- 1Faculty of Printing, Packaging Engineering and Digital Media Technology, Xi'an University of Technology, Xi'an, China
- 2Shaanxi Provincial Key Laboratory of printing and Packaging Engineering, Xi'an University of Technology, Xi'an, China
In this work, a solid polymer electrolyte (SPE) doped with carbon nanotubes (CNTs) was used as a gas sensing material for a NO2 gas sensor. The electrolytes consisted of the ionic liquids (ILs) and CNTs, which were immobilized in a poly(vinylidene fluoride) (PVDF) matrix. The SPE membranes were characterized by scanning electron microscopy (SEM), X-ray photoelectron spectroscopy (XPS), Fourier-transform infrared spectroscopy (FTIR), and cyclic voltammetry (CV). The experimental results show that the addition of an appropriate amount of CNTs can appropriately improve the electrochemical performance of the SPE membrane. It was shown that NO2 gas sensors with an appropriate amount of CNTs added to their SPEs had a higher gas sensitivity than those with SPE containing no CNTs. When the mass ratio of PVDF, N-methyl-2-pyrrolidone (NMP), IL, and CNT was 1:4:1:0.08, the SPE showed the best gas sensitivity, and its sensitivity is 0.00275 V/ppm.
Introduction
From serious and widespread acid rain to photochemical smog events, NO2 is becoming more and more harmful to humans and nature. Therefore, the detection of NO2 gas is of great significance for environmental monitoring (Mizutani et al., 2005). There are many types of sensors available on the market today (Morón et al., 2015). These include, for example, electrochemical and optical sensors (Campbell and Collins, 1997; Bakker and Qin, 2006) and potentiometric sensors (Nakamaya, 2001). Electrochemical sensors, which use electrochemical principles to detect and measure a wide range of materials, are numerous and important (Nádherná et al., 2012). Electrochemical sensors are often used for the detection of gases, including NO2, NH3, H2O, and CO (Benvidi et al., 2017).
Sensitive materials are used for electrochemical sensors, including metal nanoparticles, carbon-based nanomaterials, inorganic compounds, and electrolytes. The sensitive materials that are currently widely used, and their performances are shown in Table 1. Electrolytes are often used as sensitive materials in gas detection. Electrochemical gas sensors can be divided into two groups: the first group comprises sensors with liquid-phase electrolytes, and the second includes solid-state electrolytes (Kuberský et al., 2013). Conventional electrochemical sensors have problems with liquid electrolyte leakage, which can affect the functioning of the sensor and shorten its life. Sensors with solid polymer electrolytes (SPE) do not suffer from electrolyte leakage (Varshney et al., 2003). Generally, SPEs are composed of polymers and a solid solution of inorganic salts embedded in polymer matrices. It is often advantageous to replace classical, liquid electrolytes in sensors with solids that exhibit ionic conductivity (Kitzelmann and Deprez, 1984). However, the poor compatibility of polymers with most inorganic salts often leads to low ionic conductivity (Yang et al., 2014). Solid electrolytes doped with ionic liquids (ILs) also exhibit low ionic conductivities. Therefore, it is necessary to further increase the conductivity of SPEs to meet the current needs of sensors (Ibrahim et al., 2012). In order to improve the conductivity of the SPEs, it has been proven that the addition of a conductive additive is one of the most effective methods (Moalleminejad and Chung, 2015). Carbon nanotubes (CNTs) have high conductivity, low resistivity, and high stability (Morishita et al., 2011) and can be used as additives. They are used in many applications, such as supercapacitors and solar energy, and are good substrates for manufacturing high-efficiency electronic devices (Cheng et al., 2011). CP composites of carbon nanomaterials represented by multi-walled carbon nanotubes have been successfully applied to electrochemical sensors, as shown in Table 2. Carbon nanomaterials exhibit good performance in sensors. Xiao et al. (2014) demonstrated the preparation of Poly[(vinylidenefluoride)-co-hexafluoropropylene]-based composite polymer electrolyte (CPE) membranes that were doped with various amounts of CNTs. It is clear that adding an appropriate amount of CNTs into the polymer matrix can enhance the ionic conductivity. An approach to the preparation of gas sensors is the use of SPE, in which the ionic liquid and carbon nanotubes are immobilized in the polymer matrix (Kuberský et al., 2015).
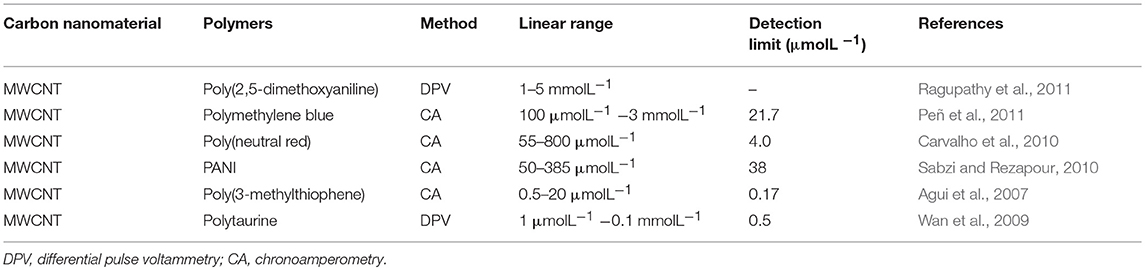
Table 2. CP composites containing carbon nanomaterials have been successfully applied to electrochemical sensing.
In the work reported here, CNTs were successfully used in SPE. Ionic liquids and CNTs were immobilized in a blend of poly(vinylidene fluoride) (PVDF) and 1-methyl-2-pyrrolidone (NMP). Different doping amounts of carbon nanotubes were chosen, and their physical and chemical properties were characterized using a number of techniques. When the ratio of PVDF:NMP:IL:CNTs was 1:4:1:0.08, the as-prepared SPEs presented excellent properties for practical applications.
Materials and Methods
Materials
Polyvinylidene fluoride was obtained from Shanghai Aichun Biological Technology Co., Ltd. 1-methyl-2-pyrrolidone was bought from Shanghai Zhanyun Chemical Co., Ltd. Carbon nanotube dispersion (the carbon tube content being 8 wt%) was purchased from Suzhou Carbon Technology Co., Ltd. 1-ethyl-3-methylimidazolium tetrafluoroborate was purchased from Shanghai Aichun Biological Technology Co., Ltd. All the purchased reagents were used without further purification. Polyethylene terephthalate was obtained from Bleher Folientechnik GmbH. Carbon paste conductive ink was bought from Ten Printing Equipment Technology Co., Ltd. Diazo photosensitive adhesive was bought from Shenzhen Jinfei Screen Printing Equipment Co., Ltd.
Printing of the Three-Electrode System
With the use of computer-aided design (CAD) software, an interdigitated three-electrode system was drawn to obtain a digital file. The obtained digital file was made into a positive film piece, and the film piece was closely attached to a screen plate coated with diazo photosensitive adhesive and irradiated with UV light for 180 s in a plate-printing machine. Then, the unirradiated photosensitive light was washed off with a water gun. An interdigitated three-electrode system was printed on a polyethyleneterephthalate (PET) transparent plastic film using a treated screen plate. Screen printing was used for printing the interdigital three-electrode system. Conductive carbon paste ink was used for the printing of the electrode.
Preparation of Carbon Nanotube-Doped Polymer Electrolyte
SPE without CNTs was prepared in the laboratory. The SPEs consisted of an IL:PVDF:NMP weight ratio of 1:1:4, which were stirred and dispersed for 20 min. The suspension prepared above was placed in a vacuum drying oven and heat-treated at 80°C to obtain a transparent plastic polymer, which was a solid electrolyte. PVDF was used as the polymer matrix and NMP as the solvent of the PVDF. Ionic liquids that had suitable properties for the formation of the SPE layer were immobilized in the PVDF matrix. Based on the solid electrolyte prepared in our laboratory, CNTs were incorporated, with the ratio of PVDF, NMP, and ionic liquids remaining basically the same with varied doping amounts of CNTs. Since the CNT dispersion contains NMP, the amount of NMP actually added will be reduced, and the total amount will remain unchanged. PVDF,1-ethyl-3-methylimidazolium tetrafluoroborate ([Emim][BF4]), NMP, and CNT dispersion were dispersed for 20 min at a certain mass ratio at room temperature until PVDF was completely dispersed, and a black suspension was obtained. The suspension prepared above was placed in a vacuum drying oven and heat-treated at 80°C to obtain a plastic polymer, which was a solid electrolyte. In order to find the optimum content of CNTs, four groups of experiments were carried out by doping different amounts CNTs in an SPE. The ratios of PVDF:NMP:IL:CNTs used were 1:4:1:0.16, 1:4:1:0.12, 1:4:1:0.08, and 1:4:1:0.04. The synthesis of CNT-doped solid electrolyte is shown in Figure 1A.
Sensor Fabrication
The solid electrolyte was uniformly coated on the printed interdigital three-electrode and allowed to stand at room temperature for 24 h. Then, the hydrophobic-gas-permeable membrane was covered on the working portion of the gas sensor coated with the solid electrolyte, that is, the NO2 sensor. The sensor preparation process is shown in Figure 1. The structure of the prepared gas sensor is shown in Figure 2A.
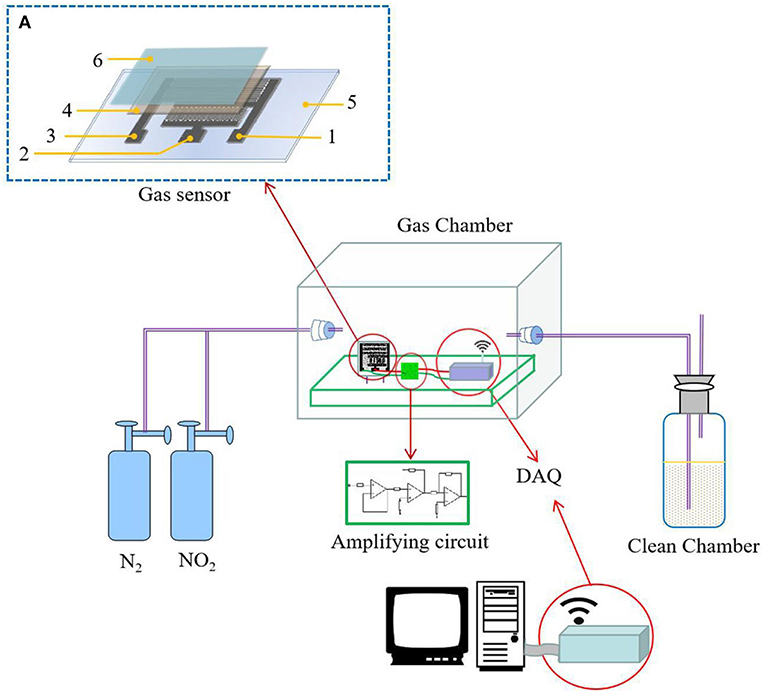
Figure 2. The measurement setup: (A) the structure of the prepared gas sensor [the numbers in the figure are marked as follows: (1) working electrode, (2) reference electrode, (3) auxiliary electrode, (4) solid polymer electrolyte (SPE), (5) PET film substrate, and (6) the hydrophobic-gas-permeable membrane].
Measurement Setup
The gas sensing test system consisted of a closed air chamber, a potentiostat, an amplifying circuit, a signal collecting device, and an exhaust-gas-collecting device. The closed glass container used was a self-contained closed air chamber. In the airtightness test, a constant potentiometer was used to provide a constant potential to the gas sensor. First, nitrogen gas was introduced to discharge the interfering gas, and then a quantitative concentration of NO2 was introduced. After the gas sensor detected the NO2, the data were collected. When collecting the data on the computer side, the obtained current signal was converted into a voltage signal. Then, the obtained voltage signal was amplified by an amplifying circuit, with the measurement setup shown in Figure 2.
Characterization Methods
Scanning electron microscopy (SEM, SU-8010, Hitachi, Japan) was applied to observe the morphologies of the SPE films. Fourier-transform infrared (FTIR) spectra were recorded on the sample/KBr-pressed pellets using Avatar380 (Thermo Fisher, USA) at room temperature. The chemical compositions of the solid electrolyte surfaces were determined by X-ray photoelectron spectroscopy (XPS) carried out on an AXIS ULTRA (KRATOS, UK) with an Al Kα X-ray source at 10 kV and 10 mA. Cyclic voltammetry (CV) was performed on an electrochemical work station (AUT86832), purchased from Metrohm China Ltd.
Results and Discussion
SEM Analysis
The surface structure of the solid electrolyte membrane after the addition of the ionic liquid is shown in Figures 3a,b. The surface of the solid electrolyte membrane which is not doped with CNTs is relatively flat, and there are small-sized pores on the surface. The pores on the surface of the membrane is not rich. Such pores have the capability to retain ionic liquid in them (Kumar and Hashmi, 2010). The surface structure of the solid electrolyte membrane after the addition of CNTs is shown in Figures 3c,d. As can be seen in Figure 3c, there are more pores on the surface. The bright features are caused by the electrostatically charged edges of the structure, and the high electrical conductivity of the carbon nanotubes may also cause this result (Chen et al., 2015). As shown in Figure 3d, it was found that the white traces of MWCNTs were still well-dispersed in the PVDF matrix (Wang et al., 2017). The solid electrolyte doped with carbon nanotubes (PVDF/IL/CNTs) film encapsulates the flowing ions and can be used directly as a solid thin film electrolyte without further processing.
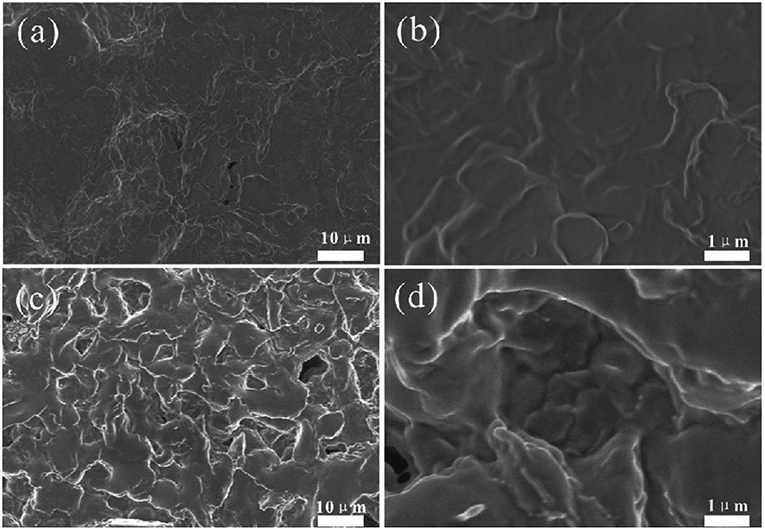
Figure 3. SEM images of PVDF/IL (a,b) and PVDF/IL/CNTs (1:4:1:0.08) (c,d) with different magnifications of 5000× (a,c) and 30,000× (b,d).
XPS Analysis
XPS was used to evaluate the atomic element information of the solid electrolyte membrane, as shown in Figures 4A,B. The undoped carbon nanotubes (PVDF/IL) and the CNT-doped solid electrolyte (PVDF/IL/CNTs) were composed of four elements, C, N, O, and F, and the spectrum shows prominent C1s and F1s peaks. The elemental analysis of the solid electrolyte membrane is shown in Table 3. The F element content of the CNT-doped solid electrolyte membrane increased, while the C element content decreased, and the F1s/C1s ratio increased from 0.11 to 0.47. This means that additional fluorine-containing polar groups were introduced after doping with the CNTs, which may be the result from solvents or residual organic groups in the commercially available CNT dispersions. The X-ray photoelectron spectrum of C, N, and O of undoped and doped CNTs samples are shown in Figures 5A–C, 6A–C, respectively. C1s core level spectra of undoped and doped CNTs samples are displayed in Figures 5A, 6A. The sample of undoped carbon nanotubes is C=C bond at 284.9 eV. At 286.5 eV is the C=N bond due to the ionic liquid. The oxygen-bonded carbon at 288.5 eV can be derived from the solvent during the preparation process. The peak at 290.4 eV corresponds to the carbon bonded in -CF2- (Chakradhar et al., 2014). The sample doped with CNTs is C=C bond at 284.3 eV. At 286 eV is the C=N bond due to the ionic liquid. This oxygen-bonded carbon at 288.3 eV can be derived from the solvent during the preparation process. The peak at 290.2 eV corresponds to the carbon bonded in -CF2- (Chakradhar et al., 2014). These peaks are consistent with the main chemical composition of the synthesized undoped CNTs samples. This result confirmed that IL, MWCNT and PVDF did not produce new binding energy after mixing. In the high-resolution spectra of N1s and O1s, as shown in Figures 5, 6, respectively. The core level spectra of N1s of the doped CNTs and undoped CNTs are located at 401.5 and 401.7 eV, respectively. This is attributed to the nitrogen portion of the C=N bond in the IL. The core level spectra peaks of the solid electrolyte O1s doped with CNTs and undoped CNTs are located at 531.7 and 532.3 eV, which is attributed to the oxygen portion of the C=O bond in the solvent. These peaks confirmed the binding energy shown in Figures 5A, 6A.
FTIR Analysis
FTIR was used to characterize the functional groups of the solid electrolyte membrane. It can be seen in Figure 7B that the broad peak around 3,400 cm−1 corresponds to the O-H stretching vibration of water (Nawanil et al., 2013). The peak at 3,078 cm−1 is derived from the tensile vibration of C-H on the imidazole ring. The peaks at 2,078 and 1,408 cm−1 are attributed to the tensile vibration of C=N and C-N in the IL, respectively. The peak at 1,637 cm−1 is the tensile vibration peak of C=C, which may also be the vibration peak of C=O, attributed to the NMP solvent. It can be seen in Figure 7A that the peak at 3,624 cm−1 is attributed to the tensile vibration of O-H, and the peaks at 3,164 and 3,123 cm−1 are the stretching vibration absorption peak of C-H on the imidazole ring. The peak at 1,668 cm−1 is related to the C=C extension of the CNTs (Ramesh et al., 2006). The peaks at 1,573 and 1,403 cm−1 are attributed to the tensile vibrations of C=N and C-N of [Emim][BF4]. The peak of 1,454 cm−1 corresponds to the vibration of either -CH3 or -CH2. The peak at 1,049 cm−1 is a tensile vibration of C-F derived from PVDF. We found that in the infrared of the doped IL and CNTs solid electrolyte, a prominent peak corresponding to IL was observed, indicating the presence of IL on the surface of the MWCNTs and possible interaction with the MWCNTs. The peak at 1,230 cm−1 corresponds to the antisymmetric stretching of CF3. The peak at 1,168 cm−1 is the characteristic imidazole peak (Sharma et al., 2014), which confirms the presence of IL. In summary, it can be seen from the peaks in Figures 7A,B that the carbon nanotubes and the ionic liquid have a certain interaction and are filled into the PVDF.
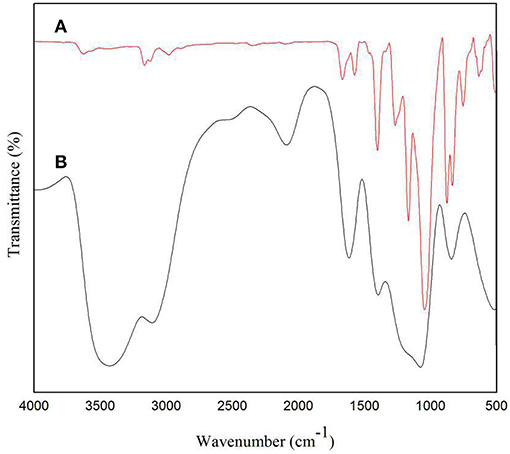
Figure 7. FTIR spectra of (A) solid electrolyte doped with carbon nanotubes and (B) solid electrolyte without carbon nanotubes.
CV Analysis
In previous studies of energy storage devices, CV was the most reasonable method for testing and analyzing the behavior of material capacitance (Kumar et al., 2012). Figure 8 shows a cyclic voltammetry characteristic curve after doping with different amounts of CNTs. The samples were scanned at a high rate of 80 mV/s. The electrochemical properties of PVDF/IL/CNTs electrolyte membranes were investigated using a CV test between the potential ranges of −1.5 and 1.5. It is well-known that as the closed region of the CV curve is larger, the specific capacitance of the sample is expected to be larger (Xing et al., 2006). As can be seen in the figure, the solid electrolyte membrane having a ratio of 1:4:1:0.08 in Figure 8B showed better capacitance than the other samples. The closed area under the CV curve of Figures 8C,D is quite small, indicating a very low specific capacitance. This may be due to an increase in the relative content of the CNTs, resulting in a decrease in dispersibility in the dispersion, affecting the transport of electrons. Therefore, we conclude that adding an appropriate amount of CNTs is beneficial to increase the capacitance. A solid electrolyte membrane having a doping ratio of 1:4:1:0.08 showed good sensitivity in later gas sensor applications.
Effect of the Doping Amount of Carbon Nanotubes
The detection of nitrogen dioxide by a current sensor having a polymer electrolyte is relatively well-known, and a specific redox reaction occurs on the electrode (Altšmíd et al., 2015). The focus of this work is on systematic studies of the four doping amounts of CNTs described above. All sensors with different amounts of CNTs inserted into the SPE were prepared according to the procedures described in the sections above. Each SPE layer with a particular carbon nanotube concentration was formed under the same thermal conditions (80°C) to eliminate the possible effects of thermal conditions on sensor characteristics. Sensitivity was calculated from the calibration curve of a particular sensor (Figure 9). As can be seen from Figure 9, among all other ratios, the gas sensitivity of the ratio of 1:4:1:0.08 is clearly the best, and its sensitivity is 0.00275 V/ppm. Therefore, we conclude that adding a proper amount of CNTs can improve the gas sensor sensitivity.
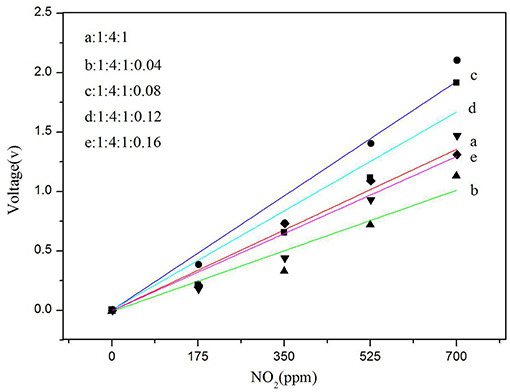
Figure 9. Dependence of sensor voltage on NO2 concentration for different MWCNT (Sample b, c, d, and e) contents in SPE and solid electrolyte without MWCNT as a blank control (sample a).
Conclusions
A novel PVDF/IL/CNTs SPE for an electrochemical NO2 sensor was successfully prepared. Electron microscopy of carbon nanotube-doped solid electrolytes showed that MWNT and IL were well-dispersed in the matrix, which has important practical significance for the preparation of solid electrolytes. A uniform SPE film was prepared by adjusting the CNT contents. The PVDF/NMP/IL/CNTs (1:4:1:0.08) SPE film has a good wide electrochemical window (~3.0 V), and the solid electrolyte membrane with a ratio of 1:4:1:0.08 has good capacitance. In the initial application of the NO2 sensor, a solid electrolyte application with a ratio of 1:4:1:0.08 exhibited high sensitivity in the sensor, and its sensitivity is 0.00275 V/ppm. Further improvements to the electrochemical NO2 sensor can be expected by optimizing the preparation of the solid electrolyte.
Author Contributions
RL proposed the idea and guided the experiment. QL did the experiment with the help of YC. BD contributed to data analysis. SZ gave some suggestions.
Funding
This work was supported in part by NSF of the Science and Technology Department of Shaanxi Province under Grant No. 2019JM-122, Doctoral Research Initiation Fund of Xi'an University of Technology under Grant No. 108-451119007, NSF of the Science and Technology Department of Shaanxi Province under Grant No. 2018JQ5100, NSF of the Key Laboratory of Shaanxi Provincial Department of Education under Grant No. 15JS075, and Shaanxi Collaborative Innovation Center of Green Intelligent Printing and Packaging.
Conflict of Interest Statement
The authors declare that the research was conducted in the absence of any commercial or financial relationships that could be construed as a potential conflict of interest.
Acknowledgments
We thank LetPub for its linguistic assistance.
References
Agui, L., Penafarfal, C., Yanezsedeno, P., and Pingarron, J. (2007). Poly-(3-methylthiophene)/carbon nanotubes hybrid composite-modified electrodes. Electrochim. Acta 52,7946–7952. doi: 10.1016/j.electacta.2007.06.051
Altšmíd, J., Syrový, T., Syrová, L., Kuberský, P., Hamáček, A., Zmeškal, O., et al. (2015). Ionic liquid based polymer electrolytes for NO2 electrochemical sensors. Mater. Sci. 21, 415–418. doi: 10.5755/j01.ms.21.3.737
Bakker, E., and Qin, Y. (2006). Electrochemical sensors. Anal. Chem. 78:3965. doi: 10.1021/ac060637m
Benvidi, A., Nikmanesh, M., Tezerjani, M. D., Jahanbani, S., Abdollahi, M., Akbariet, A., et al. (2017). A comparative study of various electrochemical sensors forhydrazinedetection based on imidazole derivative anddifferent nano-materials ofMCM-41, RGO and MWCNTs: using net analyte signal (NAS)for simultaneous determination of hydrazine and phenol. J. Electroanal. Chem. 787, 145–157. doi: 10.1016/j.jelechem.2017.01.006
Campbell, D., and Collins, R. A. (1997). A study of the interaction between nitrogen dioxide and lead phthalocyanine using electrical conduction and opticalabsorption. Thin Solid Films 295, 277–282. doi: 10.1016/S0040-6090(96)09403-5
Carvalho, R. C., Gouveia-Caridade, C., and Brett, C. M. A. (2010). Glassy carbon electrodes modified by multiwalled carbon nanotubes and poly(neutral red): a comparative study of different brands and application to electrocatalytic ascorbate determination. Anal. Bioanal. Chem. 398, 1675–1685. doi: 10.1007/s00216-010-3966-3
Chakradhar, R. P. S., Prasad, G., Bera, P., and Anandan, C. (2014). Stable superhydrophobic coatings using pvdf–mwcnt nanocomposite. Appl. Surf. Sci. 301, 208–215. doi: 10.1016/j.apsusc.2014.02.044
Chen, G. X., Zhang, S., Zhou, Z., and Li, Q. (2015). Dielectric properties of poly(vinylidene fluoride) composites based on bucky gels of carbon nanotubes with ionic liquids. Polymer Compos. 36, 94–101. doi: 10.1002/pc.22917
Cheng, Q., Tang, J., Ma, J., Zhang, H., Shinyaa, N., and Qin, L. C. (2011). Graphene and carbon nanotube composite electrodes for supercapacitors with ultra-high energy density. Phys. Chem. Chem. Phys. 13, 17615–17624. doi: 10.1039/c1cp21910c
Ibrahim, S., Ahmad, R., and Johan, M. R. (2012). Conductivity and optical studies of plasticized solid polymer electrolytes doped with carbon nanotube. J. Luminescence 132,147–152. doi: 10.1016/j.jlumin.2011.08.004
Jacek, G., Kloskowski, A., Chrzanowski, W., Stepnowski, P., and Namiesnik, J. (2016). Application of ionic liquids in amperometric gas sensors. Crit. Rev. Anal. Chem. 46, 122–138. doi: 10.1080/10408347.2014.989957
Kitzelmann, D., and Deprez, J. (1984). Electro-chemicalsensor for the detection of reducing gases, in particular carbon monoxide, hydrazine and hydrogen in air. Int. J. Hydrogen Energy 9:636. doi: 10.1016/0360-3199(84)90256-8
Kuberský, P., Altšmíd, J., Hamáček, A., Nešpurek, S., and Zmeškal, O. (2015). An electrochemical NO2 sensor based on ionic liquid: influence of the morphology of the polymer electrolyte on sensor sensitivity. Sensors 15, 28421–28434. doi: 10.3390/s151128421
Kuberský, P., Hamáček, A., Nešpurek, S., Soukup, R., and Vik, R. (2013). Effect of the geometry of a working electrode on the behavior of a planar amperometric NO2 sensor based on solid polymer electrolyte. Sens. Actuat. B Chem. 187, 546–552. doi: 10.1016/j.snb.2013.03.081
Kumar, D., and Hashmi, S. A. (2010). Ionic liquid based sodium ion conducting gel polymer electrolytes. Solid State Ion. Diffus. React. 181, 416–423. doi: 10.1016/j.ssi.2010.01.025
Kumar, Y., Pandey, G. P., and Hashmi, S. A. (2012). Gel polymer electrolyte based electrical double layer capacitors: comparative study with multiwalled carbon nanotubes and activated carbon electrodes. J. Phys. Chem. C 116, 26118–26127. doi: 10.1021/jp305128z
Mizutani, Y., Matsuda, H., Ishiji, T., Furuya, N., and Takahashi, K. (2005). Improvementof electrochemical NO2, sensor by use of carbon–fluorocarbon gas permeable electrode. Sens. Actuat. B Chem. 108, 815–819. doi: 10.1016/j.snb.2004.11.084
Moalleminejad, M., and Chung, D. D. L. (2015). Dielectric constant and electrical conductivity of carbon black as an electrically conductive additive in a manganese-dioxide electrochemical electrode, and their dependence on electrolyte permeation. Carbon 91, 76–87. doi: 10.1016/j.carbon.2015.04.047
Morishita, T., Matsushita, M., Katagiri, Y., and Fukumori, K. (2011). A novel morphological model for carbon nanotube/polymer composites having high thermal conductivity and electrical insulation. J. Mater. Chem. 21, 5610–5614. doi: 10.1039/C0JM04007J
Morón, C., Cabrera, C., Morón, A., García, A., and González, M. (2015). Magnetic sensors based on amorphous ferromagnetic materials: a review. Sensors 15, 28340–28366. doi: 10.3390/s151128340
Nádherná, M., Opekar, F., Reiter, J., and Štulík, K. (2012). A planar, solid-state amperometric sensor for nitrogen dioxide, employing an ionic liquid electrolyte contained in a polymeric matrix. Sens. Actuat. B Chem. 161, 811–817. doi: 10.1016/j.snb.2011.11.037
Nakamaya, S. (2001). Potentiometric NO2, gas sensor using LiRESiO 4, (RE=Nd and Sm). Ceramics Int. 27, 191–194. doi: 10.1016/S0272-8842(00)00062-6
Nawanil, C., Rerkratn, A., Seeharaj, P., and Vittayakorn, N. (2013). Characterization and dielectric properties of nanocomposite made of lead zirconate nanofibers and polyvinylidene fluoride improved with carbon nanotubes. Integrat. Ferroelectrics 149, 18–24. doi: 10.1080/10584587.2013.852904
Peñ, A. R. C., Bertotti, M., and Brett, C. M. A. (2011). Methylene blue/multiwall carbon nanotube modified electrode for the amperometric determination of hydrogen peroxide. Electroanalysis 23, 2290–2296. doi: 10.1002/elan.201100324
Ragupathy, D., Park, J. J., Lee, S. C., Kim, J. C., Gomathi, P., Kim, M. K., et al. (2011). Electrochemical grafting of poly(2,5-dimethoxy aniline) onto multiwalled carbon nanotubes nanocomposite modified electrode and electrocatalytic oxidation of ascorbic acid. Macromol. Res. 19, 764–769. doi: 10.1007/s13233-011-0802-3
Ramesh, B. P., Blau, W. J., Tyagi, P. K., Misra, D. S., Ali, N., Gracio, J., et al. (2006). Thermogravimetric analysis of cobalt-filled carbon nanotubes deposited by chemical vapour deposition. Thin Solid Films 494, 128–132. doi: 10.1016/j.tsf.2005.08.220
Rehman, A., and Zeng, X. (2012). Ionic liquids as green solvents and electrolytes for robustchemical sensor development. Accounts Chem. Res. 45, 1667–1677. doi: 10.1021/ar200330v
Sabzi, E., and Rezapour, K. (2010). Polyaniline-multi-wall-carbon nanotube nanocomposites as a dopamine sensor. J. Serbian Chem. Soc. 75,537–549. doi: 10.2298/JSC090527012S
Sarkar, S., Guibal, E., Quignard, F., and Sengupta, K. A. (2012). Polymer-supported metals and metal oxide nanoparticles: synthesis, characterization, and applications. J. Nanopart. Res. 14:715. doi: 10.1007/s11051-011-0715-2
Sharma, M., Sharma, S., Abraham, J., Thomas, S., Madras, G., and Bose, S. (2014). Flexible emi shielding materials derived by melt blending pvdf and ionic liquid modified mwnts. Mater. Res. Express 1:035003. doi: 10.1088/2053-1591/1/3/035003
Varshney, P., Deepa, M., Agnihotry, S. A., and Ho, K. C (2003). Photo-polymerized films of lithium ion conducting solid polymer electrolyte for electrochromic windows (ecws). Solar Energy Mater. Solar Cells 79, 449–458. doi: 10.1016/S0927-0248(03)00020-5
Wan, Q., Wang, X., Yu, F., Wang, X., and Yang, N. (2009). Poly(taurine)/mwnt-modified glassy carbon electrodes for the detection of acetaminophen. J. Appl. Electrochem. 39:6.785–790. doi: 10.1007/s10800-008-9721-1
Wang, P., Xu, P., Zhou, Y., Yang, Y., and Ding, Y. (2017). Effect of MWCNTs and P[MMA-IL] on the crystallization and dielectric behavior of pvdf composites. Eur. Polymer J. 2017:003. doi: 10.1016/j.eurpolymj.2017.12.003
Xiao, W., Li, X., Guo, H., Wang, Z., Li, Y, and Yang, B, (2014). Preparation and physicochemical performances of poly[(vinylidenefluoride)-co-hexafluoropropylene]-based composite polymer electrolytes doped with modified carbon nanotubes PolymerInternational 63, 307–314. doi: 10.1002/pi.4506
Xing, W., Qiao, S. Z., Ding, R. G., Li, F., Lu, G. Q., Yan, Z. F., et al. (2006). Superior electric double layer capacitors using ordered mesoporous carbons. Carbon 44, 216–224. doi: 10.1016/j.carbon.2005.07.029
Yang, L., Hu, J. Y., Lei, G., and Liu, H. T. (2014). Ionic liquid-gelled polyvinylidene fluoride/polyvinyl acetate polymer electrolytefor solidsupercapacitor. Chem. Eng. J. 258, 320–326. doi: 10.1016/j.cej.2014.05.149
Keywords: poly(vinylidene fluoride), ionic liquids, solid electrolyte, carbon nanotubes, NO2 gas sensor
Citation: Luo R, Li Q, Du B, Zhou S and Chen Y (2019) Preparation and Characterization of Solid Electrolyte Doped With Carbon Nanotubes and its Preliminary Application in NO2 Gas Sensors. Front. Mater. 6:113. doi: 10.3389/fmats.2019.00113
Received: 08 November 2018; Accepted: 30 April 2019;
Published: 29 May 2019.
Edited by:
John Zhanhu Guo, Tickle College of Engineering, University of Tennessee, Knoxville, United StatesReviewed by:
Jose Antonio Gomez-Tejedor, Universitat Politècnica de València, SpainKrisztian Kordas, University of Oulu, Finland
Copyright © 2019 Luo, Li, Du, Zhou and Chen. This is an open-access article distributed under the terms of the Creative Commons Attribution License (CC BY). The use, distribution or reproduction in other forums is permitted, provided the original author(s) and the copyright owner(s) are credited and that the original publication in this journal is cited, in accordance with accepted academic practice. No use, distribution or reproduction is permitted which does not comply with these terms.
*Correspondence: Bin Du, ZHViaW5AeGF1dC5lZHUuY24=