, limiting access to systemic delivery of materials and cells through the vasculature. The variation in injury observed from person to person in each stroke occurrence causes significant difficulty in normalization of therapeutic methods. Due to the high variability of tissue damage and resultant function between patients, there is a lack of a standardized way to quantify both the severity and recovery of the disease. In order to form an effective therapy, these ambiguities must be addressed to formulate a common language that can measure functional outcomes in stroke trials.
Biomaterial-Based Biomimicry
We have detailed the importance of native and remodeled microenvironments in the SVZ and their role in driving cell function. The resident cell types have been described in addition to the ways these cells interact and impact one another. Both direct and indirect interactions with ECM molecules impact cell behavior, providing biomimetic scaffolds the opportunity to regulate cell behavior by modifying scaffolds to provide cues that cells in the host environment would have (Delcroix et al., 2010). Together, knowledge of SVZ cells, exogenous cells, and the ECM molecules that control cell fate and maintain the SVZ niche, tissue engineers can create biomaterials to produce a bioreplicative system that may improve experimental and therapeutic approaches.
Ideally, direct implantation of a biomaterial into the stroke-damaged area would promote cell adhesion and survival, cellular cues for healthy tissue development, and induce the recovery of the damaged ischemic core. Here, we discuss critical scaffold attributes required to facilitate poststroke healing in the brain, and two classes of biomaterials that have been demonstrated advantageous for brain repair: synthetic and natural materials.
Considerations for Development of an Ideal Scaffold
The ultimate goal of a biomimetic scaffold is to facilitate neural tissue regeneration and functional recovery following stroke. A scaffold must promote survival and proliferation of transplanted cells within the damage site, engage healthy interaction with endogenous cells, and promote the recovery of damaged neural circuitry. The mechanical and physical properties of a biomaterial impact the administration of the scaffold, the target injection site, and endogenous/exogenous cell response. In particular, solid scaffolds must present a compressive moduli allowing for cell survival rather than promoting tissue stress and damage upon implantation. A decrease in NSC proliferation in 3-D alginate scaffolds is correlated with an increase in the material modulus, where the greatest differentiation expression was attributed to the softest hydrogels. These soft hydrogels possess an elastic modulus comparable to brain tissue (100–1,000 Pa) (Banerjee et al., 2009). In addition to mechanical constraints, the initiation of the cellular and molecular cascade following transplantation must be considered. A biocompatible material is one that can coexist with living tissues without eliciting a local and systemic immune response in host tissue. Biocompatible materials do not promote a foreign body response, do not produce cytotoxic effects to the milieu, and limit the inflammatory and immune reaction in the brain (Boisserand et al., 2016). Byproducts from biomaterial degradation can be bioactive, influencing the surrounding environment and affecting both host and transplanted cells, thereby producing detrimental effects. Photopolymerization processes, which are cross-linking reactions that occur with light exposure, are often used for hydrogel production; however, these reactions can lead to the formation of free-radicals that compromise cell survival. Polymers that can polymerize at physiological conditions, dependent on temperature or pH, avoid these potential toxic effects (Ghuman and Modo, 2016). Consideration of how biomaterials will interact with host tissue is critical to contribute to functional recovery of both the infarct area and surrounding tissues.
Scaffold degradation over time to create or restore neural circuitry in stroke-damaged tissue. Degradation allows for integration of transplanted cells into the cavity. There is a fine balance between supporting cells during transplantation and controlling the rate of degradation. Injectable biomaterials, including hydrogels, are attractive candidates for stroke injury lesion cavities, which vary in size and morphology between patients. Hydrogels that structurally fill the cavity space and induce repopulation of cell depleted tissue space can be advantageous, as long as the mechanical properties are controlled in a manner that avoids further damage to the lesion cavity caused by increased intracerebral pressure with large volume of hydrogel injection. In fact, Modo et al. observed that microglia and astrocytes were able to infiltrate an ECM hydrogel where cell infiltration allows for a repopulation of host cells and ECM remodeling (Ghuman et al., 2016). While bulk hydrogel implantation into infarcted and lesional cavities has demonstrated some benefit, implantation of microencapsulated biological moieties has also demonstrated promise in the area of stroke recovery and repair. Microencapsulation techniques in which cells are encapsulated in approximately 95 microsphere diameter polymeric hydrogel spheres can be used to control the microenvironment an implanted cell is initially exposed to, thereby maintaining proper function of the cell type. In particular, is the maintenance of cell function is applicable to the SVZ niche where the cytoarchitecture organization is important to maintaining cellular and biochemical cues (Franco et al., 2011). Growth factor incorporation into biomaterials can contribute to creating biomimetic microenvironments. As one example, growth factors, including IGF-1, that are encapsulated into gelatin microspheres, promoted increased endogenous neurogenesis in the SVZ of adult mice. Hepatocyte growth factor containing microspheres also increased neuroblast migration from the SVZ to the stroke-injured tissue in the same model (Nakaguchi et al., 2012). FGF-2 in heparin–chitosan scaffolds demonstrates sustained survival and growth of NSC, where these multifunctional, biocompatible microspheres are optimized for NSC grafting (Struzyna et al., 2014). The use of stem cells and growth factors in conjunction with biocompatible and degradable scaffolds shows high potential to create a microenvironment that promotes functional recovery following injury. The use of 2-D polymer scaffolds has been advantageous as a means of exploring conditions for optimal cell growth and survival, for probing cell-surface interactions, and for trialing manipulations of the microenvironment that are impactful to cell response. In fact, such models that have been used to deconstruct the SVZ niche include the presentation of FGF covalently attached to a network of polyamide nanofibers. The scaffold maintained biological efficacy of FGF-2, strongly activating FGF receptors (Nur et al., 2008). In addition, EGF tethered to poly(methyl methacrylate)-graft-poly(ethylene oxide) promotes MSC spreading and survival around the biomaterial (Fan et al., 2007). These advantageous promote the use of 2-D polymeric substrates as bioreplicative constructs is a method of optimizing conditions for cell culture prior to advancement to 3-D replicates of the SVZ niche. In a 2-D model, cell–cell adhesions are confined to a horizontal plane, while a 3-D model allows for adhesions on all sides. A 3-D environment constrains cells to an artificial niche, enabling dynamic variation or continuous remodeling, which are not capable in a 2-D monolayer model.
Elastic, polymeric scaffolds make an ideal candidate for neurosurgical techniques to deliver scaffolds to the SVZ. While the goal is to fill the stroke cavity with a pliable and tunable scaffold which resembles mechanical properties of the native environment that will temporarily replace lost tissue, the material must also promote the infiltration of new and healthy cells. Rat-derived NSCs vary their cellular fate depending on the hydrogel stiffness, stressing the importance of biomaterial mechanical properties on differentiation potential (Leipzig and Shoichet, 2009). Stiffness and diffusion capacity must be carefully assessed prior to scaffold delivery in order to avoid detrimental effects to surrounding tissues in addition to differentiation to undesired cellular fates.
Synthetic Polymeric Materials
Synthetic biomaterials enable direct control of key properties, including degradation rate, material stiffness, and protein incorporation. Cell incorporation in combination with these materials calls for careful observation of cell–scaffold interactions, consequently allowing for control of cell processes, such as differentiation, morphological formation, and extension, to ultimately enhance connectivity in the ischemia area (Ghuman and Modo, 2016).
Poly-(Ethylene Glycol)
Poly-(ethylene glycol) is a biologically inert, non-toxic polymeric material, presenting excellent biocompatibility and resistance of protein adsorption. The mechanical properties of PEG depend on its molecular weight, as increasing the chain length of PEG increases stiffness and viscosity (Parlato et al., 2014). PEG can be chemically modified by tethering peptides or proteins through cross-linking reactions. Due to its inert nature, the incorporation of biologically active peptides cell-adhesive peptides, such as RGD and YIGSR, promotes cell attachment to the material (Gonzalez et al., 2004). PEGylation, the process of attaching PEG polymer chains to molecules and macrostructures, changes the chemical and physical properties of the material (Roudsari et al., 2016), making PEG a highly used polymer in a broad spectrum of tissue engineering applications. PEG-based microspheres modified by adhesive sequence RGD and metalloproteinase sensitive sequence have been utilized to encapsulate murine NSCs and brain ECs, demonstrating 60–80% cell viability, cell spreading, and migration through the scaffold (Franco et al., 2011).
In order to observe PEG as a candidate for neural repair and recovery, degradation characteristics as well as delivery of both cells, and growth factors are under investigation. As one example, varying mass profiles of PEG were examined. The astrocyte response varied with degradation rate, where slowly degrading/non-degrading gels display a prolonged astrocytic response. Astrocytes extended their processes into the hydrogel, where microglia infiltrated the hydrogel and facilitated the enzymatic process. Hydrogels decreased acute microglial response during the week following implantation, suggesting PEG-based materials are beneficial for CNS delivery for both drugs and cells (Bjugstad et al., 2010). In addition, PEG-based hydrogels with increased lactic acid content and encapsulated neural cells, including postmiotic neurons and multipotent precursor cells, demonstrate an increase in cell proliferation and survival, establishing an advantage of rendering PEG materials as degradable in order to be relevant to neural cells delivered to the stroke-injured brain (Lampe et al., 2010). Delivery of EGF using modified PEG hydrogels demonstrates a significant increase in tissue penetration as well as endogenous NSCs and progenitor cells in the SVZ when delivered to the ventricles of the brain. PEG modification in this way decreased EGF degradation by proteases, allowing for a greater protein accumulation in surrounding tissues, penetrating deeply into the tissues, seen in both the healthy and stroke-injured mice brain (Cooke et al., 2011). Thereby, these studies provide support for the use of PEG as a highly adaptable and tunable scaffold to be utilized for delivery of both cells and growth factors in stroke repair applications.
Polylactide Acid (PLA), Polyglycolide Acid (PGA), and Poly(Lactic-Co-Glycolic Acid) (PLGA)
Utilizing PLA and PGA alone or as the copolymer PLGA renders a bioactive polymer with the ability to closely control material properties, specifically degradation (Ghuman and Modo, 2016). PGA, a hydrophilic polymer, can rapidly degrade over a course of 2–4 weeks in vivo, while hydrophobic PLA degrades slower. Incorporation of these materials, PLGA in particular, can produce a variety of biomaterials, including nano and microparticles, which present high biocompatibility, low immunogenic response, and high structural support for NSCs to enhance brain repair (Boisserand et al., 2016).
Polyglycolide acid scaffolds have been effective for encapsulating NSCs, illustrating vascular formation and access to nutrients via diffusion (Park et al., 2002). In addition, a copolymer of PLGA-PEG nanoparticles loaded with thyroid hormone, which has a neuroprotective role in ischemia damage and is permeable across the BBB, demonstrates a decrease in tissue infarction and brain edema when delivered to a MCAO mouse model (Mdzinarishvili et al., 2013). PLGA based microspheres can bypass the inflammatory cascade and reduce host immune response, where these microspheres produce an inflammatory response similar to that of just a needle tract, presenting a peak in astrocyte activation 1-week post injection in rat brain models (Emerich et al., 1999). Another study shows human derived NSCs seeded on VEGF-releasing PLGA microparticles delivered to a MCAO rat model exhibiting a burst and sustained VEGF release, promoting attraction to ECs from the host, and facilitating the re-establishment of neovasculature through the cell and growth factor delivery (Bible et al., 2012). These results suggest that PLGA, due to its controlled degradation rates and high biocompatibility, has potential for a stroke therapeutic delivery system of drugs, cells, or reparative and regenerative factors.
Polypyrrole
Electric fields in vivo play a crucial role in control of cell potential and neuronal field potential. Electrical stimulation of stem cells is known to facilitate stem cell and progenitor cell fate. Experimentation using electrical properties may be beneficial to differentiate stem cells and re-build neural circuitry. The use of conductive scaffolds makes plausible the manipulation of stem cells, in particular, the use of Polypyrrole (PPy) (George et al., 2017). Following the seeding of cells, ion channel density and neurite outgrowth are manipulated by signaling intrinsic electrical cues. PPy generates a minimal inflammatory response, supports cell growth, and guides stem cells toward differentiation (Stewart et al., 2015). By applying electrical stimulation to NSCs seeded on laminin-coated PPy films in a timely manner demonstrates neuron differentiation (Stewart et al., 2015). A study preconditioning human neural progenitor cells in a cell chamber system seeded on a PPy scaffold presents an improvement in neurologic function in a rat stroke model (George et al., 2017). The VEGF-A pathway gene expression was altered following electrical stimulation, correlated with endogenous vasculature remodeling (George et al., 2017). This suggests that electrically preconditioning stem cells can be beneficial to enhance stroke recovery.
Natural Materials
Chitosan
Chitosan is a biodegradable, inexpensive material with low toxicity that can be modified through incorporation of ECM proteins to the surface, including laminin and fibronectin that regulate cell functions (Haipeng et al., 2000; Cheng et al., 2007; Yang et al., 2010). Accordingly, due to its ability to be modified, chitosan carriers have demonstrated NSC survival, proliferation, and cell differentiation into desired phenotypes (Yang et al., 2010). In terms of neural repair, chitosan use is advantageous as the material undergoes alkaline hydrolysis, directly controlling the amine content and ultimately impacting cell adhesion and neurite extension (Cheng et al., 2007). In addition, oxygen plasma treatment preserves the chitosan microstructure in nerve conduit models, confirming improvement in behavior and functional recovery (Cheng et al., 2007). Chitosan nanoparticles loaded with acetyl-11-keto-β-boswellic acid, a resin extract which may control inflammatory responses, demonstrate a neuroprotective effect in a MCAO rat model, reducing infarct volume and cell death (Ding et al., 2016). In addition, the encapsulation of ESC derived ECs into a chitosan-based hydrogel loaded with VEGF microtubes presents minimal cytotoxicity in vivo and high cell survival, inducing neovascularization in hind limb ischemia mouse models (Lee et al., 2015). Chitosan-based biomaterials continue to be explored for the use in nerve repair for brain injury due to the lack of immune response post injection.
Hyaluronic Acid (HA)
Hyaluronic acid is a linear-chain polysaccharide that has demonstrated a positive role in nerve regeneration as well as neuronal development. HA is biocompatible and ubiquitous in the body. HA plays a role in CNS development and has been utilized in conjunction with neuronal progenitor cells in various applications, including the use of photocrosslinkable hydrogels varying in stiffness, presenting differences in differentiation potential (Seidlits et al., 2010; Khaing et al., 2014). The mechanical properties of HA hydrogels can be manipulated, promoting the potential to direct neural progenitor differentiation (Seidlits et al., 2010) in addition to reducing scar formation following neurolysis (Ikeda et al., 2003). These hydrogel systems express mechanical properties similar to that of brain tissue and can be altered through photo cross-linking systems, testing a variety of compressive moduli. In normal tissue, hyaluronan has a range of molecular weights that play critical rolls in controlling cell motility, cell growth, and angiogenesis (Back et al., 2005). Engrafting transplanted mouse, human progenitor, and human glial-restricted precursor cells in HA-PEG gels demonstrates prolonged survival in the xenograft, despite the induction of a mild inflammatory response after 2 weeks (Liang et al., 2013). The optimization of HA based scaffolds is under exploration for neural repair therapy as this polysaccharide plays a key role in stabilizing the ECM and regulating cell processes such as adhesion, motility, proliferation, and differentiation.
Hyaluronan–Methylcellulose (HAMC)
In HAMC systems, fine tuning of material properties is obtained by altering both the composition and molar mass of HA and methylcellulose (MC). While hyaluronan and MC are both biocompatible, hyaluronan is beneficial due to its non-immunogenic and anti-inflammatory properties (Vercruysse and Prestwich, 1998), and MC is a non-cell-adhesive material with the ability to fill brain lesions (Wells et al., 1997; Tate et al., 2001). The combination of these two materials is an ideal option for many neural therapies. HAMC polymers are fast-gelling and can be made into an injectable material. The gelation mechanisms, degradation, and cell adhesion of these materials can be varied and are well characterized (Tate et al., 2001; Gupta et al., 2006; Shoichet et al., 2007). Future uses of HAMC models rely upon their non-immunogenic nature, which is advantageous for injection therapies due to the reduced risk of infection at the injection site.
Fibrin
Fibrin has been used extensively as a scaffold for nerve regeneration, allowing for axonal regeneration and cell migration in gap nerve injuries (Williams et al., 1983; Taylor et al., 2004). Since fibrin is susceptible to enzymatic degradation, neurites are able to degrade these gels via plasmin activity (Herbert et al., 1996). Fibrin-based drug delivery systems have been used to release neurotrophins, a family of proteins that positively impact survival and development of neurons. Fibrin gel systems have been used to promote cell migration, neural fiber extension, and growth factor delivery (Taylor et al., 2004). In addition, fibrin matrices have been used in conjunction with glial-derived neurotrophic factor to enhance neurite extension (Wood et al., 2009), further demonstrating the advantages of this material for re-building nerve connectivity. IPSCs mixed with a fibrin glue injected to an MCAO rat model resulted in a reduction in infarct volume, improvement in behavior, a reduction in pro-inflammatory cytokines, and an increase of anti-inflammatory cytokines (Chen et al., 2010).
Collagen
Collagen, one of the most abundant proteins, is a biocompatible and immunogenic material that promotes cell adhesion and growth (Cross et al., 2010). Collagen can be remodeled by cells and is readily accessible for research and therapeutic use, as it can be obtained commercially or through established extraction protocols. However, due to the weak bulk mechanical strength of collagen, collagen-based materials must be altered to mimic the ECM and facilitate native cell interactions and biophysical cues (Knapp et al., 1997). The modulus or tensile strength of collagen can be increased through the addition of molecules, such as glycosaminoglycans (Lee et al., 2001).
Due to the biocompatibility and tunable mechanical strength of collagen, studies using collagen hydrogels have demonstrated the ability to encapsulate NSCs to increase cell survival when compared to injection of cells alone into the lesion cavity. NSCs assemble into the pores of the collagen matrix, where cells survive, differentiate, and re-build neural synapses in the MCAO rat model, displaying the start of collagen degradation after 6 days. Degradation processes facilitate the recovery of neural tissue post ischemia and allow for NSC integration to the host network (Yu et al., 2010). The incorporation of hyaluronan, heparin, and collagen into a hydrogel loaded with NSCs into a photo thrombotic stroke mouse model exhibits survival of the neural cells transplanted into the infarct cavity, a decrease in activated microglia and macrophage cells around the scaffold, and an increase in cell survival (Zhong et al., 2010). Due to the remodeling potential of a collagen scaffold, in addition to its use for observing dynamic cell processes, collagen is an attractive biomaterial for improving angiogenesis and vasculogenesis in stroke models.
Perspective and Future Directions
Biomaterial platforms have great potential to mimic the native interactions of the SVZ. Nevertheless, these materials must induce resident cell function and limit further damage to the stroke-injured tissue, presenting significant challenges for researchers and engineers. Ideally, a biomaterial must be biocompatible and degradable. This promotes cell interactions including molecular and biological signaling and cues, which mimic the native transplantation niche. Intracranial delivery systems must be optimized, beginning from rodent models in order to enhance knowledge of SVZ maintenance and dynamic response following brain trauma. This expanding pool of knowledge will ultimately translate to human trials. The translation to human models, however, poses its own challenges, as the human SVZ differs from that of the rodent in cellular architecture. In addition, large-scale efficacy and randomized controlled trials must be conducted to ultimately test the efficiency and safety of stroke therapeutics.
In order to produce a biomimetic scaffold that takes into account the many requirements and considerations discussed here, intense collaboration between neurologists, neural cell biologists, tissue engineers, and imaging experts is required. More profound knowledge of stem cell therapy is necessary to determine whether stem cells take on the function of the cells they replace, or if the cells integrate and need training by surrounding cells to function. Such discoveries would also aid in the understanding of these cells’ impact on endogenous neurogenesis. The implications of these discoveries would contribute to neurological diseases that compromise brain homeostasis not limited to stroke, including Parkinson’s disease and Huntington’s disease. Using the ability of NSCs to differentiate and self-renew, these cells have great potential for cell replacement and gene therapy for a broad category of neurological disease (Kim, 2004). The observation of molecular pathways between the critical cell types discussed above will guide development of scaffolds that promote both neurogenesis and angiogenesis.
As survival of transplanted cells remains a major limitation of successful cell therapy, a tissue engineered strategy must focus on transplantation methods that do not compromise cell viability. In addition, therapies must be tunable by nature, thereby addressing patient heterogeneity. Patients show variety in lesion size, location, and neuroanatomy postischemic stroke. The availability of stem cells to incorporate into biomimetic scaffolds must further be explored to ensure that these cell sources, in addition to resident cells, will limit the inflammatory cascade from occurring. It is important that research further examine how immune cells can be neuroprotective or damaging or if their role is solely dependent on the microenvironment, in order to achieve both positive and negative impacts.
Imaging techniques will require advanced technology to monitor stem cell activity, biomarker expression, structural changes, and the integrity of the infarct area. Using fluorescent microscopy, distribution of transplanted cells and material can be observed over time, where migration of stem cells to the lesion site is desirable. In this way, temporal dynamics of migration can be observed in addition to histological analysis of transplanted cells to the lesion area. Cell tracking is made feasible through bioluminescent optical imaging at a low cost, however, limited by the low penetration of light. In order to track cells deeper in tissue, positron emission tomography, single-photon emission computed tomography, and magnetic resonance imaging (MRI) can penetrate deeper within a subject utilizing a radioactive isotope (Gavins and Smith, 2015). MRI allows for imaging modalities to assess pathophysiological changes, outcomes of cerebral ischemia, vascular lesions, and blood flow. By observing parenchyma changes through this tool, ischemic lesions and the advancing ischemia can be observed, as well as the direct consequences of the injury (Nour and Liebeskind, 2014). Computed tomographic scans of the brain allow monitoring of hemorrhaging, an observation required before intravenous rtPA can be administered (Menon et al., 2015). Customized imaging can give insight into the area of infarction over the course of therapy, where this enhanced detail is crucial when considering translation to human trial.
In summary, there is strong potential in our ability to produce and optimize biomaterials that mimic the SVZ, during homeostasis and following stroke. By incorporating multiple cell types, important matrix proteins and moieties, and appropriate biochemical and biomechanical cues native to the SVZ niche, promotion and enhancement of neurogenesis and angiogenesis can be attained. Once accomplished, this can revolutionize the limitations of stroke therapies, where there is currently no effective treatment for full recovery following ischemia. Future therapies must mimic the SVZ niche to maintain stem cells in a quiescent, undifferentiated state with controlled differentiation potential, in addition to the direct cell to cell interactions that regulate homeostasis and promote repair following injury. As a result, integrating neuroanatomy and biomaterials can be an advantageous collaboration to confront a pressing clinical need to produce a therapy with fine-tuned control over a biomaterial’s properties. Through the manipulation of engineered scaffolds, the ultimate goal of treating degenerative activity in the SVZ to allow for functional recovery and neural circuitry post-trauma can be achieved.
Author Contributions
RM and AG contributed to the writing and editing of this manuscript.
Conflict of Interest Statement
The authors declare that the research was conducted in the absence of any commercial or financial relationships that could be construed as a potential conflict of interest.
Acknowledgments
The authors would like to acknowledge Amanda Pellowe for review and comments in the writing of this manuscript.
Funding
This work was supported by the National Institutes of Health 5R01EB016629-03.
References
Aguado, B. A., Mulyasasmita, W., Su, J., Lampe, K. J., and Heilshorn, S. C. (2012). Improving viability of stem cells during syringe needle flow through the design of hydrogel cell carriers. Tissue Eng. Part A 18, 806–815. doi: 10.1089/ten.TEA.2011.0391
Alexander, L. D., Black, S. E., Gao, F., Szilagyi, G., Danells, C. J., and Mcilroy, W. E. (2010). Correlating lesion size and location to deficits after ischemic stroke: the influence of accounting for altered peri-necrotic tissue and incidental silent infarcts. Behav. Brain Funct. 6, 6–6. doi:10.1186/1744-9081-6-6
Alvarez-Buylla, A., and García-Verdugo, J. M. (2002). Neurogenesis in adult subventricular zone. J. Neurosci. 22, 629–634.
Armulik, A., Genové, G., and Betsholtz, C. (2011). Pericytes: developmental, physiological, and pathological perspectives, problems, and promises. Dev. Cell 21, 193–215. doi:10.1016/j.devcel.2011.07.001
Arvidsson, A., Collin, T., Kirik, D., Kokaia, Z., and Lindvall, O. (2002). Neuronal replacement from endogenous precursors in the adult brain after stroke. Nat. Med. 8, 963–970. doi:10.1038/nm747
Back, S. A., Tuohy, T. M. F., Chen, H., Wallingford, N., Craig, A., Struve, J., et al. (2005). Hyaluronan accumulates in demyelinated lesions and inhibits oligodendrocyte progenitor maturation. Nat. Med. 11, 966–972. doi:10.1038/nm1279
Baeten, K. M., and Akassoglou, K. (2011). Extracellular matrix and matrix receptors in blood-brain barrier formation and stroke. Dev. Neurobiol. 71, 1018–1039. doi:10.1002/dneu.20954
Banerjee, A., Arha, M., Choudhary, S., Ashton, R. S., Bhatia, S. R., Schaffer, D. V., et al. (2009). The influence of hydrogel modulus on the proliferation and differentiation of encapsulated neural stem cells. Biomaterials 30, 4695–4699. doi:10.1016/j.biomaterials.2009.05.050
Barone, F. C., Arvin, B., White, R. F., Miller, A., Webb, C. L., Willette, R. N., et al. (1997). Tumor necrosis factor-alpha. A mediator of focal ischemic brain injury. Stroke 28, 1233–1244. doi:10.1161/01.STR.28.6.1233
Ben-David, U., and Benvenisty, N. (2011). The tumorigenicity of human embryonic and induced pluripotent stem cells. Nat. Rev. Cancer 11, 268–277. doi:10.1038/nrc3034
Bible, E., Qutachi, O., Chau, D. Y. S., Alexander, M. R., Shakesheff, K. M., and Modo, M. (2012). Neo-vascularization of the stroke cavity by implantation of human neural stem cells on VEGF-releasing PLGA microparticles. Biomaterials 33, 7435–7446. doi:10.1016/j.biomaterials.2012.06.085
Bikbaev, A., Frischknecht, R., and Heine, M. (2015). Brain extracellular matrix retains connectivity in neuronal networks. Nature 5, 14527. doi:10.1038/srep14527
Bjugstad, K. B., Lampe, K., Kern, D. S., and Mahoney, M. (2010). Biocompatibility of poly(ethylene glycol)-based hydrogels in the brain: an analysis of the glial response across space and time. J. Biomed. Mater. Res. A. 95, 79–91. doi:10.1002/jbm.a.32809
Bliss, T., Guzman, R., Daadi, M., and Steinberg, G. K. (2007). Cell transplantation therapy for stroke. Stroke 38, 817–826. doi:10.1161/01.STR.0000247888.25985.62
Boisserand, L. S. B., Kodama, T., Papassin, J., Auzely, R., Moisan, A., Rome, C., et al. (2016). Biomaterial applications in cell-based therapy in experimental stroke. Stem Cells Int. 2016, 6810562. doi:10.1155/2016/6810562
Bosman, F. T., and Stamenkovic, I. (2003). Functional structure and composition of the extracellular matrix. J. Pathol. 200, 423–428. doi:10.1002/path.1437
Brown, R. C., Morris, A. P., and O’Neil, R. G. (2007). Tight junction protein expression and barrier properties of immortalized mouse brain microvessel endothelial cells. Brain Res. 1130, 17–30. doi:10.1016/j.brainres.2006.10.083
Buhnemann, C., Scholz, A., Bernreuther, C., Malik, C. Y., Braun, H., Schachner, M., et al. (2006). Neuronal differentiation of transplanted embryonic stem cell-derived precursors in stroke lesions of adult rats. Brain 129, 3238–3248. doi:10.1093/brain/awl261
Carlen, M., Meletis, K., Goritz, C., Darsalia, V., Evergren, E., Tanigaki, K., et al. (2009). Forebrain ependymal cells are notch-dependent and generate neuroblasts and astrocytes after stroke. Nat. Neurosci. 12, 259–267. doi:10.1038/nn.2268
Carmichael, S. T. (2006). Cellular and molecular mechanisms of neural repair after stroke: making waves. Ann. Neurol. 59, 735–742. doi:10.1002/ana.20845
Carmichael, S. T., Archibeque, I., Luke, L., Nolan, T., Momiy, J., and Li, S. (2005). Growth-associated gene expression after stroke: evidence for a growth-promoting region in peri-infarct cortex. Exp. Neurol. 193, 291–311. doi:10.1016/j.expneurol.2005.01.004
Carmichael, S. T., and Chesselet, M. F. (2002). Synchronous neuronal activity is a signal for axonal sprouting after cortical lesions in the adult. J. Neurosci. 22, 6062–6070.
Carmichael, S. T., Wei, L., Rovainen, C. M., and Woolsey, T. A. (2001). New patterns of intracortical projections after focal cortical stroke. Neurobiol. Dis. 8, 910–922. doi:10.1006/nbdi.2001.0425
Chen, J., Li, Y., Wang, L., Lu, M., Zhang, X., and Chopp, M. (2001). Therapeutic benefit of intracerebral transplantation of bone marrow stromal cells after cerebral ischemia in rats. J. Neurol. Sci. 189, 49–57. doi:10.1016/S0022-510X(01)00557-3
Chen, S., Lewallen, M., and Xie, T. (2013). Adhesion in the stem cell niche: biological roles and regulation. Development 140, 255–265. doi:10.1242/dev.083139
Chen, S. J., Chang, C. M., Tsai, S. K., Chang, Y. L., Chou, S. J., Huang, S. S., et al. (2010). Functional improvement of focal cerebral ischemia injury by subdural transplantation of induced pluripotent stem cells with fibrin glue. Stem Cells Dev. 19, 1757–1767. doi:10.1089/scd.2009.0452
Cheng, H., Huang, Y.-C., Chang, P.-T., and Huang, Y.-Y. (2007). Laminin-incorporated nerve conduits made by plasma treatment for repairing spinal cord injury. Biochem. Biophys. Res. Commun. 357, 938–944. doi:10.1016/j.bbrc.2007.04.049
Codega, P., Silva-Vargas, V., Paul, A., Maldonado-Soto, A. R., Deleo, A. M., Pastrana, E., et al. (2014). Prospective identification and purification of quiescent adult neural stem cells from their in vivo niche. Neuron 82, 545–559. doi:10.1016/j.neuron.2014.02.039
Colak, D., Mori, T., Brill, M. S., Pfeifer, A., Falk, S., Deng, C., et al. (2008). Adult neurogenesis requires Smad4-mediated bone morphogenic protein signaling in stem cells. J. Neurosci. 28, 434–446. doi:10.1523/JNEUROSCI.4374-07.2008
Cooke, M. J., Wang, Y., Morshead, C. M., and Shoichet, M. S. (2011). Controlled epi-cortical delivery of epidermal growth factor for the stimulation of endogenous neural stem cell proliferation in stroke-injured brain. Biomaterials 32, 5688–5697. doi:10.1016/j.biomaterials.2011.04.032
Cross, V. L., Zheng, Y., Won Choi, N., Verbridge, S. S., Sutermaster, B. A., Bonassar, L. J., et al. (2010). Dense type I collagen matrices that support cellular remodeling and microfabrication for studies of tumor angiogenesis and vasculogenesis in vitro. Biomaterials 31, 8596–8607. doi:10.1016/j.biomaterials.2010.07.072
Daneman, R., Zhou, L., Kebede, A. A., and Barres, B. A. (2010). Pericytes are required for blood-brain barrier integrity during embryogenesis. Nature 468, 562–566. doi:10.1038/nature09513
Delcroix, G. J. R., Schiller, P. C., Benoit, J.-P., and Montero-Menei, C. N. (2010). Adult cell therapy for brain neuronal damages and the role of tissue engineering. Biomaterials 31, 2105–2120. doi:10.1016/j.biomaterials.2009.11.084
Delgado, A. C., Ferron, S. R., Vicente, D., Porlan, E., Perez-Villalba, A., Trujillo, C. M., et al. (2014). Endothelial NT-3 delivered by vasculature and CSF promotes quiescence of subependymal neural stem cells through nitric oxide induction. Neuron 83, 572–585. doi:10.1016/j.neuron.2014.06.015
Denes, A., Vidyasagar, R., Feng, J., Narvainen, J., Mccoll, B. W., Kauppinen, R. A., et al. (2007). Proliferating resident microglia after focal cerebral ischaemia in mice. J. Cereb. Blood Flow Metab. 27, 1941–1953. doi:10.1038/sj.jcbfm.9600495
Ding, Y., Qiao, Y., Wang, M., Zhang, H., Li, L., Zhang, Y., et al. (2016). Enhanced neuroprotection of acetyl-11-keto-β-boswellic acid (AKBA)-loaded O-carboxymethyl chitosan nanoparticles through antioxidant and anti-inflammatory pathways. Mol. Neurobiol. 53, 3842–3853. doi:10.1007/s12035-015-9333-9
Doetsch, F. (2003). A niche for adult neural stem cells. Curr. Opin. Genet. Dev. 13, 543–550. doi:10.1016/j.gde.2003.08.012
Dohgu, S., Takata, F., Yamauchi, A., Nakagawa, S., Egawa, T., Naito, M., et al. (2005). Brain pericytes contribute to the induction and up-regulation of blood-brain barrier functions through transforming growth factor-beta production. Brain Res. 1038, 208–215. doi:10.1016/j.brainres.2005.01.027
Duncan, K., Gonzales-Portillo, G. S., Acosta, S. A., Kaneko, Y., Borlongan, C. V., and Tajiri, N. (2015). Stem cell-paved biobridges facilitate stem transplant and host brain cell interactions for stroke therapy. Brain Res. 1623, 160–165. doi:10.1016/j.brainres.2015.03.007
Emerich, D. F., Tracy, M. A., Ward, K. L., Figueiredo, M., Qian, R., Henschel, C., et al. (1999). Biocompatibility of poly (DL-lactide-co-glycolide) microspheres implanted into the brain. Cell Transplant. 8, 47–58. doi:10.1177/096368979900800114
Falcão, A. M., Marques, F., Novais, A., Sousa, N., Palha, J. A., and Sousa, J. C. (2012). The path from the choroid plexus to the subventricular zone: go with the flow! Front. Cell. Neurosci. 6:34. doi:10.3389/fncel.2012.00034
Fan, V. H., Tamama, K., Au, A., Littrell, R., Richardson, L. B., Wright, J. W., et al. (2007). Tethered epidermal growth factor provides a survival advantage to mesenchymal stem cells. Stem Cells 25, 1241–1251. doi:10.1634/stemcells.2006-0320
Fanning, A. S., Jameson, B. J., Jesaitis, L. A., and Anderson, J. M. (1998). The tight junction protein ZO-1 establishes a link between the transmembrane protein occludin and the actin cytoskeleton. J Biol Chem 273, 29745–29753. doi:10.1074/jbc.273.45.29745
Franco, C. L., Price, J., and West, J. L. (2011). Development and optimization of a dual-photoinitiator, emulsion-based technique for rapid generation of cell-laden hydrogel microspheres. Acta Biomater. 7, 3267–3276. doi:10.1016/j.actbio.2011.06.011
Gage, F. H. (2000). Mammalian neural stem cells. Science 287, 1433–1438. doi:10.1126/science.287.5457.1433
Gattazzo, F., Urciuolo, A., and Bonaldo, P. (2014). Extracellular matrix: a dynamic microenvironment for stem cell niche(). Biochim. Biophys. Acta 1840, 2506–2519. doi:10.1016/j.bbagen.2014.01.010
Gavins, F. N. E., and Smith, H. K. (2015). Cell tracking technologies for acute ischemic brain injury. J Cereb Blood Flow Metab 35, 1090–1099. doi:10.1038/jcbfm.2015.93
Geevarghese, A., and Herman, I. M. (2014). Pericyte-endothelial cross-talk: implications and opportunities for advanced cellular therapies. Transl. Res. 163, 296–306. doi:10.1016/j.trsl.2014.01.011
George, P. M., Bliss, T. M., Hua, T., Lee, A., Oh, B., Levinson, A., et al. (2017). Electrical preconditioning of stem cells with a conductive polymer scaffold enhances stroke recovery. Biomaterials 142, 31–40. doi:10.1016/j.biomaterials.2017.07.020
Ghuman, H., Massensini, A. R., Donnelly, J., Kim, S.-M., Medberry, C. J., Badylak, S. F., et al. (2016). ECM hydrogel for the treatment of stroke: characterization of the host cell infiltrate. Biomaterials 91, 166–181. doi:10.1016/j.biomaterials.2016.03.014
Ghuman, H., and Modo, M. (2016). Biomaterial applications in neural therapy and repair. Chin Neurosurg J 2, 34. doi:10.1186/s41016-016-0057-0
Girouard, H., and Iadecola, C. (2006). Neurovascular coupling in the normal brain and in hypertension, stroke, and Alzheimer disease. J. Appl. Physiol. 100, 328–335. doi:10.1152/japplphysiol.00966.2005
Goldberg, J. S., and Hirschi, K. K. (2009). Diverse roles of the vasculature within the neural stem cell niche. Regen. Med. 4, 879–897. doi:10.2217/rme.09.61
Gonzalez, A. L., Gobin, A. S., West, J. L., Mcintire, L. V., and Smith, C. W. (2004). Integrin interactions with immobilized peptides in polyethylene glycol diacrylate hydrogels. Tissue Eng. 10, 1775–1786. doi:10.1089/ten.2004.10.1775
Gonzalez-Cano, L., Fuertes-Alvarez, S., Robledinos-Anton, N., Bizy, A., Villena-Cortes, A., Fariñas, I., et al. (2016). p73 is required for ependymal cell maturation and neurogenic SVZ cytoarchitecture. Dev. Neurobiol. 76, 730–747. doi:10.1002/dneu.22356
Greenberg, D. A., and Jin, K. (2006). Growth factors and stroke. NeuroRx 3, 458–465. doi:10.1016/j.nurx.2006.08.003
Gupta, D., Tator, C. H., and Shoichet, M. S. (2006). Fast-gelling injectable blend of hyaluronan and methylcellulose for intrathecal, localized delivery to the injured spinal cord. Biomaterials 27, 2370–2379. doi:10.1016/j.biomaterials.2005.11.015
Haipeng, G., Yinghui, Z., Jianchun, L., Yandao, G., Nanming, Z., and Xiufang, Z. (2000). Studies on nerve cell affinity of Chitosan-derived materials. J. Biomed. Mater. Res. 52, 285–295. doi:10.1002/1097-4636(200011)52:2%3C285::AID-JBM7%3E3.0.CO;2-G
Herbert, C. B., Bittner, G. D., and Hubbell, J. A. (1996). Effects of fibinolysis on neurite growth from dorsal root ganglia cultured in two- and three-dimensional fibrin gels. J. Comp. Neurol. 365, 380–391. doi:10.1002/(SICI)1096-9861(19960212)365:3%3C380::AID-CNE4%3E3.0.CO;2-0
Hermann, A., Maisel, M., and Storch, A. (2006). Epigenetic conversion of human adult bone mesodermal stromal cells into neuroectodermal cell types for replacement therapy of neurodegenerative disorders. Expert Opin. Biol. Ther. 6, 653–670. doi:10.1517/14712598.6.7.653
Ikeda, K., Yamauchi, D., Osamura, N., Hagiwara, N., and Tomita, K. (2003). Hyaluronic acid prevents peripheral nerve adhesion. Br. J. Plast. Surg. 56, 342–347. doi:10.1016/S0007-1226(03)00197-8
Imayoshi, I., Sakamoto, M., Yamaguchi, M., Mori, K., and Kageyama, R. (2010). Essential roles of notch signaling in maintenance of neural stem cells in developing and adult brains. J Neurosci 30, 3489–3498. doi:10.1523/JNEUROSCI.4987-09.2010
Ishibashi, S., Sakaguchi, M., Kuroiwa, T., Yamasaki, M., Kanemura, Y., Shizuko, I., et al. (2004). Human neural stem/progenitor cells, expanded in long-term neurosphere culture, promote functional recovery after focal ischemia in Mongolian gerbils. J. Neurosci. Res. 78, 215–223. doi:10.1002/jnr.20246
Jensen, M. B., Yan, H., Krishnaney-Davison, R., Al Sawaf, A., and Zhang, S.-C. (2013). Survival and differentiation of transplanted neural stem cells derived from human induced pluripotent stem cells in a rat stroke model. J Stroke Cerebrovasc Dis 22, 304–308. doi:10.1016/j.jstrokecerebrovasdis.2011.09.008
Kalladka, D., and Muir, K. W. (2014). Brain repair: cell therapy in stroke. Stem Cells Cloning 7, 31–44. doi:10.2147/SCCAA.S38003
Karoubi, G., Ormiston, M. L., Stewart, D. J., and Courtman, D. W. (2009). Single-cell hydrogel encapsulation for enhanced survival of human marrow stromal cells. Biomaterials 30, 5445–5455. doi:10.1016/j.biomaterials.2009.06.035
Karpowicz, P., Willaime-Morawek, S., Balenci, L., Deveale, B., Inoue, T., and Van Der Kooy, D. (2009). E-cadherin regulates neural stem cell self-renewal. J Neurosci 29, 3885–3896. doi:10.1523/JNEUROSCI.0037-09.2009
Khaing, Z. Z., Thomas, R. C., Geissler, S. A., and Schmidt, C. E. (2014). Advanced biomaterials for repairing the nervous system: what can hydrogels do for the brain? Mater. Today 17, 332–340. doi:10.1016/j.mattod.2014.05.011
Kim, S. U. (2004). Human neural stem cells genetically modified for brain repair in neurological disorders. Neuropathology 24, 159–171. doi:10.1111/j.1440-1789.2004.00552.x
Knapp, D. M., Barocas, V. H., Moon, A. G., Yoo, K., Petzold, L. R., and Tranquillo, R. T. (1997). Rheology of reconstituted type I collagen gel in confined compression. J Rheol 41, 971–993. doi:10.1122/1.550817
Korn, J., Christ, B., and Kurz, H. (2002). Neuroectodermal origin of brain pericytes and vascular smooth muscle cells. J. Comp. Neurol. 442, 78–88. doi:10.1002/cne.1423
Kriegstein, A., and Alvarez-Buylla, A. (2009). The glial nature of embryonic and adult neural stem cells. Annu. Rev. Neurosci. 32, 149–184. doi:10.1146/annurev.neuro.051508.135600
Krupinski, J., Kaluza, J., Kumar, P., Kumar, S., and Wang, J. M. (1994). Role of angiogenesis in patients with cerebral ischemic stroke. Stroke 25, 1794–1798. doi:10.1161/01.STR.25.9.1794
Lacar, B., Herman, P., Platel, J.-C., Kubera, C., Hyder, F., and Bordey, A. (2012). Neural progenitor cells regulate capillary blood flow in the postnatal subventricular zone. J. Neurosci. 32, 16435–16448. doi:10.1523/JNEUROSCI.1457-12.2012
Lalancette-Hebert, M., Gowing, G., Simard, A., Weng, Y. C., and Kriz, J. (2007). Selective ablation of proliferating microglial cells exacerbates ischemic injury in the brain. J. Neurosci. 27, 2596–2605. doi:10.1523/JNEUROSCI.5360-06.2007
Lambertsen, K. L., Meldgaard, M., Ladeby, R., and Finsen, B. (2005). A quantitative study of microglial-macrophage synthesis of tumor necrosis factor during acute and late focal cerebral ischemia in mice. J. Cereb. Blood Flow Metab. 25, 119–135. doi:10.1038/sj.jcbfm.9600014
Lampe, K. J., Bjugstad, K. B., and Mahoney, M. J. (2010). Impact of degradable macromer content in a poly(ethylene glycol) hydrogel on neural cell metabolic activity, redox state, proliferation, and differentiation. Tissue Eng. Part A 16, 1857–1866. doi:10.1089/ten.tea.2009.0509
Lee, C. R., Grodzinsky, A. J., and Spector, M. (2001). The effects of cross-linking of collagen-glycosaminoglycan scaffolds on compressive stiffness, chondrocyte-mediated contraction, proliferation and biosynthesis. Biomaterials 22, 3145–3154. doi:10.1016/S0142-9612(01)00067-9
Lee, S., Valmikinathan, C. M., Byun, J., Kim, S., Lee, G., Mokarram, N., et al. (2015). Enhanced therapeutic neovascularization by CD31-expressing cells and embryonic stem cell-derived endothelial cells engineered with chitosan hydrogel containing VEGF-releasing microtubes. Biomaterials 63, 158–167. doi:10.1016/j.biomaterials.2015.06.009
Lehtinen, M. K., Zappaterra, M. W., Chen, X., Yang, Y. J., Hill, A. D., Lun, M., et al. (2011). The cerebrospinal fluid provides a proliferative niche for neural progenitor cells. Neuron 69, 893–905. doi:10.1016/j.neuron.2011.01.023
Leipzig, N. D., and Shoichet, M. S. (2009). The effect of substrate stiffness on adult neural stem cell behavior. Biomaterials 30, 6867–6878. doi:10.1016/j.biomaterials.2009.09.002
Lemons, M. L., and Condic, M. L. (2008). Integrin signaling is integral to regeneration. Exp. Neurol. 209, 343–352. doi:10.1016/j.expneurol.2007.05.027
Liang, Y., Walczak, P., and Bulte, J. W. M. (2013). The survival of engrafted neural stem cells within hyaluronic acid hydrogels. Biomaterials 34, 5521–5529. doi:10.1016/j.biomaterials.2013.03.095
Lim, D. A., Tramontin, A. D., Trevejo, J. M., Herrera, D. G., Garcia-Verdugo, J. M., and Alvarez-Buylla, A. (2000). Noggin antagonizes BMP signaling to create a niche for adult neurogenesis. Neuron 28, 713–726. doi:10.1016/S0896-6273(00)00148-3
Lindvall, O., and Kokaia, Z. (2010). Stem cells in human neurodegenerative disorders – time for clinical translation? J. Clin. Invest. 120, 29–40. doi:10.1172/JCI40543
Marlier, Q., Verteneuil, S., Vandenbosch, R., and Malgrange, B. (2015). Mechanisms and functional significance of stroke-induced neurogenesis. Front. Neurosci. 9:458. doi:10.3389/fnins.2015.00458
Marthiens, V., Kazanis, I., Moss, L., Long, K., and Ffrench-Constant, C. (2010). Adhesion molecules in the stem cell niche – more than just staying in shape? J. Cell. Sci. 123, 1613–1622. doi:10.1242/jcs.054312
Marti, H. J. H., Bernaudin, M., Bellail, A., Schoch, H., Euler, M., Petit, E., et al. (2000). Hypoxia-induced vascular endothelial growth factor expression precedes neovascularization after cerebral ischemia. Am. J. Pathol. 156, 965–976. doi:10.1016/S0002-9440(10)64964-4
Mdzinarishvili, A., Sutariya, V., Talasila, P. K., Geldenhuys, W. J., and Sadana, P. (2013). Engineering triiodothyronine (T3) nanoparticle for use in ischemic brain stroke. Drug Deliv Transl Res 3, 309–317. doi:10.1007/s13346-012-0117-8
Menon, B. K., Campbell, B. C. V., Levi, C., and Goyal, M. (2015). Role of imaging in current acute ischemic stroke workflow for endovascular therapy. Stroke 46, 1453–1461. doi:10.1161/STROKEAHA.115.009160
Moskowitz, M. A., Lo, E. H., and Iadecola, C. (2010). The science of stroke: mechanisms in search of treatments. Neuron 67, 181–198. doi:10.1016/j.neuron.2010.07.002
Mozaffarian, D., Benjamin, E. J., Go, A. S., Arnett, D. K., Blaha, M. J., Cushman, M., et al. (2016). Executive summary: heart disease and stroke statistics – 2016 update: a report from the American Heart Association. Circulation 133, 447–454. doi:10.1161/CIR.0000000000000366
Nakaguchi, K., Jinnou, H., Kaneko, N., Sawada, M., Hikita, T., Saitoh, S., et al. (2012). Growth factors released from gelatin hydrogel microspheres increase new neurons in the adult mouse brain. Stem Cells Int. 2012, 7. doi:10.1155/2012/915160
Ng, S.-C., De La Monte, S. M., Conboy, G. L., Karns, L. R., and Fishman, M. C. (1988). Cloning of human GAP 43: growth association and ischemic resurgence. Neuron 1, 133–139. doi:10.1016/0896-6273(88)90197-3
Nomura, T., Göritz, C., Catchpole, T., Henkemeyer, M., and Frisén, J. (2010). EphB signaling controls lineage plasticity of adult neural stem cell niche cells. Cell Stem Cell 7, 730–743. doi:10.1016/j.stem.2010.11.009
Nour, M., and Liebeskind, D. S. (2014). Imaging of cerebral ischemia: from acute stroke to chronic disorders. Neurol. Clin. 32, 193–209. doi:10.1016/j.ncl.2013.1007.1005
Nour, M., Scalzo, F., and Liebeskind, D. S. (2013). Ischemia-reperfusion injury in stroke. Interv Neurol 1, 185–199. doi:10.1159/000353125
Nur, E. K. A., Ahmed, I., Kamal, J., Babu, A. N., Schindler, M., and Meiners, S. (2008). Covalently attached FGF-2 to three-dimensional polyamide nanofibrillar surfaces demonstrates enhanced biological stability and activity. Mol. Cell. Biochem. 309, 157–166. doi:10.1007/s11010-007-9654-8
Ottone, C., Krusche, B., Whitby, A., Clements, M., Quadrato, G., Pitulescu, M. E., et al. (2014). Direct cell–cell contact with the vascular niche maintains quiescent neural stem cells. Nat. Cell Biol. 16, 1045–1056. doi:10.1038/ncb3045
Park, K. I., Teng, Y. D., and Snyder, E. Y. (2002). The injured brain interacts reciprocally with neural stem cells supported by scaffolds to reconstitute lost tissue. Nat Biotechnol 20, 1111–1117. doi:10.1038/nbt751
Parlato, M., Reichert, S., Barney, N., and Murphy, W. L. (2014). Poly(ethylene glycol) hydrogels with adaptable mechanical and degradation properties for use in biomedical applications(). Macromol. Biosci. 14, 687–698. doi:10.1002/mabi.201300418
Patel, A. R., Ritzel, R., Mccullough, L. D., and Liu, F. (2013). Microglia and ischemic stroke: a double-edged sword. Int. J. Physiol. Pathophysiol. Pharmacol. 5, 73–90.
Potts, M. B., Silvestrini, M. T., and Lim, D. A. (2013). Devices for cell transplantation into the central nervous system: design considerations and emerging technologies. Surg. Neurol. Int. 4, S22–S30. doi:10.4103/2152-7806.109190
Qian, L., and Saltzman, W. M. (2004). Improving the expansion and neuronal differentiation of mesenchymal stem cells through culture surface modification. Biomaterials 25, 1331–1337. doi:10.1016/j.biomaterials.2003.08.013
Ramirez-Castillejo, C., Sanchez-Sanchez, F., Andreu-Agullo, C., Ferron, S. R., Aroca-Aguilar, J. D., Sanchez, P., et al. (2006). Pigment epithelium-derived factor is a niche signal for neural stem cell renewal. Nat. Neurosci. 9, 331–339. doi:10.1038/nn1657
Reyes, J. H., O’Shea, K. S., Wys, N. L., Velkey, J. M., Prieskorn, D. M., Wesolowski, K., et al. (2008). Glutamatergic neuronal differentiation of mouse embryonic stem cells following transient expression of neurogenin 1 and treatment with BDNF and GDNF: in vitro and in vivo studies. J. Neurosci. 28, 12622–12631. doi:10.1523/JNEUROSCI.0563-08.2008
Romanic, A. M., White, R. F., Arleth, A. J., Ohlstein, E. H., and Barone, F. C. (1998). Matrix metalloproteinase expression increases after cerebral focal ischemia in rats: inhibition of matrix metalloproteinase-9 reduces infarct size. Stroke 29, 1020–1030. doi:10.1161/01.STR.29.5.1020
Roudsari, L. C., Jeffs, S. E., Witt, A. S., Gill, B. J., and West, J. L. (2016). A 3D poly(ethylene glycol)-based tumor angiogenesis model to study the influence of vascular cells on lung tumor cell behavior. Sci. Rep. 6, 32726. doi:10.1038/srep32726
Ruan, G. P., Han, Y. B., Wang, T. H., Xing, Z. G., Zhu, X. B., Yao, X., et al. (2013). Comparative study among three different methods of bone marrow mesenchymal stem cell transplantation following cerebral infarction in rats. Neurol. Res. 35, 212–220. doi:10.1179/1743132812Y.0000000152
Ruan, L., Wang, B., Zhuge, Q., and Jin, K. (2015). Coupling of neurogenesis and angiogenesis after ischemic stroke. Brain Res. 1623, 166–173. doi:10.1016/j.brainres.2015.02.042
Rustenhoven, J., Aalderink, M., Scotter, E. L., Oldfield, R. L., Bergin, P. S., Mee, E. W., et al. (2016). TGF-beta1 regulates human brain pericyte inflammatory processes involved in neurovasculature function. J. Neuroinflammation 13, 37. doi:10.1186/s12974-016-0503-0
Salinas, C. N., and Anseth, K. S. (2008). The influence of the RGD peptide motif and its contextual presentation in PEG gels on human mesenchymal stem cell viability. J. Tissue Eng. Regen. Med. 2, 296–304. doi:10.1002/term.95
Seidlits, S. K., Khaing, Z. Z., Petersen, R. R., Nickels, J. D., Vanscoy, J. E., Shear, J. B., et al. (2010). The effects of hyaluronic acid hydrogels with tunable mechanical properties on neural progenitor cell differentiation. Biomaterials 31, 3930–3940. doi:10.1016/j.biomaterials.2010.01.125
Sharma, V., Ling, T. W., Rewell, S. S., Hare, D. L., Howells, D. W., Kourakis, A., et al. (2012). A novel population of α-smooth muscle actin-positive cells activated in a rat model of stroke: an analysis of the spatio-temporal distribution in response to ischemia. J Cereb Blood Flow Metab 32, 2055–2065. doi:10.1038/jcbfm.2012.107
Shen, Q., Wang, Y., Kokovay, E., Lin, G., Chuang, S.-M., Goderie, S. K., et al. (2008). Adult SVZ stem cells lie in a vascular niche: a quantitative analysis of niche cell-cell interactions. Cell Stem Cell 3, 289–300. doi:10.1016/j.stem.2008.07.026
Shoichet, M. S., Tator, C. H., Poon, P., Kang, C., and Douglas Baumann, M. (2007). Intrathecal drug delivery strategy is safe and efficacious for localized delivery to the spinal cord. Prog. Brain Res. 161, 385–392. doi:10.1016/S0079-6123(06)61027-3
Sobel, R. A. (1998). The extracellular matrix in multiple sclerosis lesions. J. Neuropathol. Exp. Neurol. 57, 205–217. doi:10.1097/00005072-199803000-00001
Sommer, C. J. (2017). Ischemic stroke: experimental models and reality. Acta Neuropathol. 133, 245–261. doi:10.1007/s00401-017-1667-0
Spassky, N., Merkle, F. T., Flames, N., Tramontin, A. D., Garcia-Verdugo, J. M., and Alvarez-Buylla, A. (2005). Adult ependymal cells are postmitotic and are derived from radial glial cells during embryogenesis. J. Neurosci. 25, 10–18. doi:10.1523/JNEUROSCI.1108-04.2005
Stewart, E., Kobayashi, N. R., Higgins, M. J., Quigley, A. F., Jamali, S., Moulton, S. E., et al. (2015). Electrical stimulation using conductive polymer polypyrrole promotes differentiation of human neural stem cells: a biocompatible platform for translational neural tissue engineering. Tissue Eng. Part C Methods 21, 385–393. doi:10.1089/ten.TEC.2014.0338
Stolp, H. B., and Molnár, Z. (2015). Neurogenic niches in the brain: help and hindrance of the barrier systems. Front. Neurosci. 9:20. doi:10.3389/fnins.2015.00020
Struzyna, L. A., Katiyar, K., and Cullen, D. K. (2014). Living scaffolds for neuroregeneration. Curr Opin Solid State Mater Sci 18, 308–318. doi:10.1016/j.cossms.2014.07.004
Tabata, H., Yoshinaga, S., and Nakajima, K. (2012). Cytoarchitecture of mouse and human subventricular zone in developing cerebral neocortex. Exp Brain Res 216, 161–168. doi:10.1007/s00221-011-2933-3
Tate, M. C., Shear, D. A., Hoffman, S. W., Stein, D. G., and Laplaca, M. C. (2001). Biocompatibility of methylcellulose-based constructs designed for intracerebral gelation following experimental traumatic brain injury. Biomaterials 22, 1113–1123. doi:10.1016/S0142-9612(00)00348-3
Taylor, S. J., Mcdonald, J. W., and Sakiyama-Elbert, S. E. (2004). Controlled release of neurotrophin-3 from fibrin gels for spinal cord injury. J Control Release 98, 281–294. doi:10.1016/j.jconrel.2004.05.003
Thomson, J. A., Itskovitz-Eldor, J., Shapiro, S. S., Waknitz, M. A., Swiergiel, J. J., Marshall, V. S., et al. (1998). Embryonic stem cell lines derived from human blastocysts. Science 282, 1145–1147. doi:10.1126/science.282.5391.1145
Trounson, A., and McDonald, C. (2015). Stem cell therapies in clinical trials: progress and challenges. Cell Stem Cell 17, 11–22. doi:10.1016/j.stem.2015.06.007
Vercruysse, K. P., and Prestwich, G. D. (1998). Hyaluronate derivatives in drug delivery. Crit. Rev. Ther. Drug Carrier Syst. 15, 513–555. doi:10.1615/CritRevTherDrugCarrierSyst.v15.i5.30
Vishwakarma, S. K., Bardia, A., Tiwari, S. K., Paspala, S. A. B., and Khan, A. A. (2014). Current concept in neural regeneration research: NSCs isolation, characterization and transplantation in various neurodegenerative diseases and stroke: a review. J. Adv. Res. 5, 277–294. doi:10.1016/j.jare.2013.04.005
Wakabayashi, K., Nagai, A., Sheikh, A. M., Shiota, Y., Narantuya, D., Watanabe, T., et al. (2010). Transplantation of human mesenchymal stem cells promotes functional improvement and increased expression of neurotrophic factors in a rat focal cerebral ischemia model. J. Neurosci. Res. 88, 1017–1025. doi:10.1002/jnr.22279
Weinstein, J. R., Koerner, I. P., and Möller, T. (2010). Microglia in ischemic brain injury. Future Neurol. 5, 227–246. doi:10.2217/fnl.10.1
Wells, M. R., Kraus, K., Batter, D. K., Blunt, D. G., Weremowitz, J., Lynch, S. E., et al. (1997). Gel matrix vehicles for growth factor application in nerve gap injuries repaired with tubes: a comparison of biomatrix, collagen, and methylcellulose. Exp. Neurol. 146, 395–402. doi:10.1006/exnr.1997.6543
Welser-Alves, J. V., Boroujerdi, A., and Milner, R. (2014). Isolation and culture of primary mouse brain endothelial cells. Methods Mol. Biol. 1135, 345–356. doi:10.1007/978-1-4939-0320-7_28
Williams, L. R., Longo, F. M., Powell, H. C., Lundborg, G., and Varon, S. (1983). Spatial-temporal progress of peripheral nerve regeneration within a silicone chamber: parameters for a bioassay. J. Comp. Neurol. 218, 460–470. doi:10.1002/cne.902180409
Wood, M. D., Borschel, G. H., and Sakiyama-Elbert, S. E. (2009). Controlled release of glial-derived neurotrophic factor from fibrin matrices containing an affinity-based delivery system. J. Biomed. Mater. Res. A. 89A, 909–918. doi:10.1002/jbm.a.32043
Yang, B., Migliati, E., Parsha, K., Schaar, K., Xi, X., Aronowski, J., et al. (2013). Intra-arterial delivery is not superior to intravenous delivery of autologous bone marrow mononuclear cells in acute ischemic stroke. Stroke 44, 3463–3472. doi:10.1161/STROKEAHA.111.000821
Yang, Z., Duan, H., Mo, L., Qiao, H., and Li, X. (2010). The effect of the dosage of NT-3/chitosan carriers on the proliferation and differentiation of neural stem cells. Biomaterials 31, 4846–4854. doi:10.1016/j.biomaterials.2010.02.015
Yemisci, M., Gursoy-Ozdemir, Y., Vural, A., Can, A., Topalkara, K., and Dalkara, T. (2009). Pericyte contraction induced by oxidative-nitrative stress impairs capillary reflow despite successful opening of an occluded cerebral artery. Nat. Med. 15, 1031–1037. doi:10.1038/nm.2022
Young, C. C., Brooks, K. J., Buchan, A. M., and Szele, F. G. (2011). Cellular and molecular determinants of stroke-induced changes in subventricular zone cell migration. Antioxid. Redox Signal. 14, 1877–1888. doi:10.1089/ars.2010.3435
Yu, H., Cao, B., Feng, M., Zhou, Q., Sun, X., Wu, S., et al. (2010). Combinated transplantation of neural stem cells and collagen type I promote functional recovery after cerebral ischemia in rats. Anat Rec (Hoboken) 293, 911–917. doi:10.1002/ar.20941
Zhang, M., Methot, D., Poppa, V., Fujio, Y., Walsh, K., and Murry, C. E. (2001). Cardiomyocyte grafting for cardiac repair: graft cell death and anti-death strategies. J. Mol. Cell. Cardiol. 33, 907–921. doi:10.1006/jmcc.2001.1367
Keywords: stem cell transplantation, cell therapy, tissue engineering, extracellular matrix, pericytes, endothelial cells
Citation: Matta R and Gonzalez AL (2018) Stroke Repair via Biomimicry of the Subventricular Zone. Front. Mater. 5:15. doi: 10.3389/fmats.2018.00015
Received: 07 December 2017; Accepted: 05 March 2018;
Published: 23 March 2018
Edited by:
Brendan Harley, University of Illinois at Urbana–Champaign, United StatesReviewed by:
Rachael Weiss Sirianni, St. Joseph’s Hospital and Medical Center, United StatesStephanie Michelle Willerth, University of Victoria, Canada
Justin Lee Brown, Pennsylvania State University, United States
Silviya Petrova Zustiak, Saint Louis University, United States
Copyright: © 2018 Matta and Gonzalez. This is an open-access article distributed under the terms of the Creative Commons Attribution License (CC BY). The use, distribution or reproduction in other forums is permitted, provided the original author(s) and the copyright owner are credited and that the original publication in this journal is cited, in accordance with accepted academic practice. No use, distribution or reproduction is permitted which does not comply with these terms.
*Correspondence: Anjelica L. Gonzalez, YW5qZWxpY2EuZ29uemFsZXpAeWFsZS5lZHU=