- 1Otto Schott Institute of Materials Research, Friedrich Schiller University Jena, Jena, Germany
- 2State Key Laboratory of Luminescent Materials and Devices, Guangdong Provincial Key Laboratory of Fiber Laser Materials and Applied Techniques, and Institute of Optical Communication Materials, South China University of Technology, Guangzhou, China
- 3Leibniz Institute of Photonic Technology, Jena, Germany
Pressure-assisted melt filling (PAMF) of pre-fabricated micro-capillaries has been proven an effective way of fabricating hybrid optical fiber (HOF) from unusual combinations of materials. Here, we extend the applicability of PAMF to multi-anionic fluoride-sulfophosphate (FPS) glasses. FPS glasses provide extended transmission windows and high solubility for various transition metal (TM) and rare earth (RE) ion species. Using PAMF for fabricating FPS/silica HOFs can therefore act as a platform for a broad variety of optically active fiber devices. For the present demonstration purposes, we selected Cr3+- and Mn2+-doped FPS. For both glasses, we demonstrate how the spectral characteristics of the bulk material persist also in the HOF. Using a double-core fiber structure in which waveguiding is conducted in a primary GeO2-SiO2 core, mode coupling to the secondary FPS-filled core allows one to exploit the optical activity of the doped FPS glass even when the intrinsic optical loss is high.
Introduction
Fiber lasers are widely used in fields such as materials processing, sensing or, e.g., medical surgery (Jackson, 2012). In order to further extend their ranges of application, fiber materials that operate in different wavelength regimes are continuously being explored, for example, spanning the infrared spectral range for biomedical sensing, or the ultraviolet range for short-wavelength laser output. As an alternative to rare earth (RE) activator ions with mostly f –f (or sometimes f –d) electronic transitions, transition metal (TM) ions have also attracted great attention, primarily due to the electronic d–d transitions that offer significantly enhanced operation bandwidth and, in principle, tunability through adjusting the host material composition (Moncorge, 2017). TM species such as chromium (Yeh et al., 2012), nickel (Samson et al., 2002), or manganese (Ehrt, 2013) in crystalline, glassy, or glass ceramic matrix have been considered for this purpose. As a prominent example, chromium-doped glass fiber was reported for next-generation optical communication systems (Yeh et al., 2012). Efficient laser output was also obtained in the visible to near-infrared spectral range using bismuth-doped glasses as fiber materials (Dianov, 2012). Divalent manganese has drawn attention for its particular combination of tunability in various coordination sites and extended emission lifetime (Da et al., 2010a; Chen et al., 2016; Song et al., 2016).
A major challenge in all these approaches is to find an appropriate combination of matrix material, dopant species, and fiber manufacturing process. Such implementation approaches are currently being used for incorporating various materials into glass fibers, including high-pressure chemical vapor deposition (HPCVD), direct fiber drawing, the molten core method, and melt-in-tube technologies (Ballato and Peacock, 2018). When integrating the multicomponent glasses, crystals, or metals into the silica capillary, however, among other issues, unfavorable combinations of viscosity–temperature behavior and liquidus temperature typically complicate device fabrication. The fabrication of hybrid optical fibers (HOFs) offers some interesting alternatives not only in material processing but also in terms of unconventional combinations of core and cladding materials (Sorin et al., 2007; Schmidt et al., 2016). For example, HOFs may integrate glasses (Jain et al., 2016), crystals (Dragic et al., 2012), semiconductors (Peng et al., 2017), noble-metal nanowires (Tuniz et al., 2017), liquid solutions (Chemnitz et al., 2017), or even gases (Benabid et al., 2002). Current techniques for their fabrication rely mostly on advanced thermal drawing (Cheng et al., 2014; Tuniz et al., 2017) and liquid infiltration of pre-fabricated hollow or microstructured fiber (Da et al., 2010b, 2011; Schmidt et al., 2011; Jain et al., 2016; Tuniz et al., 2017). In particular, for pressure-assisted melt filling (PAMF) of capillary fiber, very high flexibility in terms of material choices and processing conditions was demonstrated. Another key benefit of this approach is that only a small amount of the glass that is to be filled into the capillary is needed. Furthermore, in comparison to other techniques such as rod-in-tube, PAMF avoids extensive pre-processing such as drilling and/or polishing of preforms. Since the fiber core material is encapsulated within the silica capillary, it remains protected from environmental influences, which is important for hygroscopic or toxic glasses acting as the fiber core. In principle, the PAMF technique enables the broadest variety of material combinations. Its major prerequisite and limitation is that the fiber core material needs to provide a low enough viscosity (e.g., <10 Pa·s) at the filling temperature, whereby the filling temperature must be low enough to avoid softening or extensive chemical interaction with the capillary materials (e.g., <1,200°C for silica). Although both PAMF and HPCVD require processing at elevated pressure, they are inherently different. In the HPCVD, the precursor mixture of raw materials is mechanically pumped into the silica capillary to obtain a high pressure. The deposition is continued until the desired fiber length is fabricated. PAMF, on the other hand, does not involve a gas-phase deposition process. Instead, the fiber core is filled with a pre-conditioned (and homogeneous) glass melt. Using PAMF, glass–glass HOFs have been prepared from combinations of a silica cladding with a chalcogenide (Wang et al., 2015), tellurite (Da et al., 2011), or phosphate (Chemnitz et al., 2016) glass. In particular, the latter holds promise for preparing optically active devices: besides their extended transmission windows in both the UV and IR spectral ranges and distinct dielectric properties, phosphate glasses offer particularly high solubility for RE, TM, or even noble-metal activator species [e.g., Nd3+ (Hu et al., 2017), Er3+ (Xu et al., 2010), Cr3+ (Landry et al., 1967), Mn2+ (Ehrt, 2015), Co2+ (Griebenow et al., 2017), or Ag+ (Thieme et al., 2015)]. As another trait, phosphate glasses exhibit high solubility for secondary anion species such as halides or oxoanions (Le et al., 2017; Wang et al., 2017; Rodrigues et al., 2019). This enables further tailoring of the material's UV-NIR transparency window, its refractive index, partial dispersion, phonon energy and solubility of dopant species, or, e.g., optical non-linearity (Topfer et al., 2000). Such poly-anionic glasses have recently been developed in the fluoride-sulfophosphate (FPS) chemical regime, where extraordinary combinations of highly polarizable sulfate ions, phosphate, and di-phosphate groups and the more localized fluoride ligands were achieved in surprisingly stable glass matrices (Le et al., 2017; Wang et al., 2017). In order to exploit these materials for optical fiber application, we now combine them with PAMF processing of HOFs, fabricating FPS/silica HOFs. In the present work, we explore how the integration of FPS glasses into silica capillaries alters the material's optical properties and how fiber optical waveguides can be obtained. This addresses the potential use of FPS glasses as active media in fiber lasers and other fiber-integrated devices. As a proof of principle, we demonstrate FPS/silica HOFs activated with Mn2+ and Cr3+, respectively. These specific TM species have been selected because of their tailorable spectroscopic properties at varying host composition and dopant concentration, and because of their particularly broad emission spectra toward broadband light sources.
Experimental Section
Exemplary glass compositions were selected in accordance with a previous analysis of the glass-forming ability of FPS glasses (Le et al., 2017). We chose three different bulk glasses with varying sulfate content, 85(MgF2, CaF2, SrF2, AlF3)-(15-x)Sr(PO3)2-xSrSO4 [mol%, with x = 5, 10, 15; denoted FP(15-x)S(x)]. For demonstrating optical activity, additional glasses were prepared from these compositions by doping with 0.05 mol% of Cr2O3 and 2 mol% of MnO2, respectively. Details on the glass preparation process (including the occurrence of evaporation losses and potential differences between nominal and actual composition) are reported elsewhere (Le et al., 2017). Batches of 100 g were melted in a muffle furnace (using Pt crucibles at 1,000–1,100°C) for 2 h, then cooled to 830–950°C and poured into a preheated graphite mold. Following this, annealing was conducted for another 2 h at 440–510°C, with subsequent cooling to room temperature at a rate of ~2 K/min. Differing from this procedure, the compositions with poor glass-forming ability (FP5S10 and FP0S15) were rapidly quenched by pouring the melt onto cold copper plates and immediately pressing with a copper stamp. Subsequent annealing of these samples was done through the same procedure as above. The temperature difference ΔT = Tx – Tg of the onset temperature of crystallization Tx and the glass transition temperature Tg was taken as a measure of glass stability. Values for FPS10S5, FPS5S10, and FPS0S15 were adopted from a previous study (Wang et al., 2017), i.e., >150, 133, and 78°C, respectively. Since FPS10S5 exhibits the highest ΔT, it was chosen as the focus composition for the present study, in particular, in the following fiber preparation process.
Hybrid fiber was manufactured using the PAMF method as shown schematically in Figure 1. We used the FP10S5 undoped glass, FP10S5:0.05Cr and FP10S5:2Mn, respectively, as the core materials of different FPS/silica HOFs. Manually drawn strands of these FPS glasses with diameters of <100 μm were placed into silica auxiliary capillaries. These were then spliced to the target capillaries and heated on a hot plate so that the FPS filament was melted. Using argon as the transmission medium, the melt was then pressurized so as to infiltrate the target capillary. The filling temperature, filling pressure, and filling time were selected in accordance with previous studies through numerical simulation and experimental optimization (Da et al., 2010b, 2011). For the target capillary, we employed three kinds of silica capillaries that were fabricated by regular fiber drawing from MCVD preforms. These included single-core silica capillaries with channel diameters of 2.5 and 8 μm, respectively, and a modified graded index fiber (MGIF) with a double-core structure. The latter consisted of two parallel channels in which a GeO2-SiO2-doped core with a diameter of 1.9 μm (GeO2 peak doping level ~11 mol%) and a hollow channel of 2.28 μm are separated by a lateral distance of 3.9 μm (center-to-center). Here, the hollow core was filled with FPS glass. For the single-core capillary with a core diameter of 2.5 μm, PAMF was finally conducted at 750°C and 50 bar for 70 min, while for the single-core capillary with a core diameter of 8 μm, PAMF was conducted at 750°C and 10 bar for 35 min. Both hybrid step index fibers (SIFs) were obtained with typical filling lengths of ~10 cm, in the following referred to as FPS–SIF. For the double-core fiber, the same conditions as for the 2.5-μm capillary were employed (referred to as FPS–MGIF).
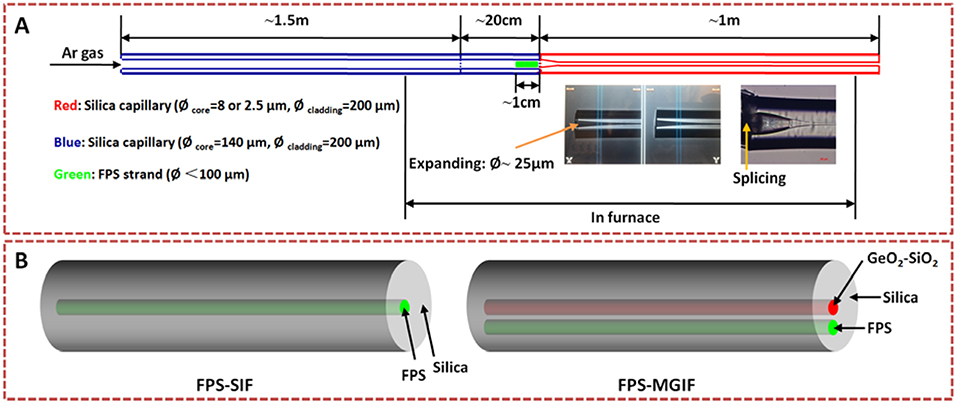
Figure 1. (A) Schematic of pressure-assisted melt filling (PAMF) for the fabrication of hybrid silica/FPS fiber and (B) two types of hybrid fibers.
UV–Vis–NIR absorption spectra of the bulk glasses were recorded on a double-beam spectrophotometer (Agilent) with a spectral resolution of 1.0 nm. Photoluminescence excitation and emission spectra as well as decay curves were recorded on a high-resolution spectrofluorometer (Horiba Jobin-Yvon), using a continuous-wave 450-W Xe lamp as excitation source and a Hamamatsu R2658P photomultiplier tube for detection. Thermomechanical analyses were conducted on a thermomechanical analyzer (TMA, Netzsch), employing a heating rate of 5 K/min under inert N2 atmosphere. The elastic properties were characterized by ultrasonic echography on co-planar, optically polished glass plates. The longitudinal and transversal wave velocities were determined with a piezoelectric transducer operating at frequencies of 8–12 MHz (Echometer 1077, Karl Deutsch GmbH & Co KG). For analyzing the filling process, side images were recorded on fiber sections (end, middle) by digital microscopy (Nikon). Furthermore, micro-Raman spectra were also collected on these side views (Renishaw) using a 785-nm laser for excitation. For the doped compositions, the local emission spectra of the FPS/silica hybrid fiber were collected with the same Raman microscope, now using a 514-nm laser for excitation. A Raman confocal diameter of about 1 μm and probe powers of 5 and 50 μW were adopted for the bulk glass and fiber, respectively.
Finally, the transmission spectra of FPS/silica hybrid fiber were collected in the visible range using the cut-back method. The transmission setup is illustrated in Figure 2. A broadband light source (supercontinuum, 450 nm to 2.4 μm) was coupled into the FPS–MGIF sample using a 50× microscope objective after passing through a polarizer oriented along the connection line between the two cores. The transmitted light was out-coupled with a 20× objective. An out-coupling objective with relatively small numerical aperture was used to collect only the mode coming from the GeO2-SiO2-doped core. The light was then transmitted through a multimode fiber (MMF) to the optical spectrum analyzer (OSA). A pinhole was used to block the light that was guided through the cladding. Higher-order modes from the FPS core with relatively high numerical apertures were not collected. As shown in Figure 2, the measured FPS–MGIF sample consists of filled and unfilled sections. There are two purposes to introduce the unfilled section (length of ~19 cm): (i) it ensures a good modal overlap at the interface of filled and unfilled sections to guarantee optimal in-coupling, and (ii) it avoids optically induced damage of the FPS end face (Jain et al., 2016). Additionally, the unfilled section was inserted into liquid with a refractive index of 1.52, which further improves the ability to remove the cladding light.

Figure 2. Schematic of the transmission setup used for the optical characterization of fiber samples (OSA, optical spectrum analyzer; MMF, multimode fiber; core diameter, 50 μm). The arrows indicate the direction of the light beam.
All measurements were performed at room temperature.
The refractive indices of the FPS glasses were determined with a Pulfrich refractometer on samples with a thickness of 5 mm. For quantifying the wavelength dependence, we used the Sellmeier equation (Sardar et al., 2003),
where S and λ0 are empirical fitting parameters.
All the reported fiber samples are listed in Table 1. Fibers “1” and “2” were used for microscopic analysis. The reported Raman spectra were collected on Fiber “2” and compared to those of the bulk FP10S5 glass. Fibers “3” and “4” were used for the analysis of the luminescent properties. The attenuation analyses were conducted on Fiber “5.”
Results and Discussion
Properties of Bulk FPS
The physical properties of silica and FP10S5 glasses as used for PAMF are summarized in Table 2. Properties of the silica glass are provided from the manufacturer (Heraeus Suprasil®-Family, Spectrosil®). From Table 2, we find that notable deviations occur in the thermal properties of silica and FPS glasses. The FPS glass has higher density, refractive index, thermal expansion coefficient, elastic modulus, and Poisson ratio than silica. Its softening temperature, Tg, and hardness are lower. These large deviations result in general incompatibility of the two glasses in classical routes for HOF manufacture, for example, using the rod-in-tube technique. This highlights the most important advantage of the PAMF method, i.e., its wide flexibility in terms of material combinations.
Figure 3 shows the absorption, excitation, emission spectra, and lifetime of chromium-doped FPS bulk glasses with various sulfate contents. The key spectroscopic parameters are summarized in Table 3. Obviously, changing glass composition leads to changes in the positions and intensities of the relevant absorption bands of the pertinent Cr species. Chromium exhibits three major absorption peaks originating from Cr3+ and Cr6+ ions (Wang et al., 2017). Due to potential crystallization in the FP0S15:0.05Cr sample, minor amounts of Cr4+ may also exist in this glass. In the cast FP10S5:0.05Cr glass, there are two broad absorption bands at 439 and 640 nm, mainly originating from the Cr3+:4A2 → 4T1 and Cr3+:4A2 → 4T2 transitions. In the glass composition with high contents of sulfate (FP0S15:0.05Cr), a very intense absorption band is obtained at ~251 nm due to the charge transfer state of Cr6+. Upon excitation at 405 nm, a broad emission band at around 734 nm is observed in the FP10S5:0.05Cr glass, corresponding to the 4T2 → 4A2 transition of Cr3+. With increasing S/P ratio, besides this broad emission band, another overlapping sharper emission band at 694 nm is observed in FP0S15:0.05Cr, originating from the spin-forbidden transition of Cr3+:2E → 4A2. This phenomenon implies that higher sulfate content leads to partial crystallization of the FP0S15:0.05Cr sample. By monitoring the emission at 734 nm in the FP10S5:0.05Cr and FP5S10:0.05Cr glasses, a continuous envelope with individual bands at 287, 425, and 600 nm is observed, originating from the 4A2 → 4T1 (4P), 4A2 → 4T1 (4F), and 4A2 → 4T2 (4F) transitions of Cr3+. For the FP0S15:0.05Cr glass, a similar excitation spectrum is seen, but with a slight difference of intensity and band positions. The lifetime of the 734-nm emission was 34.5 ± 0.08 μs. For the FP5S10:0.05Cr and FP0S15:0.05Cr samples, the lifetimes of the emission band at 694 nm reached about 74.5 ± 0.34 and 1,070 ± 2.57 μs, respectively. The much longer lifetime of 1,070 ± 2.57 μs in FP0S15:Cr glass is taken as a further result of the presence of a crystalline phase.
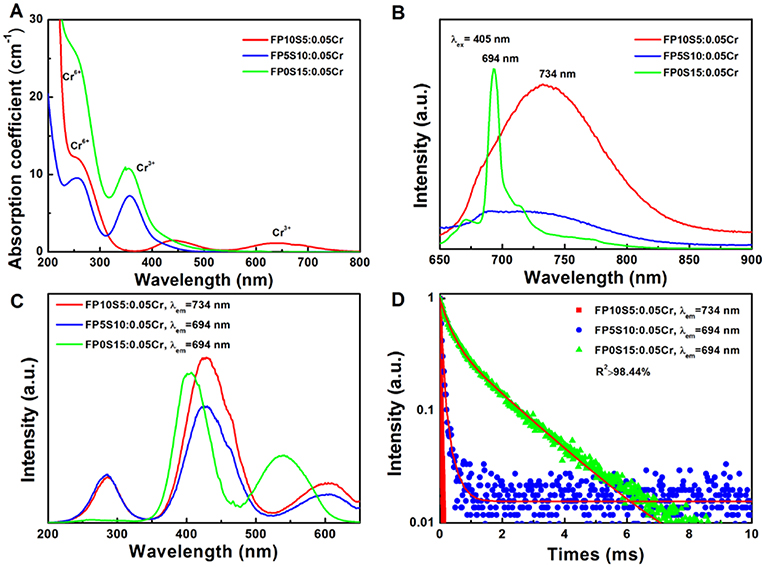
Figure 3. (A) Absorption, (B) emission spectra, (C) excitation, and (D) luminescence decay curves of chromium-doped FPS glasses with different S/P ratio.
For the Mn2+-doped glasses, the absorption, emission, excitation, and luminescence decay curves are provided in Figure 4. The key spectroscopic parameters are also summarized in Table 4. The broadband absorption peak at ~488 nm and its two shoulder peaks at 405 and 607 nm are assigned to the electronic transitions of 5Eg → 5T2g of Mn3+ (3d4 configuration, with the characteristic purple coloration), and 6A1(6S) → 4A1(4G), 4Eg(4G), and 6A1(6S) → 4T2(4G) of Mn2+ (3d5 configuration), respectively (Reddy et al., 2016). With increasing S/P ratio, the ultraviolet cutoff sideband of the Mn-doped glass shows a distinct blue shift. Upon excitation at 405 nm, a broad emission band occurs at around 590 nm (FP10S5:2Mn), corresponding to the 6T1(4G) → 6A1(6S) transition of Mn2+. The emission wavelength shows a slightly blue shift (from 590 to 576 nm), and the emission intensity becomes weaker with increasing sulfate content. This emission wavelength is significantly shorter than the one that was previously observed in fluoride-free sulfophosphate glasses (Da et al., 2010a; Möncke et al., 2014). Without going into detail at this point, we further note that the emission band is asymmetric with an overlapping feature at around 648 nm, similar to observations on Mn-doped borophosphate glasses (Ehrt, 2009). The strong compositional dependence of the emission properties of Mn2+ results from the underlying d–d transitions and their interactions with the ligand field. In the present case, Gaussian deconvolution of the emission spectra reveals the presence of two distinct components at around 580 and 630 nm. The former band is found to blue-shift (from 590 to 576 nm) with increasing S/P ratio. At the same time, its width decreases from 95 to 87 nm full width at half maximum (FWHM). The notable variation in Mn2+-related photoluminescence from glasses is typically related to variations in Mn2+ coordination between tetrahedral (green emission, high optical basicity) and octahedral (orange-red, low optical basicity) (Ehrt, 2009). We find similar features on the excitation spectra that correspond to the emissions at 590, 584, and 576 nm (see Figure 4C): all excitation spectra are composed of peaks at ~250, 350, 402, and 480 nm, corresponding to the transitions from 6A1(6S) to 4T1(4P), 4T2(4D), [4A1(4G),4E(4G)], and 4T1(4G), respectively. The dominant excitation peak is the one at ~402 nm, which is in agreement with the absorption spectra shown in Figure 4A. There is a slight blue shift of this band from 402 to 398 nm, and a red shift of the UV band from 250 to 278 nm with increasing sulfate content. The decay curves of the Mn2+-related emission exhibit a non-exponential character with average lifetimes of ~19 ± 0.02, 21 ± 0.05, and 28 ± 0.06 ms, respectively, for increasing sulfate content. The lifetime of the Mn2+-related emission slightly differs from that of other glasses, for example, Mn2+-doped phosphate (14–21 ms) (Möncke et al., 2011; Zhang et al., 2013) or sulfophosphate glasses (14.0–16.8 ms) (Da et al., 2010a), but it is significantly longer than that of borate (7 ms) (Ehrt, 2013) or aluminosilicate (~5–6 ms) (Lakshminarayana and Wondraczek, 2011) glasses.
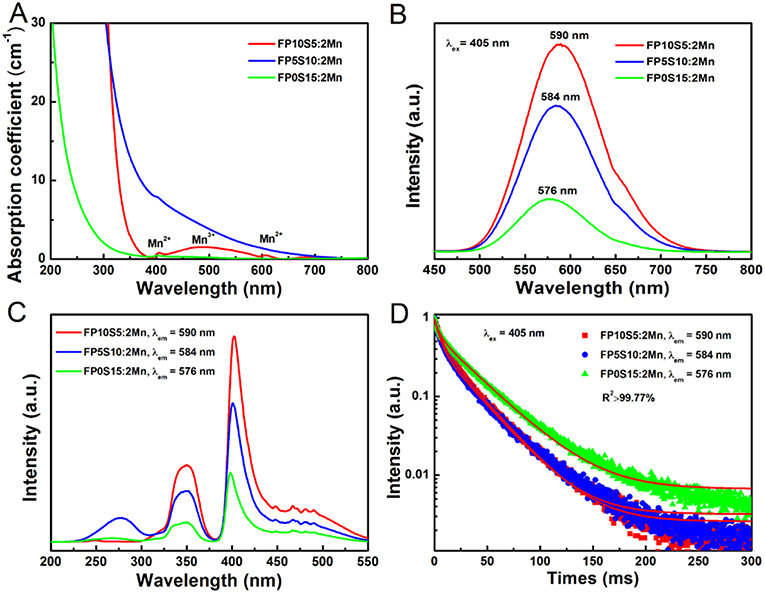
Figure 4. (A) Absorption (B), emission and (C) excitation spectra, and (D) luminescence decay curves of manganese-doped FPS glasses with different S/P ratio.
FPS/Silica Hybrid Fibers
Side views and end-face photographs of typical FPS/silica hybrid fibers with different core diameters are provided in Figure 5 (shown for undoped FPS–SIF). Figure 5A shows the boundary region between the FPS-filled and the unfilled section of a capillary with an inner diameter of 8 μm. A zoom at the filled core regions (Figures 5B,E) reveals that the FPS glass is in physical contact with the silica capillary alongside the full length of the filled sections in both fibers and that there is no delamination, porosity, or particle precipitation at least for this present observation.
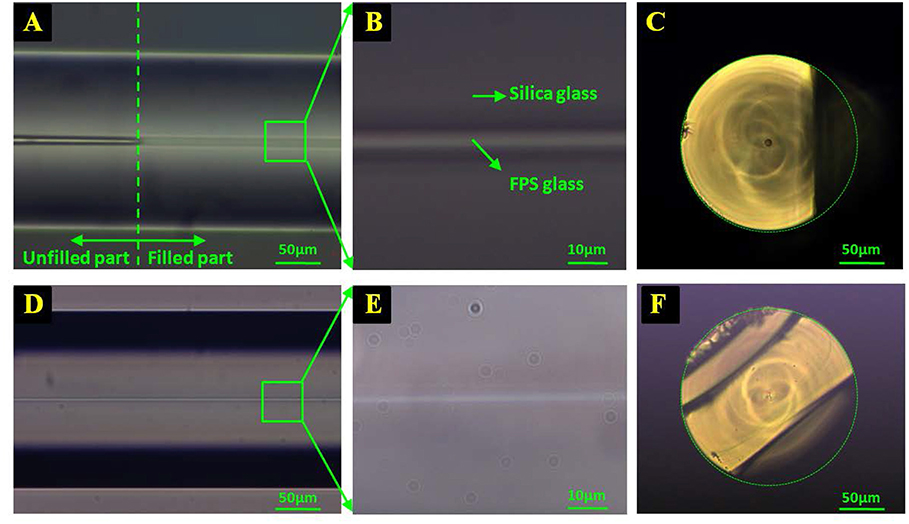
Figure 5. Microscope side views (A,B,D,E) and end-face image (C,F) of the FPS–SIF hybrid fiber [(A–C): fiber “2” and (D–F): fiber “1”)].
Raman Spectroscopy
Raman spectra of the filled section and of the cladding of FPS–SIF (fiber “2”) are given in Figure 6. The spectra were collected on four different locations as marked in the inset of Figure 6, i.e., on the surface of the bulk glass (1), on the fiber core (2), and on the fiber cladding (3) as seen on the fiber end face, and on the side view of the FPS section in the hybrid fiber (4). Spectra “1” and “2” basically correspond to FPS glass, and “3” is of silica alone, whereas “4” contains convoluted information from silica and FPS. The Raman spectra of the bulk FPS glass and of the fiber core are in very good agreement with previous structural analyses of similar FPS materials (Le et al., 2017), allowing for a clear assignment of the characteristic vibrations of v1(SO4)2− and vs(Q0) (1,000 cm−1), v2(SO4)2− (470 cm−1), and v4(SO4)2− (624 cm−1). The silica cladding “3” shows its typical Si–O–Si stretching (445 cm−1) and defect modes (491 and 605 cm−1), together with the Si–O–Si bending mode (800 cm−1) (Henderson et al., 2009). Together, all of these observations are taken as direct evidence for the efficiency of melt infiltration without notable chemical interaction between the FPS melt and the capillary walls. Noteworthy at this point, we did not observe any hints for silica dissolution such as Si–O–P stretching vibrations (1,145 cm−1) (Shibata et al., 1981) or traces of crystal precipitation.
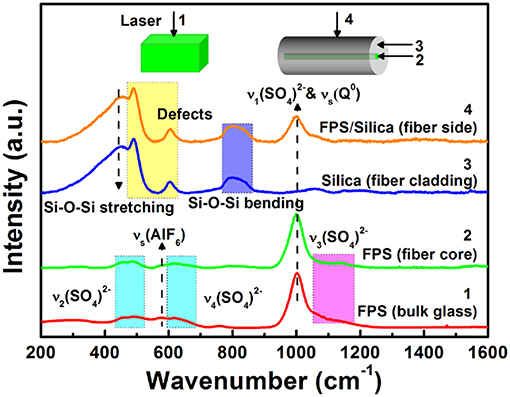
Figure 6. Raman spectra collected on different locations of the FPS–SIF (fiber “2”) and FPS bulk glass as marked in the inset: positions “2” and “3” are fiber core and fiber cladding seen from the end-face, respectively, “4” is the FPS-filled section seen from the side, and “1” is for bulk FPS.
Attenuation Measurement
Optical attenuation analysis provides qualitative information on the potential use cases of HOF devices as fiber optical waveguides. As a first step and without targeting minimal loss figures, we intend to prove optical guidance. Secondly, also in the absence of direct transmission, mode interaction in multi-core fiber can be of interest (Schmidt et al., 2009). For this reason, we considered the double-core fiber in which guidance occurs by total reflection in the GeO2-SiO2-doped region, and the (active) FPS region is exploited through mode coupling. As a directional mode coupler, the electromagnetic energy of the dual-core FPS–MGIF waveguide system is exchanged periodically along the longitudinal direction, which makes it an efficient narrowband optical fiber-integrated notch filter. The initial length of the FPS–MGIF (fiber “5”), which was used for attenuation analysis, was 68 mm. Five cut-back measurements were carried out. Figure 7A shows an example transmission spectrum with a FPS–MGIF length of 25 mm. The spectrum was normalized to a reference transmission spectrum of an unfilled MGIF. In this case, the polarization direction of incident light is parallel to the connection line of the two cores. The spectra show a series of peaks that result from modes coupling between the fundamental mode of the GeO2-SiO2 core and higher-order modes of the FPS core. The normalized attenuation is 15 dB with an FWHM bandwidth of 8 nm at the deepest dip located at 518 nm.
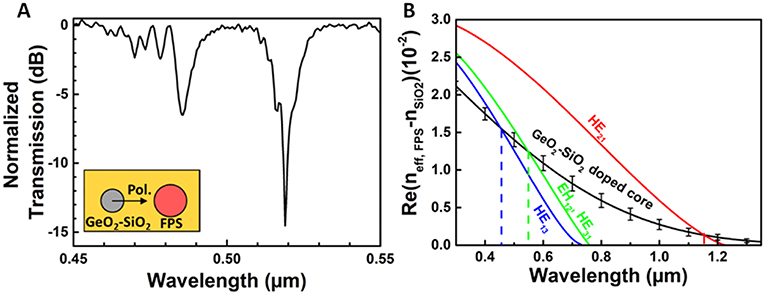
Figure 7. (A) Normalized transmission spectrum of an FPS–MGIF (fiber “5”) with a length of 25 mm. (B) Simulation of mode coupling between the fundamental mode of the GeO2-SiO2 core and the higher-order modes of the FPS-filled core. Real parts are shown of the relative effective mode indices for the different modes involved (black line: fundamental mode of the GeO2-SiO2-doped core; red, green, and blue lines: higher-order modes of the FPS core).
As described above, the MGIF consists of the GeO2-SiO2 core and the FPS core, which offers a large core-cladding index difference. By analyzing the dispersion characteristics of double-core MGIF, we can understand the operation of such hybrid fiber as a mode coupler. Figure 7B shows a simulation result of mode coupling in the FPS–MGIF. Two sharp transmission dips are observed in both polarization states with dip center wavelengths at 486 and 519 nm. These tips are associated with a matching of higher-order FPS core modes with the fundamental mode of the GeO2-SiO2 core. At these positions, the effective indices of the FPS modes cross the dispersion of the GeO2-SiO2 core mode and then the minimum transmission is observed. The maximum resonance extinction is about 15 dB with a full width half maximum bandwidth of only 2 nm for the resonance at 519 nm. The dispersion of the fundamental mode HE11 of the GeO2-SiO2 core and the dispersions of the modes of the FPS were calculated by a mode solver (ATSOS). For the calculation of existing modes in the FPS core, the refractive indices of doped and undoped silica dioxide were applied. Here, a higher refractive index for the FPS glass was used instead of the measured refractive index of the bulk glass (increased by Δn = 0.007) mainly because of the following two reasons: (i) The molten FPS glass was pressed by the argon gas into the small hollow capillary, which was moved out of the furnace promptly after filling process. Therefore, the residual stress likely increased the density of the material which results in a somewhat larger refractive index (Yablon, 2004). (ii) The accuracy of the ellipsometry for the measurement of refractive index is ±0.005. Thus, it is reasonable to increase the refractive index by a same magnitude. The relative effective mode index between the effective index of the FPS modes and the refractive index of pure silica (neff, FPS – nSiO2) are plotted to rescale the y-axis for better illustration. The couplings occur at those wavelengths where the curves of the higher FPS modes (HE13, HE31, EH12) intersect with the curve of fundamental mode of the GeO2-SiO2 core. The fundamental mode (HE11) of the FPS core does not show a cross-point with the GeO2-SiO2 mode in the observed spectral range. As seen in Figure 7B, the simulation reveals the first coupling in the infrared range; the second and third couplings are in the visible wavelength range. In the VIS range, the simulated resonance wavelengths are also at 486 and 519 nm, which are consistent with the results in the transmission spectra. The simulation results have good matching with respect to the experimental result, indicating that the refractive index of the FPS strand within the capillary is a little higher than the bulk sample.
The attenuation of the FPS–MGIF (fiber 5) at a wavelength of 518 nm was determined based on the five different cut backs (Figure 8). Using linear regression, the attenuation loss was calculated at 0.28 dB/mm. The higher fiber loss originates primarily from the intentional dopants, but potentially also from impurities in the raw materials. In addition, due to the mismatch between the thermal expansion coefficient of FPS glass and silica, interface roughness may be another factor. Although the fiber loss is high, it is already lower than previous reports on other PAMF-derived HOFs. For example, in a similar metaphosphate/silica hybrid fiber, such system's potential for supercontinuum generation was demonstrated even for a fiber loss of ~0.35 dB/mm (Chemnitz et al., 2016). Further reduction of fiber loss may be envisioned for optimized processing and enhanced purity of raw materials. From the present demonstration study, we conclude at this point that the FPS–MGIF system can act as an efficient and narrow bandwidth fiber-integrated notch filter.
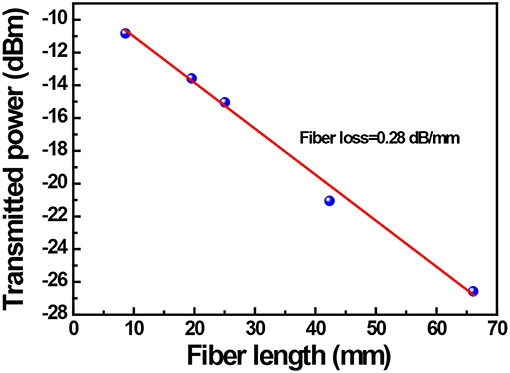
Figure 8. Attenuation loss of FPS–MGIF (fiber 5) for cut-back measurements at a wavelength of 518 nm. The linear data fit yields a fiber loss of 0.28 dB/mm.
Local Emission Spectra of the FPS/Silica Hybrid Fiber
The local emission spectra of the FPS/silica SIF hybrid fiber doped with Cr3+ (fiber “3”) and Mn2+ (fiber “4”) as well as a comparison with FPS bulk glass measured using a Raman microscope with a 514-nm laser excitation are given in Figure 9. We find intense luminescence at 767 and 630 nm in the Mn2+- and Cr3+-doped fiber core (position “1,” see the inset of Figure 9), respectively. The emission characteristics are similar to those of the bulk glasses when the spectra are normalized, with a slight red shift at the higher excitation wavelength. In the un-doped cladding region, no emission is observed (position “2”). Other Mn2+- and Cr3+-doped glass fibers have been investigated recently. For example, Mn2+-doped gallate glass and glass fiber were prepared by Lv et al. (2018). They observed a broad emission band at 635 nm and a more narrow emission at 506 nm in the Mn2+-doped gallate glass and glass ceramic, respectively, which resulted in red and green emission color. As another example, Fang et al. obtained an intense emission band ranging from 600 to 800 nm in a Cr3+-doped glass ceramic fiber upon excitation at 532 nm (Fang et al., 2015). The much narrower emission bands than our results are because of the strong crystal field of the glass ceramic matrix.
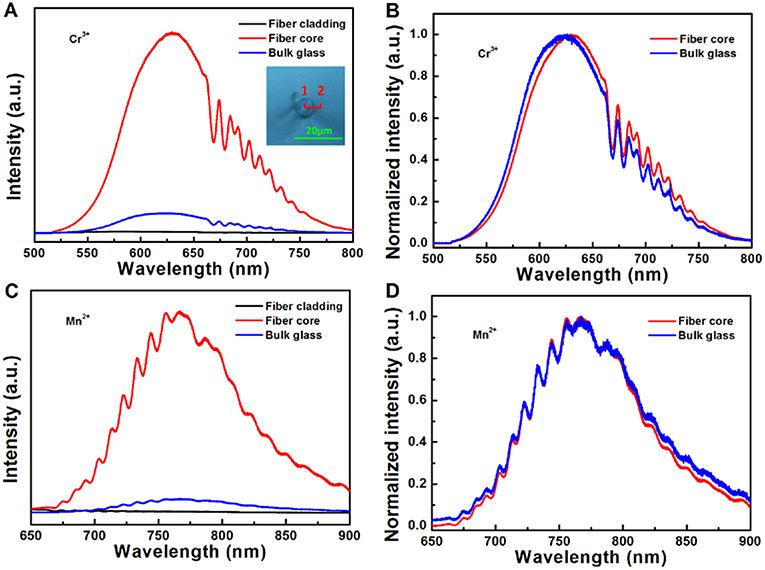
Figure 9. (A) Local emission spectra and (B) normalized emission spectra of the FPS/silica hybrid fiber doped with Cr3+ (fiber “3”) and comparison to bulk FPS glass counterpart. (C) Local emission spectra and (D) normalized emission spectra of the FPS/silica hybrid fiber doped with Mn2+ (fiber “4”) and comparison to bulk FPS glass counterpart. The inset shows the fiber core/cladding boundary region; positions “1” and “2” are the fiber core and cladding (located from the core/cladding boundary ~3 μm), respectively.
Conclusions
We presented PAMF for poly-anionic FPS glasses, fabricating FPS/silica HOF as a platform for a broad variety of optically active fiber devices. For demonstration purposes, we chose Cr3+- and Mn2+-doped FPS, on which we have shown how the spectral characteristics of the bulk material persist also in the hybrid fiber. Using a double-core fiber structure in which waveguiding is conducted in the primary GeO2-SiO2 core with mode coupling to the secondary FPS-filled core allows for exploiting the optical activity of the material even at high intrinsic loss.
Data Availability
The datasets generated for this study are available on request to the corresponding author.
Author Contributions
LW and MS conceived the present project. W-CW carried out the FPS glass and strand fabrication and was responsible for the majority of physical characterization (including UV–Vis absorption, Raman, photoluminescence, and photoluminescence excitation spectroscopy). XY and TW conducted the PAMF experiments and analyzed the obtained HOF devices. All authors participated in data evaluation, discussion, and interpretation.
Funding
This work was funded by the German Science Foundation (DFG) through grants no. WO1220/9-2, WO1220/15-1, and SCHM2655/10-1. W-CW gratefully acknowledges the Chinese Scholarship Council (CSC) for personal support.
Conflict of Interest Statement
The authors declare that the research was conducted in the absence of any commercial or financial relationships that could be construed as a potential conflict of interest.
Acknowledgments
We thank our colleagues at OSIM for help with sample preparation and data acquisition.
References
Ballato, J., and Peacock, A. C. (2018). Perspective: molten core optical fiber fabrication—a route to new materials and applications. APL Photonics 3:120903. doi: 10.1063/1.5067337
Benabid, F., Knight, J. C., Antonopoulos, G., and Russell, P. S. J. (2002). Stimulated Raman scattering in hydrogen-filled hollow-core photonic crystal fiber. Science 298, 399–402. doi: 10.1126/science.1076408
Chemnitz, M., Gebhardt, M., Gaida, C., Stutzki, F., Kobelke, J., Limpert, J., et al. (2017). Hybrid soliton dynamics in liquid-core fibres. Nat. Commun. 8, 1–11. doi: 10.1038/s41467-017-00033-5
Chemnitz, M., Wei, J. X., Jain, C., Rodrigues, B. P., Wieduwilt, T., Kobelke, J., et al. (2016). Octave-spanning supercontinuum generation in hybrid silver metaphosphate/silica step-index fibers. Opt. Lett. 41, 3519–3522. doi: 10.1364/OL.41.003519
Chen, Z. T., Song, E. H., Wu, M., Zhou, B., and Zhang, Q. Y. (2016). Exchange coupled Mn–Mn pair: an approach for super-broadband 1380 nm emission in α-MnS. Appl. Phys. Lett. 109:191907. doi: 10.1063/1.4967452
Cheng, T. L., Gao, W.Q., Kawashima, H., Deng, D., Liao, M., Matsumoto, M., et al. (2014). Widely tunable second-harmonic generation in a chalcogenide–tellurite hybrid optical fiber. Opt. Lett. 39, 2145–2147. doi: 10.1364/OL.39.002145
Da, N., Enany, A. A., Granzow, N., Schmidt, M. A., Russell, P. S., and Wondraczek, L. (2011). Interfacial reactions between tellurite melts and silica during the production of microstructured optical devices. J. Non Cryst. Solids 357, 1558–1563. doi: 10.1016/j.jnoncrysol.2010.12.032
Da, N., Peng, M., Krolikowski, S., and Wondraczek, L. (2010a). Intense red photoluminescence from Mn2+-doped (Na+; Zn2+) sulfophosphate glasses and glass ceramics as LED converters. Opt. Express 18, 2549–2557. doi: 10.1364/OE.18.002549
Da, N., Wondraczek, L., Schmidt, M. A., Granzow, N., and Russell, P. S. J. (2010b). High index-contrast all-solid photonic crystal fibers by pressure-assisted melt infiltration of silica matrices. J. Non Cryst. Solids 356, 1829–1836. doi: 10.1016/j.jnoncrysol.2010.07.002
Dianov, E. M. (2012). Bismuth-doped optical fibers: a challenging active medium for near-IR lasers and optical amplifiers. Light Sci. Appl. 1, 1–7. doi: 10.1038/lsa.2012.12
Dragic, P., Hawkins, T., Foy, P., Morris, S., and Ballato, J. (2012). Sapphire-derived all-glass optical fibres. Nat. Photonics 6, 627–633. doi: 10.1038/nphoton.2012.182
Ehrt, D. (2009). Photoluminescence in glasses and glass ceramics. IOP Conf. Ser. Mater. Sci. Eng. 2:012001. doi: 10.1088/1757-899X/2/1/012001
Ehrt, D. (2013). Zinc and manganese borate glasses—phase separation, crystallisation, photoluminescence and structure. Phys. Chem. Glasses B 54, 65–75.
Ehrt, D. (2015). Phosphate and fluoride phosphate optical glasses—properties, structure and applications. Phys. Chem. Glasses B 56, 217–234. doi: 10.13036/17533562.56.6.217
Fang, Z. J., Zheng, S. P., Peng, W. C., Zhang, H., Ma, Z. J., Zhou, S. F., et al. (2015). Fabrication and characterization of glass-ceramic fiber-containing Cr3+-doped ZnAl2O4 nanocrystals. J. Am. Ceram. Soc. 98, 2772–2775. doi: 10.1111/jace.13716
Griebenow, K., Hoppe, U., Möncke, D., Kamitsos, E. I., and Wondraczek, L. (2017). Transition-metal incorporation and Co-Sr/Mn-Sr mixed-modifier effect in metaphosphate glasses. J. Non Cryst. Solids 460, 136–145. doi: 10.1016/j.jnoncrysol.2017.01.022
Henderson, G. S., Neuville, D. R., Cochain, B., and Cormier, L. (2009). The structure of GeO2-SiO2 glasses and melts: a Raman spectroscopy study. J. Non Cryst. Solids 355, 468–474. doi: 10.1016/j.jnoncrysol.2009.01.024
Hu, L. L., He, D. B., Chen, H. Y., Wang, X., Meng, T., Wen, L., et al. (2017). Research and development of neodymium phosphate laser glass for high power laser application. Opt. Mater. 63, 213–220. doi: 10.1016/j.optmat.2016.11.052
Jackson, S. D. (2012). Towards high-power mid-infrared emission from a fibre laser. Nat. Photonics 6, 423–431. doi: 10.1038/nphoton.2012.149
Jain, C., Rodrigues, B. P., Wieduwilt, T., Kobelke, J., Wondraczek, L., and Schmidt, M. A. (2016). Silver metaphosphate glass wires inside silica fibers—a new approach for hybrid optical fibers. Opt. Express 24, 3258–3267. doi: 10.1364/OE.24.003258
Lakshminarayana, G., and Wondraczek, L. (2011). Photoluminescence and energy transfer in Tb3+/Mn2+ co-doped ZnAl2O4 glass ceramics. J. Solid State Chem. 184, 1931–1938. doi: 10.1016/j.jssc.2011.05.059
Landry, R. J., Fournier, J. T., and Young, C. G. (1967). Electron spin resonance and optical absorption studies of Cr3+ in a phosphate glass. J. Chem. Phys. 46, 1285–1290. doi: 10.1063/1.1840846
Le, Q. H., Palenta, T., Benzine, O., Griebenow, K., Limbach, R., Kamitsos, E. I., et al. (2017). Formation, structure and properties of fluoro-sulfo-phosphate poly-anionic glasses. J. Non Cryst. Solids 477, 58–72. doi: 10.1016/j.jnoncrysol.2017.09.043
Lv, S. C., Shanmugavelu, B., Wang, Y. P., Mao, Q. N., Zhao, Y. J., Yu, Y. Z., et al. (2018). Transition metal doped smart glass with pressure and temperature sensitive luminescence. Adv. Opt. Mater. 6:1800881. doi: 10.1002/adom.201800881
Möncke, D., Kamitsos, E. I., Herrmann, A., Ehrt, D., and Friedrich, M. (2011). Bonding and ion–ion interactions of Mn2+ ions in fluoride-phosphate and boro-silicate glasses probed by EPR and fluorescence spectroscopy. J. Non Cryst. Solids 357, 2542–2551. doi: 10.1016/j.jnoncrysol.2011.02.017
Möncke, D., Sirotkin, S., Stavrou, E., Kamitsos, E. I., and Wondraczek, L. (2014). Partitioning and structural role of Mn and Fe ions in ionic sulfophosphate glasses. J. Chem. Phys. 141:224509. doi: 10.1063/1.4903191
Moncorge, R. (2017). Laser materials based on transition metal ions. Opt. Mater. 63, 105–117. doi: 10.1016/j.optmat.2016.05.060
Peng, S., Tang, G. W., Huang, K. M., Qian, Q., Chen, D. D., Zhang, Q. Y., et al. (2017). Crystalline selenium core optical fibers with low optical loss. Opt. Mater. Express 7, 1804–1812. doi: 10.1364/OME.7.001804
Reddy, C. P., Naresh, V., and Reddy, K. T. R. (2016). Li2O-LiF-ZnF2-B2O3-P2O5: MnO glasses—thermal, structural, optical and luminescence characteristics. Opt. Mater. 51, 154–161. doi: 10.1016/j.optmat.2015.11.035
Rodrigues, B. P., Ebendorff-Heidepriem, H., and Wondraczek, L. (2019). Decoupling mobility and charge carrier concentration in AgR-AgPO3 glasses (R = Cl, Br, I). Solid State Ion. 334, 99–104. doi: 10.1016/j.ssi.2019.02.009
Samson, B. N., Pinckney, L. R., Wang, J., Beall, G. H., and Borrelli, N. F. (2002). Nickel-doped nanocrystalline glass-ceramic fiber. Opt. Lett. 27, 1309–1311. doi: 10.1364/OL.27.001309
Sardar, D. K., Gruber, J. B., Zandi, B., Hutchinson, J. A., and Trussell, C. W. (2003). Judd-Ofelt analysis of the Er3+(4f11) absorption intensities in phosphate glass: Er3+, Yb3+. J. Appl. Phys. 93, 2041–2046. doi: 10.1063/1.1536738
Schmidt, M. A., Argyros, A., and Sorin, F. (2016). Hybrid optical fibers—An innovative platform for in-fiber photonic devices. Adv. Opt. Mater. 4, 13–36. doi: 10.1002/adom.201500319
Schmidt, M. A., Granzow, N., Da, N., Peng, M., Wondraczek, L., Russell, P., et al. (2009). All-solid band-gap guiding in tellurite-filled silica photonic crystal fibers. Opt. Lett. 34, 1946–1948. doi: 10.1364/OL.34.001946
Schmidt, M. A., Wondraczek, L., Lee, H. W., Granzow, N., Da, N., Russell, P., et al. (2011). Complex Faraday rotation in microstructured magnetooptical fiber waveguides. Adv. Mater. 23, 2681–2688. doi: 10.1002/adma.201100364
Shibata, N., Horigudhi, M., and Edahiro, T. (1981). Raman-spectra of binary high-silica glasses and fibers containing GeO2, P2O5 and B2O3. J. Non Cryst. Solids 45, 115–126. doi: 10.1016/0022-3093(81)90096-X
Song, E. H., Chen, Z. T., Wu, M., Ding, S., Ye, S., Zhou, S. F., et al. (2016). Room-temperature wavelength-tunable single-band upconversion luminescence from Yb3+/Mn2+ codoped fluoride perovskites ABF3. Adv. Opt. Mater. 4, 798–806. doi: 10.1002/adom.201500732
Sorin, F., Abouraddy, A. F., Orf, N., Shapira, O., Viens, J., Arnold, J., et al. (2007). Multimaterial photodetecting fibers: a geometric and structural study. Adv. Mater. 19, 3872–3877. doi: 10.1002/adma.200700177
Suprasil®. Heraeus. Available online at: https://www.heraeus.com
Thieme, A., Möncke, D., Limbach, R., Fuhrmann, S., Kamitsos, E. I., and Wondraczek, L. (2015). Structure and properties of alkali and silver sulfophosphate glasses. J. Non-Cryst. Solids 410, 142–150.
Topfer, T., Hein, J., Philipps, J., Ehrt, D., and Sauerbrey, R. (2000). Tailoring the nonlinear refractive index of fluoride-phosphate glasses for laser applications. Appl. Phys. B 71, 203–206. doi: 10.1007/s003400000240
Tuniz, A., Chemnitz, M., Dellith, J., Weidlich, S., and Schmidt, M. A. (2017). Hybrid-mode-assisted long-distance excitation of short-range surface plasmons in a nanotip-enhanced step-index fiber. Nano Lett. 17, 631–637. doi: 10.1021/acs.nanolett.6b03373
Wang, S. Y., Jain, C., Wondraczek, L., Wondraczek, K., Kobelke, J., Troles, J., et al. (2015). Non-Newtonian flow of an ultralow-melting chalcogenide liquid in strongly confined geometry. Appl. Phys. Lett. 106;201908. doi: 10.1063/1.4921708
Wang, W. C., Le, Q. H., Zhang, Q. Y., and Wondraczek, L. (2017). Fluoride-sulfophosphate glasses as hosts for broadband optical amplification through transition metal activators. J. Mater. Chem. C 5, 7969–7976. doi: 10.1039/C7TC01853C
Xu, S. H., Yang, Z. M., Liu, T., Zhang, W. N., Feng, Z. M., Zhang, Q. Y., et al. (2010). An efficient compact 300 mW narrow-linewidth single frequency fiber laser at 1.5μm. Opt. Express 18, 1249–1254. doi: 10.1364/OE.18.001249
Yablon, A. D. (2004). Optical and mechanical effects of frozen-in stresses and strains in optical fibers. IEEE J. Sel. Top. Quantum Electron. 10, 300–311. doi: 10.1109/JSTQE.2004.826570
Yeh, S. M., Huang, S. L., Chiu, Y. J., Taga, H., Huang, P. L., Huang, Y. C., et al. (2012). Broadband chromium-doped fiber amplifiers for next-generation optical communication systems. J. Lightwave Technol. 30, 921–927. doi: 10.1109/JLT.2012.2182758
Keywords: fluoride-sulfophosphate glass, hybrid fiber, luminescence, pressure-assisted melt filling, optical activity
Citation: Wang W-C, Yang X, Wieduwilt T, Schmidt MA, Zhang Q-Y and Wondraczek L (2019) Fluoride-Sulfophosphate/Silica Hybrid Fiber as a Platform for Optically Active Materials. Front. Mater. 6:148. doi: 10.3389/fmats.2019.00148
Received: 11 March 2019; Accepted: 12 June 2019;
Published: 12 July 2019.
Edited by:
Jianrong Qiu, Zhejiang University, ChinaReviewed by:
John Ballato, Clemson University, United StatesLaeticia Petit, Tampere University of Technology, Finland
Copyright © 2019 Wang, Yang, Wieduwilt, Schmidt, Zhang and Wondraczek. This is an open-access article distributed under the terms of the Creative Commons Attribution License (CC BY). The use, distribution or reproduction in other forums is permitted, provided the original author(s) and the copyright owner(s) are credited and that the original publication in this journal is cited, in accordance with accepted academic practice. No use, distribution or reproduction is permitted which does not comply with these terms.
*Correspondence: Lothar Wondraczek, bG90aGFyLndvbmRyYWN6ZWtAdW5pLWplbmEuZGU=
†These authors have contributed equally to this work