- CNT Application Research Center, National Institute of Advanced Industrial Science and Technology (AIST), Tsukuba, Japan
Carbon nanotubes (CNTs) are considered to be among the most promising materials of the twenty first century due to their electronic, optical, mechanical, and thermodynamic properties. As the projected use of CNTs has increased in a variety of fields, including nanoelectronics, energy, materials science, and biology, their long-term toxicity has attracted increasing concern. This issue is especially important because CNTs are extremely physically and chemically stable, and therefore may not be biodegradable. However, novel approaches for degrading CNTs have been developed. These methods, which use peroxidases, neutrophils, and macrophages in vitro and in vivo, have yielded some encouraging results. In this mini review, we summarize current research on the biodegradation of CNTs, in particular by macrophages. We also discuss the factors that influence the biodegradation of CNTs according to their individual characteristics, with the aim of facilitating their applications.
Introduction
Carbon nanotubes (CNTs) (Iijima, 1991) have interesting electronic, optical, mechanical, and thermodynamic properties (Popov, 2004), and are therefore considered to be among the most promising materials of the twenty first century. CNTs have been applied in various fields of electronics (Baughman et al., 2002; Sgobba and Guldi, 2009), composite materials (De Volder et al., 2013), and energy (Tan et al., 2012; Antiohos et al., 2013), as well as in biological fields such as therapeutics and imaging applications (Liu et al., 2009; Martincic and Tobias, 2015; Wu et al., 2019), biosensors (Feigel et al., 2011), diagnosis (Galassi et al., 2018), nano-neuroengineering (Malarkey and Parpura, 2007), and drug delivery (Wong et al., 2013; Karimi et al., 2015). However, as the number of nanocarbon products entering the market has expanded, public concern regarding possible environmental and toxicological impacts has grown. For example, some types of CNTs can increase the risk of cancer (Guo et al., 2012; Luanpitpong et al., 2016). In addition, when CNTs enter living bodies, they become trapped by the reticuloendothelial system (RES) (liver, spleen, lung, etc.) and are difficult to excrete (Jacobsen et al., 2017). Although many studies have shown that CNTs are non-toxic in vivo in mice and rats (Schipper et al., 2008; Yang et al., 2008; Sato et al., 2013), the long-term toxicity of CNTs is still an important issue that must be resolved before CNTs can be practically applied in a widespread manner.
The greatest concern about the risk of CNTs is related to their unique physicochemical nature: specifically, these materials are extremely stable and difficult to degrade. Historically, it was widely accepted that CNTs could only be chemically degraded by strong oxidants or heat treatment in oxygen (combustion) (Ros et al., 2002; Rosca et al., 2005; Zhang et al., 2007). In 2008, however, a pioneering study showed for the first time that CNTs could be biodegraded through enzymatic oxidation by horseradish peroxidase (HRP) (Allen et al., 2008). Subsequently, many studies on the biodegradation of CNTs have been performed, many of which examined enzymatic oxidation and neutrophils or macrophages in vitro (Kagan et al., 2010; Kotchey et al., 2012, 2013; Hou et al., 2016; Yang et al., 2019) and in vivo (Nunes et al., 2012; Shvedova et al., 2012a; Kagan et al., 2014). These encouraging results are conducive to extending the applications of CNTs. In vivo biodistribution studies have shown that CNTs in living bodies are mainly distributed in macrophages in liver, lung, and spleen (Jacobsen et al., 2017). Therefore, elucidation of macrophage-mediated CNT biodegradation in vitro and in vivo is essential for overcoming biosafety concerns pertaining to these materials. In this mini review, we will summarize current in vitro and in vivo research in this field, focusing on biodegradation of CNTs by macrophages, and also discuss factors that affect the biodegradations of CNTs.
Biodegradation of CNTs in vitro
Biodegradation of CNTs by Enzymes
Biodegradation of CNTs by enzymes was first reported by Allen et al. (2008). They demonstrated that oxidized single-wall CNTs (SWCNTs) could be degraded by enzymatic oxidation with HRP, a plant enzyme. Nearly all CNTs were degraded within 10 days when a trace amount of hydrogen (H2O2) was added continuously every day (Allen et al., 2008). Degradation of SWCNTs results in shortening of nanotube length, leading to production of oxidized polyaromatic hydrocarbons and ultimately CO2 (Allen et al., 2009). Later, multi-wall CNTs (MWCNTs) were studied using the same method (Russier et al., 2011; Zhao et al., 2011): the results revealed that HRP could degrade those CNTs within 90 days. CNT biodegradation studies were later extended to animal-derived peroxidases, such as myeloperoxidase (MPO) expressed in neutrophils (Kagan et al., 2010; Bhattacharya et al., 2014), eosinophil peroxidase (EPO) (Andón et al., 2013), and lactoperoxidase (LPO) (Bhattacharya et al., 2015) expressed by goblet cells. Other enzymes such as lignin peroxidase (Chandrasekaran et al., 2014), xanthine oxidase (Sureshbabu et al., 2015), and manganese peroxidase (Zhang, C. et al., 2014) are also able to break down CNTs. Together, these results show that diverse peroxidases can efficiently catalyze CNT degradation.
Biodegradation of CNTs by Macrophages
Because CNTs in living bodies are trapped by the RES system and ultimately distributed in macrophages (Jacobsen et al., 2017), biodegradation studies have focused on the breakdown of CNTs in these immune cells. Kagan et al. (2010) were the first to demonstrate that monocyte-derived macrophages freshly isolated from healthy adults can degrade oxidized SWCNTs. In a follow-up study, the same group reported that oxidative degradation of oxidized SWCNTs in phorbol 12-myristate 13-acetate (PMA)-activated THP-1 macrophages takes place mainly via a superoxide-to-peroxynitrite oxidative pathway (Kagan et al., 2014). Macrophage degradation of carbon nanohorns, which are thought to be a type of CNTs (Iijima et al., 1999), was quantitatively studied using an optical absorption method (Zhang et al., 2015). The results revealed that about 30% of oxidized carbon nanohorns were degraded by both RAW 264.7 and THP-1 cells within 9 days. Using a similar method, Yang et al. showed that SWCNTs dispersed in a solution of bovine serum albumin (BSA) could be degraded by RAW 264.7 cells and primary rat Kupffer cells (Yang et al., 2019). Degradation of oxidized CNTs by macrophages was also confirmed by observation of the structural changes of CNTs (Bussy et al., 2016; Hou et al., 2016). Studies of CNT degradation in macrophages performed to date are summarized in Table 1.
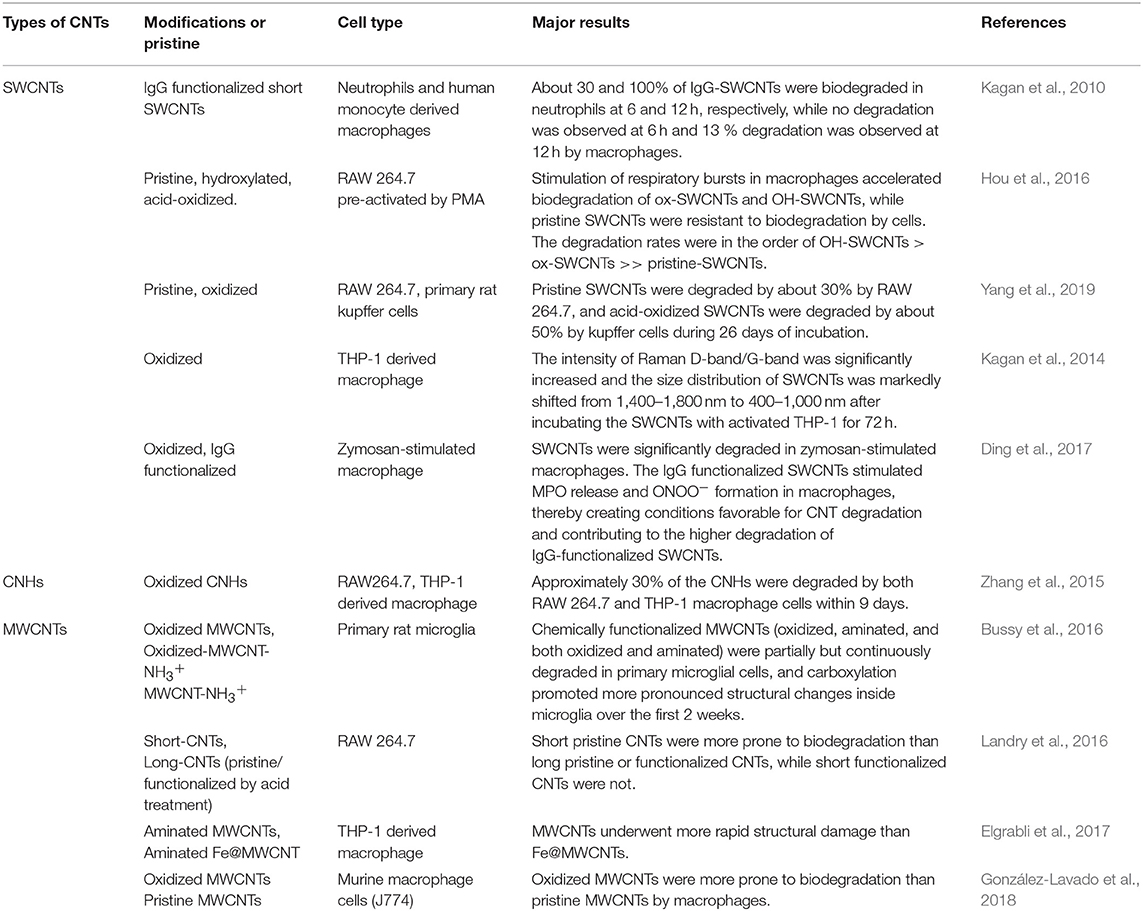
Table 1. Summary of the outcomes of macrophage-based CNT degradation methods reported in the literature.
Biodegradation Mechanism of CNTs in Macrophages
When bacteria or particles are engulfed by macrophages, oxygen consumption increases, and nicotinamide adenine dinucleotide phosphate (NADPH) oxidase assembled at the phagolysosomal membrane is activated. NADPH oxidase transfers electrons to oxygen to form reactive oxygen species (ROS) such as hydrogen peroxide (H2O2), superoxide anion (), and hydroxyl radical (OH·). ROS degrade internalized particles or bacteria, a phenomenon termed the respiratory burst or oxidative burst (Babior et al., 2002). CNTs are thought to be degraded in macrophages via this oxidative burst (Elgrabli et al., 2015; Hou et al., 2016). Using liquid-cell transmission electron microscopy, Elgrabli et al. (2015) performed in situ monitoring of ROS-mediated MWCNT degradation. They found that two degradation mechanisms are induced by hydroxyl radicals: a non–site-specific thinning process of the walls, and a site-specific transversal drilling process on pre-existing defects in the nanotubes. On the other hand, when macrophages were pre-treated with MPO or ROS inhibitors, biodegradation of CNTs in macrophages was dramatically reduced (Hou et al., 2016; Ding et al., 2017). When NADPH oxidase–deficient mice were subjected to in vivo tests, CNT degradation decreased markedly (Kagan et al., 2014). All results reported to date indicate that NADPH oxidase–dependent ROS generation plays a crucial role in CNT biodegradation by macrophages.
The biological pathway of CNT degradation in macrophages is summarized below and in Figure 1. When CNTs are engulfed by macrophages, NADPH oxidase is activated, inducing the formation of . Superoxide is then converted by superoxide dismutase (SOD) into H2O2, or reacts with free radical nitric oxide (NO·) to generate ONOO−. Enzymes such as MPO combine H2O2 with Cl− to produce hypochlorite; alternatively, in the presence of Fe3+, H2O2 is converted to OH·. ONOO−, hypochlorite, and OH· can attack CNT defects and unsaturated carbon bonds on the sidewalls of CNTs creating holes in the graphitic structure, finally resulting in the degradation of CNTs to carbon dioxide.
Biodegradation of CNTs by Macrophages in vivo
In the Lung
Elgrabli et al. (2008) intratracheally instilled MWCNTs with a diameter of about 20–30 nm into rat lungs, and found that the nanotubes persisted for 6 months, concomitant with a significant increase in the number of alveolar macrophages in the lung. Since the MWCNTs used in this study were synthesized by chemical vapor deposition method with nickel catalysts, and Ni content in MWCNTs sample was used to estimate the quantity change of MWCNTs inside of lung. Results show that MWCNT doesn't cross the lung barrier and is eliminated from the lung. MWCNT length was significantly decreased after instillation for 15 days, suggesting that the nanotubes were undergoing cleavage. In the meantime, alcohol, carbonyl, and nitrogen groups were introduced on the MWCNTs. Although further investigations are needed, the authors clearly showed that alveolar macrophages play major roles in eliminating MWCNTs from the lung. Kagan's group instilled oxidized SWCNTs into the lungs of MPO-knockout (Shvedova et al., 2012a) or NADPH oxidase–deficient mice (Kagan et al., 2014) via pharyngeal aspiration, and found that clearance of SWCNTs was markedly less effective in both mutants than in the wild type.
In the Brain
Nunes et al. stereotactically injected amine-functionalized MWCNTs into the cortex of mouse brain (Nunes et al., 2012) and found that the nanotubes were predominantly internalized within microglia, the resident macrophages of the brain, at early time points. TEM analysis of nanotubes in the tissue sections revealed severe structural deformations, reduced length, and loss of cylindrical structure, suggesting that nanotube degradation can start within 2 days post-injection. Raman spectral analysis confirmed that the degree of structural defects in CNTs increased with time. Although the underlying degradative processes and mechanisms were not elucidated, this study provided the first direct evidence for degradation of chemically functionalized MWCNTs within brain cortex.
In the Liver
Zhang et al. encapsulated Gd2O3 nanoparticle labels within CNHs. By measuring the concentration of gadolinium by inductively coupled plasma atomic emission spectroscopy (ICP-AES), they quantitatively determined the biodistribution and clearance of CNHs after intravenous injection into mice (Zhang, M. et al., 2014). About 70% of CNHs accumulated in the liver, and over 4 months 40% of the injected CNHs were cleared from the body. However, only 15% were excreted in feces, most likely via the biliary pathway into the intestine. The remaining 25% were inferred to be partly or completely degraded in the liver. No studies to date have examined the degradation of SWCNTs or MWCNTs by liver.
Factors That Influence the Biodegradability of CNTs
As shown above, CNTs can be degraded by macrophages in vitro and in vivo. However, the degree of CNT degradation is not always consistent across studies, probably due to differences in the types of CNTs and experimental conditions used. To give a comprehensive picture of the biodegradability of CNTs, here we discuss several important factors that influence biodegradability.
CNT Types and Lengths
SWCNTs are more easily degraded than MWCNTs, which take a longer time to degrade in both in vitro enzymatic systems (Zhao et al., 2011) and cells (Bussy et al., 2016). Modugno et al. compared the biodegradability of double-walled CNTs (DWCNTs) and MWCNTs of various lengths, degrees of oxidation, and functionalizations. Raman and TEM analysis revealed that short MWCNTs functionalized by amidation were most efficiently broken down by HRP, whereas DWCNTs were more resistant to the degradative action of HRP, even after functionalization (Modugno et al., 2016). Because hypochlorite generated by enzymatic reactions is a key oxidizer of CNTs inside of macrophages or neutrophils (Vlasova et al., 2011, 2012), Zhang et al. used sodium hypochlorite to investigate the biodegradation features of 11 types of commercially available CNTs. They found that degradation of CNTs was generally diameter- or thickness-dependent, with the degradation rates following the order SWCNTs ≥ CNHs > thin MWCNTs > thick MWCNTs (Zhang et al., 2019).
CNT length also seems to influence degradation rate. Landry et al. (2016) compared four MWCNTs differing in length and/or surface chemistry, and found that short pristine MWCNTs were more prone to biodegradation than longer MWCNTs. They postulated that this is because short CNTs are more accessible to iron and encapsulated higher-Fe nanoparticles than long CNTs. Iron could react with nanotubes via the Fenton reaction, leading to generation of ROS, which have been proposed to be important mediators of CNT degradation.
Surface Functionalization
Oxidation of CNTs with mixed acids is a common method used to increase CNT dispersibility or carboxylate CNTs (Rosca et al., 2005; Datsyuk et al., 2008). Oxidized or carboxylated CNTs are more easily degraded by both enzymes and macrophages than pristine ones (Table 1). This may be due to structural damage or introduction of active groups (e.g., -OH and -COOH) into the rigid structure of CNTs during the oxidation process (Datsyuk et al., 2008), aiding oxidative metabolism in the cells. Degradation rates follow the order hydroxylated SWCNTs > acid-oxidized SWCNTs >> pristine SWCNTs (Hou et al., 2016). The higher the degree of incorporated defects, the higher the rate of degradation (Zhao et al., 2011). Furthermore, peroxidase-catalyzed degradation of SWCNTs proceeds efficiently with oxidized SWCNTs, in which the negatively charged carboxylated nanotubes can orient themselves toward the positively charged arginine residues in the heme site of the enzyme (Allen et al., 2009; Kagan et al., 2010). Based on observations of biodegradation in primary rat microglia cells, Bussy et al. (2016) reported that oxidized MWCNTs promote more pronounced structural changes over the first 2 weeks.
In addition to oxidation of CNTs, covalent functionalization of CNTs with designed substrates is another attractive approach. Sureshbabu et al. (2015) linked azido coumarins and catechol derivatives to MWCNTs because these moieties are good at reducing substrates and catalytic enhancers of HRP, leading to faster degradation of CNTs relative to oxidation. They also demonstrated that purine-modified MWCNTs can be effectively degraded by xanthine oxidase. A long-term comparative study of degradation of three chemically functionalized MWCNTs (carboxylated, aminated, and both carboxylated and aminated) in primary microglial cells (Bussy et al., 2016) revealed that carboxylation enhanced degradation during the first 2 weeks relative to amination, but at later times, no differences in the rate or kinetics of degradation were observed between carboxylation and amination. These data illustrate the crucial importance of the types of surface functionalities on CNTs as determinants of enzymatic biodegradability, and point out strategies for the artificial design of biodegradable CNT materials.
In addition, functionalized CNTs have been considered for application in nanomedicine (Galassi et al., 2018; Mihajlovic et al., 2019; Wu et al., 2019). For realization of such applications with less concerns of safety, designing biodegradable CNTs by functionalization have also been studied in vivo recently (González-Lavado et al., 2018).
Impurities in CNTs
Metal catalyst impurities in CNTs can also influence their biodegradation. Iron is one of the main catalyst residues during the preparation of CNTs (Prasek et al., 2011). Iron can also take part in the Fenton reaction, and Fe-CNT complexes are widely used as synergistic catalysts for degradation of organic compounds (Liao et al., 2009; Cleveland et al., 2014). Bussy et al. (2013) found that iron catalyst nanoparticles rapidly detached from pristine SWCNT backbones in macrophages and attached to SWCNTs in the cytoplasm and nucleus. Moreover, lysosomal acidification prevents iron nanoparticle detachment from CNT bundles and protects cells from downstream toxicity. However, degradation in macrophages is more rapid for MWCNTs without iron residues than for the same MWCNTs filled with 6% (w/w) of iron oxide nanoparticles (Elgrabli et al., 2015, 2017).
Other Factors
In addition to the aforementioned factors, many other parameters could influence the biodegradability of CNTs in living systems, such as dispersion states of the CNTs (Zhang et al., 2019), cell types (Kagan et al., 2010; Yang et al., 2019), uptake of CNT and immune recognition (Turabekova et al., 2014; Mukherjee et al., 2018; Zhang et al., 2018). In addition, the biodegradability of CNTs is likely to be affected by a combination of multiple factors rather than by a single factor. For example, surface functionalization may influence biodegradation of CNTs not only due to the resultant structure change, but also due to a change in dispersibility. Furthermore, the non-covalent coating of dispersant such as lipids or proteins on the CNTs surface may interfere with the degradation (Bhattacharya et al., 2014; Lu et al., 2014), and the interaction between CNTs and the physiological environment, such as the formation of “protein corona” on the surface of CNTs (Mu et al., 2008; Zhao et al., 2015), makes evaluation more complicated. Thus, biodegradation is the result of a combination of multiple factors.
Lower Toxicity of CNT Residues After Biodegradation
As reported by Kagan et al. (2010), the residues of CNTs after biodegradation do not generate an inflammatory response when aspirated into the lungs of mice. In that study, SWCNTs were firstly degraded by MPO in the presence of a small amount of hydrogen peroxide, and then the residues of SWCNTs after degradation were administrated to mice by pharyngeal aspiration. The results showed that the levels of neutrophils and cytokines in bronchoalveolar lavage fluid of mice exposed to the SWCNT residues after MPO degradation were essentially indistinguishable from control values. By contrast, the original SWCNTs elicited an acute inflammatory response and induced the formation of tissue granulomas. These results indicated the low toxicity of the SWCNT residues and the byproducts produced in degradation (Kagan et al., 2010). The low toxicity of CNTs degraded by macrophages was also confirmed by Yang et al. (2019) in vitro. ROS play crucial roles in the biodegradation of CNTs in living systems. However, it is commonly believed that massive ROS generation induces oxidative stress, and that this is the main reason for CNT-induced cytotoxicity (Shvedova et al., 2012b). The level of ROS decreased as CNT degradation occurred, returning to control levels by day 7, suggesting that cytotoxicity decreased during degradation (Yang et al., 2019).
Summary
Many studies have shown that CNTs can be biodegraded by macrophages through enzymatic oxidation. Neither CNTs undergoing biodegradation nor the byproducts of their degradation have been reported to be cytotoxic in vitro and in vivo. In addition, many factors such as CNT type, length, surface functionalization, and impurities can influence the biodegradation of CNTs. These findings will help us to use various types of CNTs according to their characteristics. Specially, the biodegradation of CNTs in living systems might be controllable through appropriate surface functionalization, which would hopefully allow us to develop biocompatible and biodegradable CNTs for biomedical applications and achieve safety by design.
However, the biodegradation of CNTs in a living system is a complicated process. In addition to the diverse characteristics of CNTs, the bio-environments of living systems, including cell types, immune recognition, and cellular uptake, also influence the biodegradation. Although CNTs could be completely degraded by enzymatic oxidation in vitro, the complete biodegradation of CNTs in the body seems difficult at present. Thus, the long-term persistence of CNTs in living systems remains an ongoing concern regarding potential long-term toxicity.
Author Contributions
MY and MZ confirm that they are the contributors to this work and have approved it for publication.
Funding
This work was supported by a KAKENHI grant (Grant-in-Aid for Scientific Research B, 17H02742) from the Japan Society for the Promotion of Science (JSPS) and by the ZEON Corporation.
Conflict of Interest Statement
The authors declare that the research was conducted in the absence of any commercial or financial relationships that could be construed as a potential conflict of interest.
References
Allen, B. L., Kichambare, P. D., Gou, P., Vlasova, I. I., Kapralov, A. A., Konduru, N., et al. (2008). Biodegradation of single-walled carbon nanotubes through enzymatic catalysis. Nano Lett. 8, 3899–3903. doi: 10.1021/nl802315h
Allen, B. L., Kotchey, G. P., Chen, Y., Yanamala, N. V., Klein-Seetharaman, J., Kagan, V. E., et al. (2009). Mechanistic investigations of horseradish peroxidase-catalyzed degradation of single-walled carbon nanotubes. J. Am. Chem. Soc. 131, 17194–17205. doi: 10.1021/ja9083623
Andón, F. T., Kapralov, A. A., Yanamala, N., Feng, W., Baygan, A., Chambers, B. J., et al. (2013). Biodegradation of single-walled carbon nanotubes by eosinophil peroxidase. Small 9, 2721–2729. doi: 10.1002/smll.201202508
Antiohos, D., Romano, M., Chen, J., and Razal, J. M. (2013). “Carbon nanotubes for energy applications,” in Syntheses and Applications of Carbon Nanotubes and Their Composites, ed S. Suzuki (London, UK: IntechOpen Limited), 495–537.
Babior, B. M., Lambeth, J. D., and Nauseef, W. (2002). The neutrophil NADPH oxidase. Arch. Biochem. Biophys. 397, 342–344. doi: 10.1006/abbi.2001.2642
Baughman, R. H., Zakhidov, A. A., and de Heer, W. A. (2002). Carbon nanotubes-the route toward applications. Science 297, 787–792. doi: 10.1126/science.1060928
Bhattacharya, K., El-Sayed, R., Andón, F. T., Mukherjee, S. P., Gregory, J., Li, H., et al. (2015). Lactoperoxidase-mediated degradation of singlewalled carbon nanotubes in the presence of pulmonary surfactant. Carbon 91, 506–517. doi: 10.1016/j.carbon.2015.05.022
Bhattacharya, K., Sacchetti, C., El-Sayed, R., Fornara, A., Kotchey, G. P., Gaugler, J. A., et al. (2014). Enzymatic ‘stripping' and degradation of PEGylated carbon nanotubes. Nanoscale 6, 14686–14690. doi: 10.1039/C4NR03604B
Bussy, C., Hadad, C., Prato, M., Bianco, A., and Kostas, K. (2016). Intracellular degradation of chemically functionalized carbon nanotubes using a long-term primary microglial culture model. Nanoscale 8, 590–601. doi: 10.1039/C5NR06625E
Bussy, C., Paineau, E., Cambedouzou, J., Brun, N., Mory, C., Fayard, B., et al. (2013). Intracellular fate of carbon nanotubes inside murine macrophages: pH-dependent detachment of iron catalyst nanoparticles. Part. Fibre Toxicol. 10:24. doi: 10.1186/1743-8977-10-24
Chandrasekaran, G., Choi, S. K., Lee, Y. C., Kim, G. J., and Shin, H. J. (2014). Oxidative biodegradation of single-walled carbon nanotubes by partially purified lignin peroxidase from Sparassis latifolia mushroom. J. Ind. Eng. Chem. 20, 3367–3374. doi: 10.1016/j.jiec.2013.12.022
Cleveland, V., Bingham, J. P., and Kan, E. (2014). Heterogeneous fenton degradation of bisphenol a by carbon nanotube-supported Fe3O4. Sep. Purif. Technol. 133, 388–395. doi: 10.1016/j.seppur.2014.06.061
Datsyuk, V., Kalyva, M., Papagelis, K., Parthenios, J., Tasis, D., Siokou, A., et al. (2008). Chemical oxidation of multiwalled carbon nanotubes. Carbon 46, 833–840. doi: 10.1016/j.carbon.2008.02.012
De Volder, M. F., Tawfick, S. H., Baughman, R. H., and Hart, A. J. (2013). Carbon nanotubes: present and future commercial applications. Science 339, 535–539. doi: 10.1126/science.1222453
Ding, Y., Tian, R., Yang, Z., Chen, J., and Lu, N. (2017). NADPH oxidase-dependent degradation of single-walled carbon nanotubes in macrophages. J. Mater. Sci. 28:7. doi: 10.1007/s10856-016-5817-z
Elgrabli, D., Dachraoui, W., de Marmier, H., Ménard-Moyon, C., Bégin, D., Bégin-Colin, S., et al. (2017). Intracellular degradation of functionalized carbon nanotube/iron oxide hybrids is modulated by iron via Nrf2 pathway. Sci. Rep. 7:40997. doi: 10.1038/srep40997
Elgrabli, D., Dachraoui, W., Ménard-Moyon, C., Liu, X. J., Bégin, D., Bégin-Colin, S., et al. (2015). Carbon nanotube degradation in macrophages-live nanoscale monitoring and understanding of biological pathway. ACS Nano 9, 10113–10124. doi: 10.1021/acsnano.5b03708
Elgrabli, D., Floriani, M., Abella-Gallart, S., Meunier, L., Gamez, C., Delalain, P., et al. (2008). Biodistribution and clearance of instilled carbon nanotubes in rat lung. Part. Fibre Toxicol. 5:20. doi: 10.1186/1743-8977-5-20
Feigel, I. M., Vedalaa, H., and Star, A. (2011). Biosensors based on one-dimensional nanostructures. J. Mater. Chem. 21, 8940–8954. doi: 10.1039/c1jm10521c
Galassi, T. V., Jena, P. V., Shah, J., Ao, G., Molitor, E., Bram, Y., et al. (2018). An optical nanoreporter of endolysosomal lipid accumulation reveals enduring effects of diet on hepatic macrophages in vivo. Sci Transl Med. 10:eaar2680. doi: 10.1126/scitranslmed.aar2680
González-Lavado, E., Iturrioz-Rodríguez, N., Padín-González, E., González, J., García-Hevia, L., Heuts, J., et al. (2018). Biodegradable multi-walled carbon nanotubes trigger anti-tumoral effects. Nanoscale 10:11013. doi: 10.1039/C8NR03036G
Guo, N. L., Wan, Y. W., Denvir, J., Porter, D. W., Pacurari, M., Wolfarth, M. G., et al. (2012). Multiwalled carbon nanotube-induced gene signatures in the mouse lung: potential predictive value for human lung cancer risk and prognosis. J. Toxicol. Environ. Health A 75, 1129–1153. doi: 10.1080/15287394.2012.699852
Hou, J., Wan, B., Yang, Y., Ren, X. M., Guo, L. H., and Liu, J. F. (2016). Biodegradation of single-walled carbon nanotubes in macrophages through respiratoroy burst modulation. Int. J. Mol. Sci. 17:409. doi: 10.3390/ijms17030409
Iijima, S. (1991). Helical microtubules of graphitic carbon. Nature 354, 56–58. doi: 10.1038/354056a0
Iijima, S., Yudasaka, M., Yamada, R., Bandow, S., Suenaga, K., Kokai, F., et al. (1999). Nano-aggregates of single-walled graphitic carbon nano-horns. Chem. Phys. Lett. 309, 165–170. doi: 10.1016/S0009-2614(99)00642-9
Jacobsen, N. R., Møller, P., Clausen, P. A., Saber, A. T., Micheletti, C., Jensen, K. A., et al. (2017). Biodistribution of carbon nanotubes in animal models. Basic Clin. Pharmacol. Toxicol. 121 (suppl. 3), 30–43. doi: 10.1111/bcpt.12705
Kagan, V. E., Kapralov, A. A., St. Croix, C. M., Watkins, S. C., Kisin, E. R., Kotchey, G. P., et al. (2014). Lung macrophages “digest” carbon nanotubes using a superoxide/peroxynitrite oxidative pathway. ACS Nano. 8, 5610–5621. doi: 10.1021/nn406484b
Kagan, V. E., Konduru, N. V., Feng, W., Allen, B. L., Conroy, J., Volkov, Y., et al. (2010). Carbon nanotubes degraded by neutrophil myeloperoxidase induce less pulmonary inflammation. Nat Nanotechnol. 2010, 354–359. doi: 10.1038/nnano.2010.44
Karimi, M., Solati, N., Ghasemi, A., Estiar, M. A., Hashemkhani, M., Kiani, P., et al. (2015). Carbon nanotubes part II: a remarkable carrier for drug and gene discovery. Expert Opin. Drug Deliv. 12, 1089–1105. doi: 10.1517/17425247.2015.1004309
Kotchey, G. P., Hasan, S. A., Kapralov, A. A., Ha, S. H., Kim, K., Shvedova, A. A., et al. (2012). A natural vanishing act: the enzyme-catalyzed degradation of carbon nanomaterials. Acc. Chem. Res. 45, 1770–1781. doi: 10.1021/ar300106h
Kotchey, G. P., Zhao, Y., Kagan, V. E., and Star, A. (2013). Peroxidase-mediated biodegradation of carbon nanotubes in vitro and in vivo. Adv. Drug Deliv. Rev. 65, 1921–1932. doi: 10.1016/j.addr.2013.07.007
Landry, M., Pinault, M., Tchankouo, S., Charon, É., Ridoux, A., Boczkowski, J., et al. (2016). Early signs of multi-walled carbon nanotbues degradation in macrophages, via an intracellular pH-dependent biological mechanism; importance of length and functionalization. Part. Fibre Toxicol. 13:61. doi: 10.1186/s12989-016-0175-z
Liao, Q., Sun, J., and Gao, L. (2009). Degradation of phenol by heterogeneous Fenton reaction using multi-walled carbon nanotube supported Fe2O3 catalysts. Colloids Surf. A 345, 95–100. doi: 10.1016/j.colsurfa.2009.04.037
Liu, Z., Tabakman, S., Welsher, K., and Dai, H. (2009). Carbon nanotubes in biology and medicine: In vitro and in vivo detection, imaging and drug delivery. Nano Res. 2, 85–120. doi: 10.1007/s12274-009-9009-8
Lu, N., Li, J., Tian, R., and Peng, Y. Y. (2014). Binding of human serum albumin to single-walled carbon nanotubes activated neutrophils to increase production of hypochlorous acid, the oxidant cable of degrading nanotubes. Chem. Res. Toxicol. 27, 1070–1077. doi: 10.1021/tx5001317
Luanpitpong, S., Wang, L., Castranova, V., Dinu, C. Z., Issaragrisil, S., Chen, Y. C., et al. (2016). Induction of cancer-associated fibroblast-like cells by carbon nanotubes dictates its tumorigenicity. Sci. Rep. 6:39558. doi: 10.1038/srep39558
Malarkey, E. B., and Parpura, V. (2007). Applications of carbon nanotubes in neurobiology. Neurodegener. Dis. 4, 292–299. doi: 10.1159/000101885
Martincic, M., and Tobias, G. (2015). Filled carbon nanotubes in biomedical imaging and drug delivery. Expert Opin. Drug Deliv. 12, 563–581. doi: 10.1517/17425247.2015.971751
Mihajlovic, M., Mihajlovic, M., Dankers, P. Y. W., Masereeuw, R., and Sijbesma, R. P. (2019). Carbon nanotube reinforced supramolecular hydrogels for bioapplications. Macromol. Biosci. 19:e1800173. doi: 10.1002/mabi.201800173
Modugno, G., Ksar, F., Battigelli, A., Russier, J., Loncharmbon, P., da Silva, E. E., et al. (2016). A comparative study on the enzymatic biodegradability of covalently functionalized double- and multi-walled carbon nanotubes. Carbon 100, 367–374. doi: 10.1016/j.carbon.2016.01.023
Mu, Q., Liu, W., Xing, Y., Zhou, H., Li, Z., Zhang, Y., et al. (2008). Protein binding by functionalized multiwalled carbon nanotubes is governed by the surface chemistry of both parties and the nanotube diameter. J. Phys. Chem. C 112, 3300–3307. doi: 10.1021/jp710541j
Mukherjee, S. P., Bondarenko, O., Kohonen, P., Andón, F. T., Brzicov,á, T., Gessner, I., et al. (2018). Macrophage sensing of single-walled carbon nanotubes via Toll-like receptors. Sci. Rep. 8:1115. doi: 10.1038/s41598-018-19521-9
Nunes, A., Bussy, C., Gherardini, L., Meneghetti, M., Herrero, M. A., Bianco, A., et al. (2012). In vivo degradation of functionalized carbon nanotubes after stereotactic administration in the brain cortex. Nanomedicine 7, 1485–1494. doi: 10.2217/nnm.12.33
Popov, V. N. (2004). Carbon nanotubes: properties and application. Mat. Sci. Eng. R. 43, 61–102. doi: 10.1016/j.mser.2003.10.001
Prasek, J., Drbohlavova, J., Chomoucka, J., Hubalek, J., Jasek, O., Adam, V., et al. (2011). Methods for carbon nanotubes synthesis-review. J. Mater. Chem. 21, 15872–15884. doi: 10.1039/c1jm12254a
Ros, T. G., van Dillen, A. J., Geus, J. W., and Koningsberger, D. C. (2002). Surface oxidation of carbon nanofibers. Chem. Eur. J. 8, 1151–1162. doi: 10.1002/1521-3765(20020301)8:5<1151::AID-CHEM1151>3.0.CO;2-#
Rosca, I. D., Watari, F., Uo, M., and Akasaka, T. (2005). Oxidation of multiwalled carbon nanotubes by nitric acid. Carbon 43, 3124–3131. doi: 10.1016/j.carbon.2005.06.019
Russier, J., Ménard-Moyon, C., Venturelli, E., Gravel, E., Marcolongo, G., Meneghetti, M., et al. (2011). Oxidative biodegradation of single- and multi-walled carbon nanotubes. Nanoscale 3, 893–896. doi: 10.1039/C0NR00779J
Sato, Y., Yokoyama, A., Nodasaka, Y., Kohgo, T., Motomiya, K., Matsumoto, H., et al. (2013). Long-term biopersistence of tangled oxidized carbon nanotubes inside and outside macrophages in rat subcutaneous tissue. Sci. Rep. 3:2516. doi: 10.1038/srep02516
Schipper, M. L., Nakayama-Ratchford, N., Davis, C. R., Kam, N. W. S., Chu, P., Liu, Z., et al. (2008). A pilot toxicology study of single-walled carbon nanotubes in a small sample of mice. Nat. Nanotechnol. 3, 218–221. doi: 10.1038/nnano.2008.68
Sgobba, V., and Guldi, D. M. (2009). Carbon nanotubes—electronic/electrochemical properties and application for nanoelectronics and photonics. Chem. Soc. Rev. 38, 165–184. doi: 10.1039/B802652C
Shvedova, A. A., Kapralov, A. A., Feng, W. H., Kisin, E. R., Murray, A. R., Mercer, R. R., et al. (2012a). Impaired clearance and enhanced pulmonary inflammatory-fibrotic response to carbon nanotubes in myeloperoxidase-deficient mice. PLoS ONE 7:e30923. doi: 10.1371/journal.pone.0030923
Shvedova, A. A., Pietroiusti, A., Fadeel, B., and Kagan, V. E. (2012b). Mechanisms of carbon nanotube-induced toxicity: focus on oxidative stress. Toxicol. Appl. Pharmacol. 261, 121–133. doi: 10.1016/j.taap.2012.03.023
Sureshbabu, A. R., Kurapati, R., Russier, J., Ménard-Moyon, C., Bartolini, I., Meneghetti, M., et al. (2015). Degradation-by-design: surface modification with functional substrates that enhance the enzymatic degradation of carbon nanotubes. Biomaterials 72, 20–28. doi: 10.1016/j.biomaterials.2015.08.046
Tan, C. W., Tan, K. H., Ong, Y. T., Mohamed, A. R., Zein, S. H. S., and Tan, S. H. (2012). Energy and environmental applications of carbon nanotubes. Environ. Chem. Lett. 10, 265–273. doi: 10.1007/s10311-012-0356-4
Turabekova, M., Rasulev, B., Theodore, M., Jackman, J., Leszczynska, D., and Leszczynski, J. (2014). Immunotoxicity of nanoparticles: a computational study suggests that CNTs and C60 fullerenes might be recognized as pathogens by Toll-like receptors. Nanoscale 6, 3488–3495. doi: 10.1039/C3NR05772K
Vlasova, I. I., Sokolov, A. V., Chekanov, A. V., Kostevich, V. A., and Vasil'ev, V. B. (2011). Myeloperoxidase induced biodegradation of single walled carbon nanotubes is mediated by hypochlorite. Bioorg. Khim. 37, 510–521 doi: 10.1134/S1068162011040157
Vlasova, I. I., Vakhrusheva, T. V., Sokolov, A. V., Kostevich, V. A., Gusev, A. A., Gusev, S. A., et al. (2012). PEGylated single-walled carbon nanotubes activate neutrophils to increase production of hypochlorous acid, the oxidant capable of degrading nanotubes. Toxicol. Appl. Pharmacol. 264, 131–142. doi: 10.1016/j.taap.2012.07.027
Wong, B. S., Yoong, S. L., Jagusiak, A., Panczyk, T., Ho, H. K., Ang, W. H., et al. (2013). Carbon nanotubes for delivery of small molecule drugs. Adv. Drug Deliv. Rev. 65, 1964–2015. doi: 10.1016/j.addr.2013.08.005
Wu, L., Tang, H., Zheng, H., Liu, X., Liu, Y., Tao, J., et al. (2019). Multiwalled carbon nanotubes prevent tumor metastasis through switching M2-polarized macrophages to M1 via TLR4 activation. J. Biomed. Nanotechnol. 15, 138–150. doi: 10.1166/jbn.2019.2661
Yang, M., Zhang, M., Yudasaka, M., Iijima, S., and Okazaki, T. (2019). Time-dependent degradation of carbon nanotubes correlates with decreased reactive oxygen species generation in macrophages. Int. J. Nanomed. 14, 2797–2807. doi: 10.2147/IJN.S199187
Yang, S. T., Wang, X., Jia, G., Gu, Y., Wang, T., Nie, H., et al. (2008). Long-term accumulation and low toxicity of single-walled carbon nanotubes in intravenously exposed mice. Toxic Lett. 181, 182–189. doi: 10.1016/j.toxlet.2008.07.020
Zhang, C., Chen, W., and Alvarez, P. J. (2014). Manganese peroxidase degrades pristine but not surface-oxidized (carboxylated) single-walled carbon nanotubes. Environ. Sci. Technol. 48, 7918–7923. doi: 10.1021/es5011175
Zhang, M., Tahara, Y., Yang, M., Zhou, X., Iijima, S., and Yudasaka, M. (2014). Quantification of whole body and excreted carbon nanohorns intravenously injected into mice. Adv. Healthc. Mater. 3, 239–244. doi: 10.1002/adhm.201300192
Zhang, M., Yang, M., Bussy, C., Iijima, S., Kostarelos, K., and Yudasaka, M. (2015). Biodegradation of carbon nanohorns in macrophage cells. Nanoscale 7, 2834–2840. doi: 10.1039/C4NR06175F
Zhang, M., Yang, M., Morimoto, T., Tajima, N., Ichiraku, K., Fujita, K., et al. (2018). Size-dependent cell uptake of carbon nanotubes by macrophages: A comparative and quantitative study. Carbon 127, 93–101. doi: 10.1016/j.carbon.2017.10.085
Zhang, M., Yang, M., Nakajima, H., Yudasaka, M., Iijima, S., and Okazaki, T. (2019). Diameter-dependent degradation of 11 types of carbon nanotubes: safety implications. ACS Appl. Nano Mater. 2, 4293–4301. doi: 10.1021/acsanm.9b00757
Zhang, M., Yudasaka, M., Ajima, K., Miyawaki, J., and Iijima, S. (2007). Light-assisted oxidation of single-wall carbon nanohorns for abundant creation of oxygenated groups that enable chemical modifications with proteins to enhance biocompatibility. ACS Nano 1, 265–272. doi: 10.1021/nn700130f
Zhao, X., Lu, D., Hao, F., and Liu, R. (2015). Exploring the diameter and surface dependent conformational changes in carbon nanotube-protein corona and the related cytotoxicity. J. Hazard. Mater. 292, 98–107. doi: 10.1016/j.jhazmat.2015.03.023
Keywords: carbon nanotubes, carbon nanomaterial, biodegradation, macrophage—cell, enzyme
Citation: Yang M and Zhang M (2019) Biodegradation of Carbon Nanotubes by Macrophages. Front. Mater. 6:225. doi: 10.3389/fmats.2019.00225
Received: 19 July 2019; Accepted: 02 September 2019;
Published: 18 September 2019.
Edited by:
Emilia Morallon, University of Alicante, SpainReviewed by:
Alberto Bianco, Centre National de la Recherche Scientifique (CNRS), FranceFernando Torres Andón, University of Santiago de Compostela, Spain
Copyright © 2019 Yang and Zhang. This is an open-access article distributed under the terms of the Creative Commons Attribution License (CC BY). The use, distribution or reproduction in other forums is permitted, provided the original author(s) and the copyright owner(s) are credited and that the original publication in this journal is cited, in accordance with accepted academic practice. No use, distribution or reproduction is permitted which does not comply with these terms.
*Correspondence: Minfang Zhang, bS16aGFuZ0BhaXN0LmdvLmpw