- Department of High-Temperature and Functional Coatings, German Aerospace Center (DLR), Institute of Materials Research, Cologne, Germany
Undoped and Co-doped TiO2 nanoparticles were synthesized by a facile co-precipitation method and calcined at 700°C. The phase identification carried out by XRD measurements and Raman spectroscopy analysis of calcined powders reveals the formation of mainly anatase phase for undoped TiO2, and 0.5 mol.% Co-doped TiO2 whereas rutile phase for 1 mol.% Co-doped TiO2. The sensors prepared with these powders deposited on interdigital (IDE) sensor platforms were tested toward NO2 and H2 sensing properties at 600°C. As the undoped and 0.5% Co-doped TiO2 reveal n-type behavior, 1% Co-doped TiO2 shows p-type semi-conductive behavior. One percentage Co-doped TiO2 exhibits good sensing performance toward NO2 while the undoped TiO2 powder yields the best sensor performance toward H2 at 600°C. This indicates that the crystal structure of TiO2 sensing material must be adjusted depending on the nature of target gas. The results indicate that the main factor influencing high temperature gas sensor performance of nanoparticulate TiO2 is either the alteration of its electronic structure or the type of polymorphs.
Highlights
- Undoped and Co-doped TiO2 were synthesized by co-precipitation route.
- Cobalt doping of TiO2 promotes the anatase-rutile transformation.
- Undoped TiO2 at 600°C, being n-type semiconductor shows the highest response to H2.
- 1% Co-doped TiO2 with p-type behavior was more sensitive toward NO2 at 600°C.
Introduction
NOx gas sensors are gaining importance in automotive exhausts and combustion systems. Better performance resistive gas sensors can endure the harsh conditions and act effectively despite the strengthened international legislations. The corresponding gas sensors require durability at high working temperatures (exceeding >> 500°C). Semiconducting metal oxides are ideally used as gas sensing materials in resistive sensors due to their numerous benefits such as reasonably high sensitivity, easy fabrication processes and low cost (Dey, 2018). Most of transition metal oxides, such as SnO2, WO3, ZnO, NiO, or CuO used for this purpose display the optimum sensitivity at temperatures below 400°C (Wang et al., 2010). On the other hand, as one of the known semiconducting oxides, TiO2 is capable of operating as a gas sensing material at temperatures as high as 600°C (Esmaeilzadeh et al., 2012). The additional benefits of TiO2 are non-toxicity, easy fabrication, and the good chemical stability (Chen and Mao, 2007). TiO2 is a high resistive n-type semiconductor with rather poor conductivity to be adopted for sensing oxidative gases such as NO2 (Huusko et al., 1993). Previous studies report that this disadvantage can be overcome by conversion of an n-type oxide to p-type semiconductor through addition of low valence dopant atoms to alter the electronic structure. Some previous studies in our team show how the Al and Cr-addition alters the electron conductivity of TiO2 from n- to p-type leading to effective gas sensing materials. As far as Cr-doped TiO2 is concerned, the optimum temperature for sensing properties has been limited with 500°C while the Al-doped TiO2 detects NO2 at 600°C but the sensitivity is low and the signal converts to p-type at 800°C (Saruhan et al., 2013; Gönüllü et al., 2015). Several other papers discuss the relation and reasons between the nature of the electronic structure and the sensing characteristics of the oxide materials (Wisitsoraat et al., 2009; Zhaohui et al., 2013; Gönüllü et al., 2015) and report that the enhancement of the sensing performance can directly be controlled by adjustment of the electronic structure to p-type at the semi-conducting oxide sensing layer.
In order to understand further if such an alteration can be achieved by Co-doping of TiO2 and how this may affect the gas sensing behavior of TiO2, we have synthetized Co-doped TiO2 nanoparticles by oxalate induced co-precipitation method and produced sensors by depositing the powders on interdigital electrodes. The resulting powders are characterized and the sensors are analyzed to identify their H2 and NO2 sensing capabilities. This paper reports, to the best of our knowledge for the first time, the effect of Co3+doping on the high temperature gas sensing behavior of TiO2.
Experimental
Undoped and Co-doped TiO2 nanoparticles were synthesized by employing the same processing route. Firstly, the starting precursor solutions were prepared. Cobalt acetate was dissolved in acetic acid as titanium iso-propoxide was diluted separately in absolute ethanol. The cobalt acetate and titanium iso-propoxide solutions were adjusted to obtain 0.5 and 1 mol.% of cobalt in TiO2 and were labeled as 0.5 Co-doped TiO2 and 1 Co-doped TiO2, respectively. These solutions were then mixed to each other and stirred for 5 min. Oxalic acid is utilized for the co-precipitation of a powder from this mixed solution. For that, oxalic acid was dissolved in absolute ethanol solution and poured progressively in to the mixed solution. The resulting mixture was stirred for 1 h to allow the complete precipitation. The so-obtained precipitate was filtered and dried in oven at 80°C yielding a white powder for the undoped and pink colored powder for the Co-doped samples. The as-prepared precursor powder was then calcined for 3 h at 700°C in a muffle furnace under static air.
The XRD diffractograms of the co-precipitated and calcined samples were collected with a D5000 Siemens Kristalloflex O-2O powder diffractometer having Bragg-Brentano geometry. The reflections from the JCPDS database were assigned to the experimental diffractograms by means of the program EVA from BRUKER AXS.
The Raman spectroscopy measurement were carried out at the Applied University of Bonn-Rhein-Sieg on Senterra Raman spectrometer from Bruker under 532 nm and 20 mW by focusing power laser excitation on samples through a 20 x objective. The morphology of the particles was determined by Scanning Electron Microscopy (SEM) analysis using a Zeiss Ultra 55 SEM.
Specific surface area of the samples was determined using Micrometrics Tristar 3,000 equipment using N2 adsorption at 77.150 K. Samples were degassed under vacuum at 120°C for 2 h prior to the surface area measurements. The specific surface area was calculated using the Brunauer-Emmet-Teller (BET) equation.
The as prepared TiO2 and Co-doped TiO2 powders were deposited as thick films using a simple drop-coating method on alumina substrates that were previously fitted with interdigitated electrodes. The sensor measurements were carried out in a specially constructed apparatus consisting of a tube furnace and a custom-built quartz glass reactor providing a thermocouple directed at the specimen. The electrical measurements were performed by using a Keithley 2635A Sourcemeter. The sensor response for n-type semiconductors is defined by (Rgas/Rair – 1) × 100 and (Rair/Rgas – 1) × 100 for oxidizing and reducing gases, respectively, while for p-type semiconductor, (Rgas/Rair – 1) × 100 and (Rair/Rgas – 1) × 100 for reducing and oxidizing gases, respectively.
Results and Discussions
The phase compositions of all synthetized powders were initially investigated by XRD as presented in Figure 1A. The undoped and the 0.5 Co-doped TiO2 samples showed pure TiO2 consisted of its two polymorphs; anatase (majority, JCPDS 21-1272) and rutile phases (minority, JCPDS 21-127). No other phase containing Co was observed. The amount of rutile phase was found to be slightly higher in 0.5 Co-doped TiO2 than the undoped one. Also, the 1 Co-doped TiO2 sample showed only single phase TiO2 but this time the rutile polymorph was the major phase as anatase phase was in trace amount.
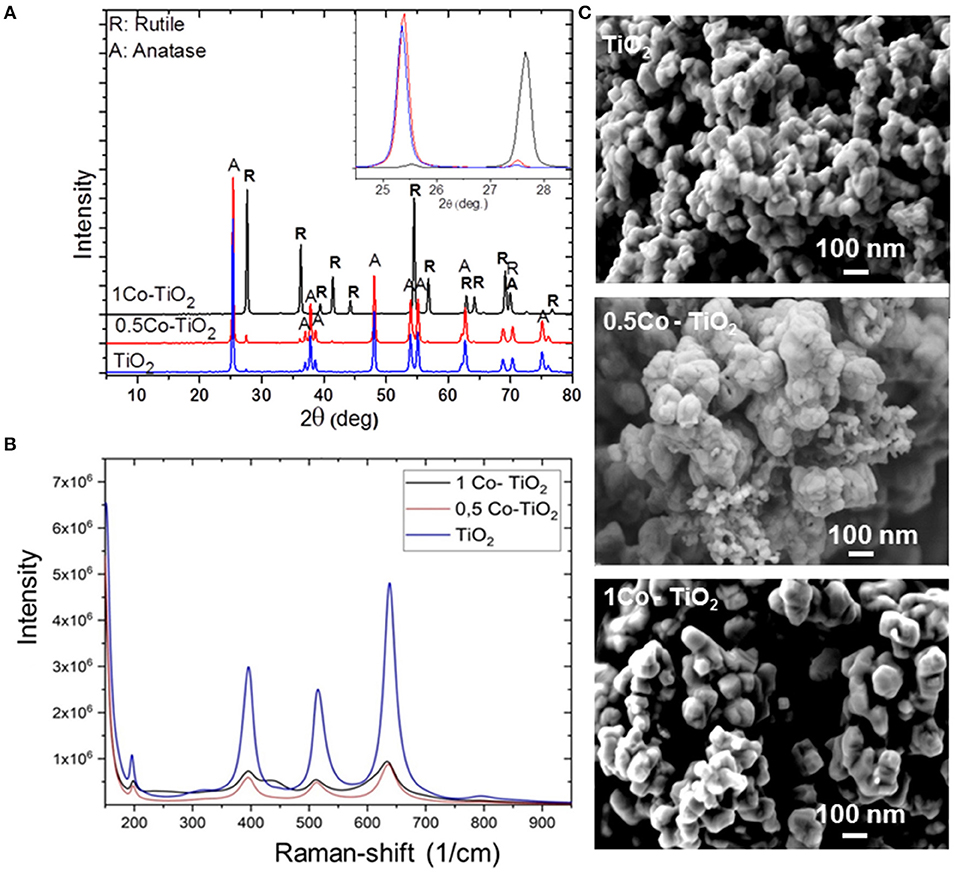
Figure 1. (A) Powder XRD patterns of undoped and Co-doped TiO2, (B) Raman spectra of undoped and Co-doped TiO2, (C) SEM images of undoped and Co-doped TiO2.
The X-ray results indicated that the cobalt dopant promotes the anatase-to-rutile phase conversion of TiO2. As presented on the inset of Figure 1A, a shift of the 2 Theta angles toward the higher values was observed indicating that the lattice parameters of TiO2 decreases on Co-addition since according to the Bragg's law equation (2dsinO = nλ), the lattice parameter is inversely proportional to 2O. Increasing 2 Theta angle does indeed mean that the lattice parameter decreases. In fact, the ionic radius of Co3+ is smaller than that of Ti4+ (Co3+ = 0.685 Å, Ti4+ = 0.745 Å). Hence, this leads to the contraction of the TiO2 unit cell on substitution of Ti4+ through Co3+. If otherwise, the substitution occurs through the incorporation of Co2+, an opposite change in the lattice parameter would be expected (i.e., 2 Theta angles shift to lower values). Since Co2+ has a larger ionic radius (Co2+ = 0.79 Å) than that of Ti4+, an expansion of the TiO2 unit cell shall occur yielding an increase of the lattice parameters. Chanda et al. has proven this assumption through their structural and magnetic studies on Co-doped TiO2 (Chanda et al., 2018). Thus, it is plausible to consider in the present case that the substitution of Ti4+ sites occurs by smaller ionic radius Co3+ leading to a volume reduction of TiO2-lattice. Therefore, an overall volume contraction of ~8% is reasonable to obtain through Co3+-doping of TiO2. This promotes the conversion of anatase to rutile because the reconstructive anatase-to-rutile transformation involves a contraction of the c-axis (Hanaor and Sorrell, 2011).
Figure 1B displays the Raman spectra of the samples that were obtained between the wavenumbers of 200–900 cm−1. The Raman lines at 197, 390, 511, 637 cm−1 can be assigned to Eg, B1g, A1g, or B1g, and Eg modes of anatase phase, respectively, which confirm that the phase condition of our Co-doped TiO2 samples belongs to the tetragonal form of anatase phase. Moreover, the spectra show that the peak intensities decrease drastically after doping. As XRD results showed that the main phase for 0.5 Co-doped TiO2 is anatase, the decrease of intensity observed in the Raman spectra can be attributed to the increase of oxygen vacancies which occurred through the substitution of Ti4+ by Co3+ to balance the charge neutrality, relying on the difference between their ionic charges. These vacancies lead to the lattice distortion and results in a change of the diffraction intensity. Similar results were reported by Chanda et al. (2018). On the other hand, the XRD measurement revealed that the sample 1 Co-doped TiO2 contains rutile as the major polymorph as anatase was only present in a very small quantity. This may explain the decrease observed at the Raman spectra of this composition. Moreover, the Raman spectra of 1 Co-doped TiO2 yielded a smaller shift toward lower wavelengths while new peaks (436 cm−1) appeared indicating the presence of rutile polymorph, as mentioned in literature (Niilisk et al., 2006).
Figure 1C shows the morphology of the synthetized powders. The microscopic investigation revealed the formation of spherical nanoparticles with sizes around 70 nm that tend to agglomerate at the undoped powder samples. Doping with Co appears to influence the powder morphology. As the sample 0.5 Co-doped TiO2 shows more agglomerated spherical particles, the sample 1 Co-doped TiO2 presented larger and well-faceted rhombohedral crystallites with less agglomeration.
The BET-measured specific surface area of the synthetized samples were 8, 7, and 4 m2/g for undoped TiO2, 0.5 Co-doped TiO2, and 1 Co-doped TiO2, respectively. The surface area decreases with the increasing cobalt content, as the greatest decrease being with the 1 Co-doped TiO2 that corresponds to the particle size increase as observed by SEM analysis (see Figure 1C).
The sensing properties of the undoped and doped TiO2 were investigated toward 200 ppm NO2 and 200 ppm H2 at 600°C using synthetic air as carrier gas. This temperature was chosen on the basis of our previous results obtained with undoped and Al- and Cr-doped TiO2 relying on the fact that undoped TiO2 is capable of NO2-sensing at above 400°C (Saruhan et al., 2013; Gönüllü et al., 2015). In fact, as the temperature increases, the sensor signal and also the response time decreases. Accordingly, our present results indicate that the equilibrium temperature for sensing is at 600°C. Additionally, it is known that the TiO2 polymorphs will change at temperatures above 700°C. In order to avoid any temperature-induced complexity related to polymorph and electronic structures and to emphasize the effect of Co-dopant on the sensing properties, the operating temperature has been kept below 700°C.
As Figure 2A shows, the sensors yield higher response toward H2 than NO2. The H2 sensor responses are 42, 23, and 33% for undoped, 0.5 Co-doped TiO2 and 1 Co-doped TiO2, respectively. The undoped sample shows the highest response toward H2. The presence of Co-dopant seems to decrease the H2-sensing performance of TiO2 even though doping creates more oxygen vacancies in TiO2. This behavior can be attributed to the increase of rutile polymorph content on doping with cobalt, as previously reported, rutile is the less active (in term of functional properties) polymorph of TiO2 (Luttrell et al., 2014). On the other hand, the 1 Co-doped TiO2 which contains predominantly rutile polymorph showed a higher response toward H2 than 0.5 Co-doped TiO2. This discrepancy can be explained by alteration of the conductivity from n-type to p-type. In fact the dynamic response of the sensors toward H2 given in Figure 2B reveals that undoped and 0.5 Co-doped TiO2 exhibit n-type semi-conductivity (i.e., their electrical resistance decreases upon interaction with hydrogen) while the 1 Co-doped TiO2 yields p-type conductivity (its electrical resistance increases when reducing gas is introduced).
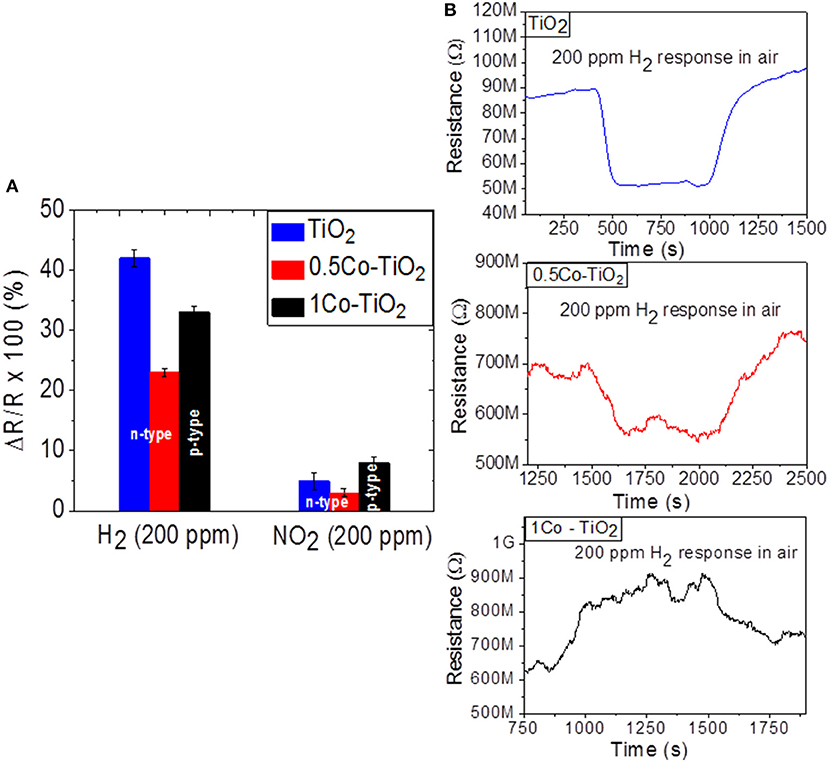
Figure 2. Sensor response of undoped and Co-doped TiO2 toward (A) NO2 and H2 at 600°C and (B) the relevant dynamic responses toward H2.
The sensor responses measured toward the oxidizing gas NO2 were 5, 3, and 8% for undoped, 0.5 Co-doped TiO2 and 1 Co-doped TiO2, respectively. In the case of NO2 sensing, the 1 Co-doped TiO2 showed the highest response. This may be mainly due to the electronic alteration of TiO2 from n to p-type semiconductor. Previous literature points out that this alteration can be utilized for the detection of oxidizing gas (Huusko et al., 1993). Our current results confirm that the dominant factor for the gas sensing property of the Co-doped TiO2 depend on the existing polymorphs as well as the nature of target gas (oxidizing or reducing). In the case of reducing gases, the type of polymorphs has more influence on the gas sensitivity than the type of electronic structure, while an opposite trend can be observed for oxidizing gases.
Considering these preliminary results, sensing properties of the undoped TiO2 toward H2 and of 1 Co-doped TiO2 toward NO2 are further investigated at 600°C. Figures 3A,C display the dynamic response of the sensor with undoped TiO2 at 600°C to various H2 concentrations (2,500, 5,000, and 10,000 ppm) and those with 1 Co-doped TiO2 to various NO2 concentrations (100, 200, and 400 ppm), respectively. As these response measurements exhibit, upon gas exposure, the sensor reaches to an equilibrium resistance value and settles back almost to the original baseline resistance value when the test gas is vented. This sequence has been reproduced for all the applied gas concentrations, demonstrating very good reproducibility and stability at the sensor behavior. For undoped TiO2, the calculated sensor signals were 58% for 2,500, 64% for 5,000, and 72%, for 10,000 ppm of H2, while for 1 Co-doped TiO2, the sensing signal was 32% for 100, 40% for 200, and 55% for 400 ppm of NO2, respectively. These investigations indicate that the gas response increases with increasing concentration of NO2 suggesting the capability of the sensor for quantitative analysis. In order to confirm the stability and reproducibility of the synthesized undoped TiO2 and 1 Co-doped TiO2 powders at the same H2 and NO2 concentrations, respectively, the sensor tests were repeated by introducing into the test chamber three times 10,000 ppm H2 (for undoped TiO2) and 200 ppm NO2 (for 1 Co-doped TiO2) under the same test conditions. The dynamic curves of these investigations are shown in Figures 3B,D. The average value of the gas response is 70% for H2 and 38% for NO2 for undoped TiO2 and 1 Co-doped TiO2 respectively, with a negligible deviation. Even though the baseline resistance changes slightly with time, the stability of the sensors is maintained well.
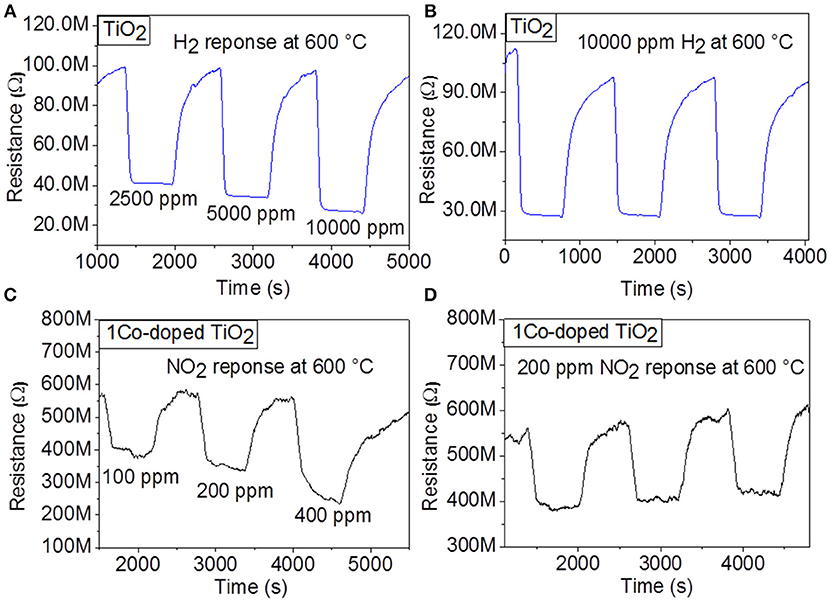
Figure 3. Dynamic response of gas sensors based on TiO2 and 1Co-doped TiO2 to various concentrations of (A) H2, (C) NO2, respectively, and to (B) 10,000 ppm H2 and (D) 100 ppm NO2, respectively at 600°C.
Conclusion
This paper reports the successful synthesis of undoped and Co-doped TiO2 nanoparticles by a facile co-precipitation route through the use of oxalic acid. According to the X-ray diffraction and Raman spectroscopy analysis, the substitution of Ti4+ by Co3+ creates oxygen vacancies and promotes the anatase-to-rutile transformation. The sample 1 Co-doped TiO2 which reveals p-type conductive behavior yields an enhanced NO2 response at 600°C under air as carrier gas. However, the undoped TiO2 shows n-type semiconductor behavior with the highest sensor response toward H2 at 600°C. The enhancement of high temperature NO2 sensing performance is related to the alteration in electronic structure as well as the formation of rutile polymorph in the 1 Co-doped TiO2 sample. It has been a remarkable effect to obtain two different behaviors by only addition of very small quantities of Co-dopant to TiO2 under the same processing conditions. If the Co-dopant content exceeds 1%, CoTiO3 starts to form as a secondary phase introducing a new parameter to take into consideration. For the perspective of NO2 sensing optimization, Co-dopant contents between 0.5 and 1 mol.% may also be considered.
Data Availability Statement
All datasets generated for this study are included in the manuscript/supplementary files.
Author Contributions
RL has synthesized and characterized the material for sensor application. BS have initiated the idea of applying the Co-dopant to TiO2 and gave Feedback in evaluating the sensing mechanism with this material.
Conflict of Interest
The authors declare that the research was conducted in the absence of any commercial or financial relationships that could be construed as a potential conflict of interest.
Acknowledgments
The authors thank Rene Breuch and Johannes Warmer of H-BRS for Raman Spectroscopy measurements. The grant provided by the DLR-DAAD Fellowship program under no. 284 is acknowledged.
References
Chanda, A., Rout, K., Vasundhara, M., Joshi, S. R., and Singh, J. (2018). Structural and magnetic study of undoped and cobalt doped TiO2 nanoparticles. RSC Adv. 8, 10939–10947. doi: 10.1039/C8RA00626A
Chen, X., and Mao, S. (2007). Titanium dioxide nanomaterials: synthesis, properties, modifications, and applications. Chem. Rev. 107, 2891–2959. doi: 10.1021/cr0500535
Dey, A. (2018). Semiconductor metal oxide gas sensors: a review. Mater. Sci. Eng. B 229, 206–217. doi: 10.1016/j.mseb.2017.12.036
Esmaeilzadeh, J., Marzbanrad, E., Zamani, C., and Raissi, B. (2012). Fabrication of undoped-TiO2 nanostructure-based NO2 high temperature gas sensor using low frequency AC electrophoretic deposition method. Sens. Actuat. B 161, 401–405. doi: 10.1016/j.snb.2011.10.051
Gönüllü, Y., Haidry, A. A., and Saruhan, B. (2015). Nanotubular Cr-doped TiO2 for use as high-temperature NO2 gas sensor. Sens. Actuat. B 217, 78–87. doi: 10.1016/j.snb.2014.11.065
Hanaor, D. A. H., and Sorrell, C. C. (2011). Review of the anatase to rutile phase transformation. J. Mater. Sci. 46, 855–874. doi: 10.1007/s10853-010-5113-0
Huusko, J., Lantto, V., and Torvela, H. (1993). TiO2, thick-film gas sensors and their suitability for NOx monitoring. Sens. Actuat. B 16, 245–248. doi: 10.1016/0925-4005(93)85188-G
Luttrell, T., Halpegamage, S., Tao, J., Kramer, A., Sutter, E., and Batzill, M. (2014). Why is anatase a better photocatalyst than rutile? Model studies on epitaxial TiO2 films. Sci. Rep. 4:4043. doi: 10.1038/srep04043
Niilisk, A., Moppel, M., Pärs, M., Sildos, I., Jantson, T., Avarmaa, T., et al. (2006). Structural study of TiO2 thin films by micro-Raman spectroscopy. Cent. Eur. J. Phys. 4, 105–116. doi: 10.1007/s11534-005-0009-3
Saruhan, B., Yüce, A., Gönüllü, Y., and Kelm, K. (2013). Effect of Al doping on NO2 gas sensing of TiO2 at elevated temperatures. Sens. Actuat. B 187, 586–597. doi: 10.1016/j.snb.2013.04.111
Wang, C., Yin, L., Zhang, L., Xiang, D., and Gao, R. (2010). Metal oxide gas sensors: sensitivity and influencing factors. Sensors 10, 2088–2106. doi: 10.3390/s100302088
Wisitsoraat, A., Tuantranont, A., Comini, E., Sberveglieri, G., and Wlodarski, W. (2009). Characterization of n-type and p-type semiconductor gas sensors based on NiOx doped TiO2 thin films. Thin Solid Films 517, 2775–2780. doi: 10.1016/j.tsf.2008.10.090
Keywords: co-precipitation, high-temperature sensor, nitrogen oxides, hydrogen, electronic structure, co-doped TiO2
Citation: Lontio Fomekong R and Saruhan B (2019) Synthesis of Co3+ Doped TiO2 by Co-precipitation Route and Its Gas Sensing Properties. Front. Mater. 6:252. doi: 10.3389/fmats.2019.00252
Received: 07 June 2019; Accepted: 25 September 2019;
Published: 10 October 2019.
Edited by:
Zoltán Kónya, University of Szeged, HungaryReviewed by:
Josep Albero, Instituto de Tecnología Química (ITQ), SpainAnita Lloyd Spetz, Linköping University, Sweden
Copyright © 2019 Lontio Fomekong and Saruhan. This is an open-access article distributed under the terms of the Creative Commons Attribution License (CC BY). The use, distribution or reproduction in other forums is permitted, provided the original author(s) and the copyright owner(s) are credited and that the original publication in this journal is cited, in accordance with accepted academic practice. No use, distribution or reproduction is permitted which does not comply with these terms.
*Correspondence: Bilge Saruhan, YmlsZ2Uuc2FydWhhbkBkbHIuZGU=