Recent Advances in Fluorescent Silk Fibroin
- 1Institute of Nano Bio Regenerative Medicine, Hallym University, Chuncheon, South Korea
- 2Department of Otorhinolaryngology-Head and Neck Surgery, Chuncheon Sacred Heart Hospital, School of Medicine, Hallym University, Chuncheon, South Korea
Silk is a natural macromolecular protein consisting of fibroin and sericin. Silk fibroin (SF), derived from Bombyx mori, is a representative fibrous protein that has been used mainly in fashion textiles and surgical sutures. Also, SF has been extensively applied as a potential biomaterial in a number of biomedical and biotechnological fields, as SF can be reconstituted in various forms through physical and chemical processes. In addition to direct use of SF with intrinsic structure and properties, there are many researchers attempt to insert more novel functional properties into SF, despite retaining its favorable natural characteristics. In recent years, fluorescent silk obtained through various methods, such as the genetic modification or dye feeding method, has been applied in a variety of medical fields. These functionalized silks have properties that can be applied to new and versatile fields more than currently existing fields such as drug delivery, monitoring surgical and wound healing processes. This review focuses on the preparation methods and the latest technological advances on the use of fluorescent SF materials, especially their biomedical applications.
Introduction
SF from the Bombyx mori silkworm is a block copolymer and consists of 26 kDa light and 390 kDa heavy chain joined by a disulfide bridge (Yang et al., 2004; Vepari and Kaplan, 2007). The sequence of SF amino acid includes repetitive Gly–Ala–Gly–Ala–Gly–Ser repeats that can be self-assembled into aa β-sheet structure (Heslot, 1998; Zhou et al., 2001). SF has been highlighted as a functional biomaterial with excellent biocompatibility, remarkable mechanical properties such as low inflammation-inducing effect, excellent water vapor with oxygen permeability, blood compatibility, and controllable degradation.
Until now, SF has been fabricated into various forms for different applications. For example, SF has been used to make films, sponges, electrospun mats, nano- or microparticles, and hydrogels (Rockwood et al., 2011; Sultan et al., 2018). Because of these features, SFs are increasingly being studied for biomedical uses, including bone, tendon, ligament, cartilage, skin, nerve, cornea, tympanic membrane, dental, artificial kidney, and bladder (Altman et al., 2003; Vepari and Kaplan, 2007; Kim et al., 2010; Rockwood et al., 2011; Lee et al., 2014; Font Tellado et al., 2017; Lee et al., 2017; Park et al., 2018; Tang et al., 2018; Yeon et al., 2018; Sultan et al., 2019). Recently, to outfit a number of applications, SF has been chemically modified through several aqueous or organic solvent-processing methods, resulting in the change of its chemical, physical, and mechanical properties (Murphy and Kaplan, 2009; Li et al., 2012). Chemical modifications of SF through coupling reactions, amino acid modification, grafting reactions have been displayed to change characteristics of silk material, including hydrophilicity, beta-sheet self-assembly, materials structure, and solution feature.
During the last decade, several efforts have been conducted for the modification of the surface and functionalization of SF using a vast wide range of technology and materials with functional properties to meet the worldwide market demands of natural and smart materials. Successful surface modification of silk can be overcome its intrinsic shortcomings with enhanced end-user performance. Surface modifications of SF are achieved through physical techniques (such as UV, Gas, Plasma treatment), chemical approaches (such as grafting copolymerization techniques, and chemical agents including acid anhydrides and polycarboxylic acids) (Li et al., 2012). Through surface modification, silk products with improved performance in the field of fibers and biomaterials can be obtained. This technology allows us to regulate cell response and improve cell adhesion to silk substrates.
UV irradiation is a very effective method of surface alteration for industrial uses, owing to its big excitation spectrum, multiple wavelengths availabilities, and cost-effective (Bergonzo et al., 1992; Ying Zhang et al., 1993; Periyasamy et al., 2007a). Surface modification of the SF has been achieved using UV treatment to enhance adhesion and wettability without any significant losses in crystallinity, mass, and strength (Park et al., 2007; Periyasamy et al., 2007a, b). An adequate increment of hydrophilicity of SF materials would be feasible for cell proliferation and adhesion. Simultaneously, some of the essential properties of silk clothes could be altered by UV irradiation.
Gas cures can impart various properties to SF dependent on the gas species used. For instance, ozone treatment has been used to increase the flexibility and yellowness indices of both fresh and degummed silk materials (Sargunamani and Selvakumar, 2006). Under atmospheric pressure, the use of ammonia-gas to treat SF materials increased the softness without any change of the apparent dyeing rate and crystallinity (Lee et al., 2006). Plasma, a hot ionized gas consisting of a mixture of electrons, also has been used to surface modification of SF materials. For example, the hydrophobicity of SF was increased when SF was treated with hexafluoropropene (C3F6) plasma (Li and Jinjin, 2007).
Chemical modifying agents such as acid anhydrides and polycarboxylic acids can produce chemical bonds by reacting with basic amino acids in SF. These chemical linkages can advance the hydrophobicity, thermal stability, and increase recovery of the SF materials without any significant effect on mechanical properties (Arai et al., 2001a, 2003; Davarpanah et al., 2009). Like these chemical agents can also use to graft other materials onto SF fibers create a novel silk material completely new properties. Acid anhydrides and polycarboxylic acids have also been used as bridge linkages to graft polysaccharide chitosan onto SF. This chitosan grafted SF demonstrated antibacterial activity and increased dyeability (Marsh et al., 1955; Arai et al., 2003). Other chemical agents, including EPSIA (a silicone-containing epoxy crosslinking agent) and isocyanates, have also been used to surface modifications of SF materials (Otterburn, 1977; Maclaren, 1981; Arai et al., 2001b; Cai et al., 2001).
Over several decades, fluorescent proteins have been used as a biotechnological tool to explore the function of a particular gene of interest through directly monitoring, visualizing, and measuring protein expression in living cells. Fluorescent SF has gained numerous attention for its applicability in various biotechnological and medical applications due to its inherent fluorescence property achieved through the insertion of the fluorescence gene into silk cocoons. The addition of the fluorescence properties into SF via gene insertion escapes the conjugation step to insert a fluorescently tagged protein such as green fluorescent protein (GFP). Therefore, the introduction of fluorescent properties in SF offers several potential advantages over the fluorescently tagged protein.
In the past decade, many studies have been reported to make silkworm silk with special characteristics for its application in new fields. Dyeing methods for obtaining silk of various colors are typically harsh conditions involving high temperature and pH changes and require additional steps to restore the original properties of silk. Recently, a method has been developed that produces functional silks that biologically introduce dyes into SF (Tansil et al., 2011b). Otherwise, the modification of silk proteins by genetic engineering has attracted much attention (Kikuchi et al., 1992; Yamao et al., 1999; Zhang et al., 1999; Zhao et al., 2001). Typically, a GFP is gene-targeted with the fibroin gene to obtain green fluorescent silkworm cocoons (Zhao et al., 2001). Bulk production of three colors (red, green, and orange) of fluorescent silks has been achieved through transgenic silkworms (Iizuka et al., 2013). These fluorescent silks of different colors can be potentially useful in medical applications and as silk fiber for clothing.
Therefore, in the current review, we summarize the preparation approaches of several fluorescent silk materials, present an updated review of the cutting-edge methodological advances on the use of fluorescent silk in biomedical applications. Fluorescent SF with properties and utility unattainable with conventional silk materials would have enormous potential and will offer biomedical applications, including drug delivery, bio-imaging, and sensing.
Preparation Methods of Fluorescent Silk Materials
Natural (Autofluorescence)
When silkworms eat mulberry leaves, various pigments are absorbed into the silkworm body, including various nutrients. Silkworms can produce yellow fluids and cocoons by binding the yellow carotenoid (among the pigments absorbed into the silkworm body) pigment to the intracellular carotenoid-binging protein (CBP). The Y (Yellow blood) gene is the determinant of the cocoon color in the silkworm Bombyx mori and controls the uptake of carotenoids into the intestinal mucosa and the silk gland. Sakudoh et al. (2007) reported that colorless hemolymph and white cocoons resulted in the Y recessive strain, due to an improper mRNA splicing that appeared by a transposon-associated genomic omission, creates an incompetent CBP mRNA. Mainly, the pigments are integrated into the sericin with a little absorption into fibroin. White SF produced due to the removal of sericin by a degumming process. However, there is considerable confirmation presenting that silk cocoons have a fluorescent nature, generally due to the existence of flavonoids, including quercetin derivatives (Tamura et al., 2002; Daimon et al., 2010; Kusurkar et al., 2013) and carotenoid compounds (Harizuka, 1953). It has been evident that like silk cocoons, SF-derived scaffolds exhibit a fluorescence index in UV, green, and blue, lights (Mandal and Kundu, 2009; Zhang et al., 2010; Kundu et al., 2012; Hodgkinson et al., 2014; Bhardwaj et al., 2015). Since the SF scaffold releases fluorescence at wavelengths identical in color to the fluorescent dyes themselves, the intrinsic fluorescence of SF can interfere with the signal from such fluorescent dyes (Georgakoudi et al., 2007).
Amirikia et al. (2018) fabricated an SF scaffold using the freeze-drying method and examined the fluorescence of the SF scaffold both prior and after cell seeding and fluorescent labeling using confocal microscopy. They subsequently explored the fluorescent index of the SF scaffold chemically. Cells labeled with fluorophore seeded into the scaffold exhibited a similar fluorescent color as the scaffold itself when stimulated by a similar wavelength of light. SF solution showed absorption maxima at 277 nm while emission maxima 345 nm, in the UV-Vis and fluorescence spectroscopy, which is a typical protein-derived signal. Thus, they concluded that the fluorescence of the SF scaffold related to intrinsic protein fluorescence and not to flavonoid constituents of cocoons from which the SF protein was derived. In addition, Kusurkar et al. (2013) applied a “water glass” based approach to obtain the fluorophores from silk cocoons.
Feeding Method Using a Dye-Containing Diet
There is an early attempt to serving coloring matter to silkworms in the twentieth century (Edwards, 1921). This kind of study was to examine the persistence of mulberry leaves in the gut of silkworms (Lombardi, 1920) or the toxicity associated with particular dyes to silkworms (Campbell, 1932). Various studies demonstrated that orcein was up taken to silk cocoons from silkworm’s food, some other dyes were absorbed by guts or even traveled into eggs of the silkworm (Jucci and Ponsevernoi, 1930; Goris and Muehlemann, 1936). Numerous other tested dyes, including gentian violet, berlin blue, safranin, Nile blue, methylene blue, neutral red, alizarin, fuchsin, indigo carmine, and methyl orange were not taken up in silk (Jucci and Ponsevernoi, 1930). Due to the absence of proper and accurate characterization techniques, these above-mentioned studies results were not conclusive regards to the incorporation of the dyes into silk, particularly in SF. The molecular effect of these dyes or other additives into silk was not also correlated.
For the first time, rhodamine B as a dye uptake into the SF has been shown by Tansil et al. They fed silkworms on a dye-containing food, and confirmed via advanced characterization (Tansil et al., 2011a, b). Fluorescent silk cocoons and fibers were obtained by serving silkworms with a mixture of mulberry leaf powder and fluorescent molecules such as rhodamine B, rhodamine 101, and rhodamine 110. It was evident that SF can interact with rhodamine B, rhodamine 101, and rhodamine 110 by molecular recognition (Tansil et al., 2011b).
Genetic Modification
Recently genetically altered silks derived from engineered silkworms have been demonstrated (Yamao et al., 1999). Once the silkworm strain is developed, transgenic silkworms can be easily multiplied and retained. Transformed silkworm was first successfully developed by Tamura et al. (2000) using a constructed vector with a Piggy Bac transposon system derived from Trichoplusiani, a Lepidoptera insect. Also, the Piggy Bac transfer vector has been used to conduct many silkworm transformation studies so far. Among them, a transgenic silkworm produced recombinant silkworm cocoon containing human collagen protein has been reported in 2003 (Tomita et al., 2003). Expression systems were established to produce recombinant proteins using fibroin and sericin, respectively. Currently, fluorescent transgenic silkworms have been developed using various transformation vectors (Liu et al., 2009). The insertion of the transgene into the genome of silkworm allows obtaining specific desired characteristics via altering the silk protein (Tamura et al., 2000; Iizuka et al., 2013).
Several kinds of transformation vectors have been constructed using silk genes for the alteration of silk (Tomita, 2011). The vector using the fibroin L chain gene and the fibroin H chain gene is suitable for the fabrication of modified silks among the constructed vectors. Numerous useful silks with functional properties are produced using the vector and have been revealed to retain useful properties for medicines and fabrics. For instance, recombinant silkworm/spider silk produced by the transgenic silkworm is harder than normal silk fibers and as hard as a natural dragline (Teule et al., 2012). Recently biologically active silk constituted a chimeric protein of human fibroblast growth factor, and fibroin L chain has been stated to be applicable as a novel biomaterial for tissue engineering (Hino et al., 2006). The film derived from silk comprising with a chimeric protein of the fibroin L chain, and fibronectin sequence or a partial collagen peptide has been exhibited to have improved cell adhesion activity in comparison to original silk (Yanagisawa et al., 2007). It is crucial to develop a cost-effective production approach that produced enormous quantities of modified silk to expand the utility of transgenic silkworm silks. Iizuka et al. (2013) developed a transgenic silkworm that produces fluorescent silk and set up an isolation method to remove sericin at low temperatures to develop it into a suitable material that can be applied to various industrial fields. Fluorescent silk dresses and lights were produced based on this technology (Figure 1). Kim et al. developed a microcarrier by inserting EGFP of jellyfish into exon 2 of the heavy chain gene of silkworm fibroin. The green fluorescence remains intact, and the green fluorescence gene is transmitted to the next generation. They also have developed transgenic silkworm expressing the red and blue fluorescent protein (Kim et al., 2012, 2013, 2014).
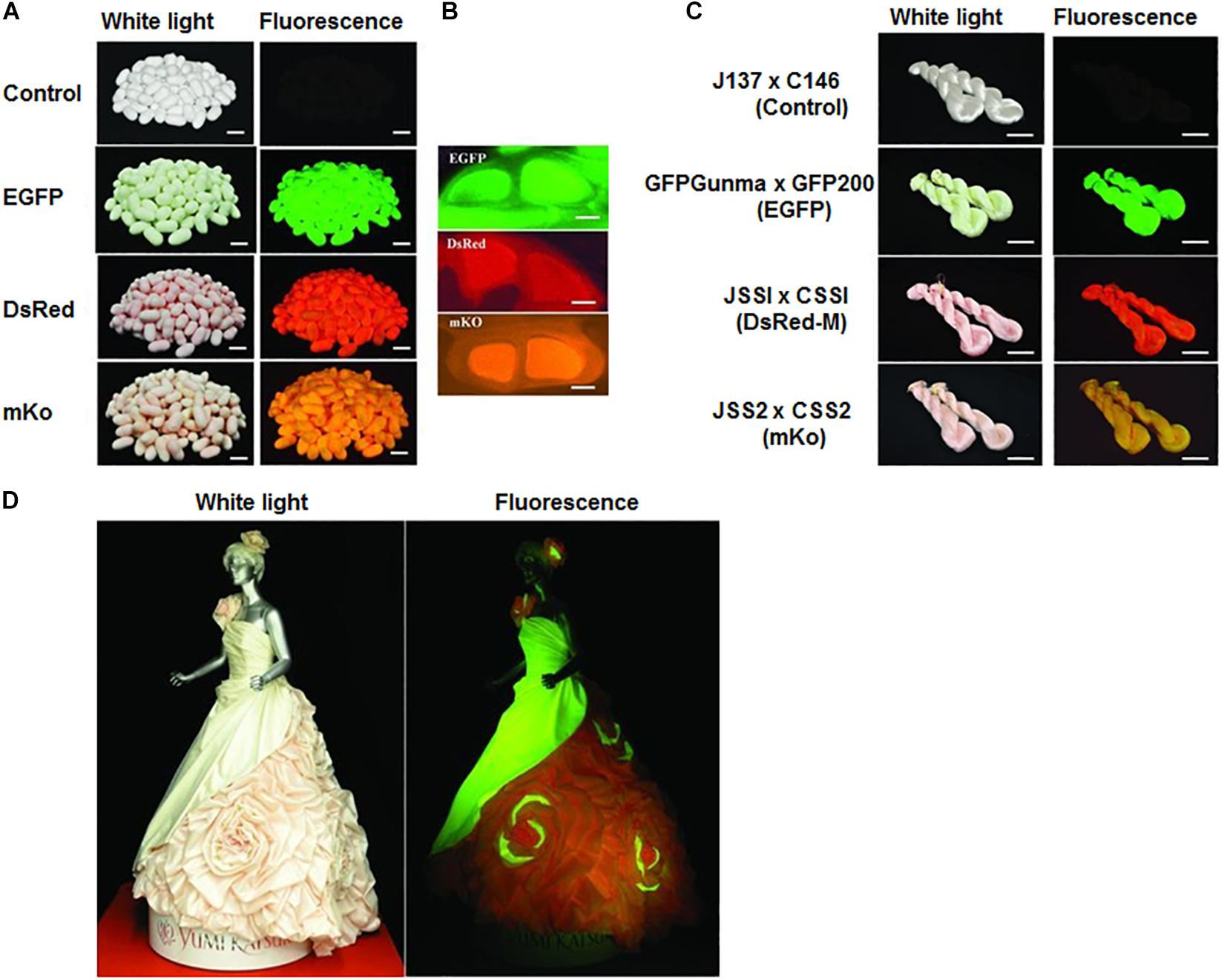
Figure 1. Colored Fluorescent Silk Made by Transgenic Silkworms. (A) Colored fluorescent cocoons and (B) cross-section of the cocoon silk produced by the hybrid races. (C) Fluorescent silks. (D) Wedding dress made by the colored fluorescent silks. Reproduced from Iizuka et al. (2013) with permission from John Wiley and Sons.
Post-treatment
Fixation of inorganic solvent particles on silk fibers is another method for obtaining fluorescent SF. Chu and Liu demonstrated a novel method of preparing fluorescent silkworm silks by integrating semiconductor nanocrystals quantum dots (QDs) into the silks through electrostatic absorption using a fixer of a polyelectrolyte (PE) (Chu and Liu, 2008). Therefore, silkworm silks incorporated with QDs had adjustable colors from green to near-infrared, which penetrated via an opaque pigskin of ∼3.5 mm in thickness. Ko et al. fabricated a novel SF-based carbon quantum dots (SF-CQDs), which was synthesized over a short time (20 min) via a facile microwave-irradiation method (Ko et al., 2018). These SF-CQDs exhibited good crystallinity, water dispersity, low toxicity, photoluminescence stability, optical properties, and biocompatibility.
Recently, several studies have been used the addition of dye into the SF solution method to develop dye-doped SF in the forms of nanoparticles, films, and electrospun (Kaneko et al., 1991; Myung et al., 2008; Min et al., 2017). Kaneko et al. (1991) incorporated luminescence from the excitation state of Ru (bpy)32+ (bpy, 2,2′-bipyridine) into an SF membrane by casting a mixture of aqueous solutions of a 1 mM Ru (bpy)3 2+ and 2.8 wt.% SF. The excitation lifetime of the incorporated dye complex into an SF membrane exhibited lengthier (1700 ns) than the value (598 ns) in aqueous solution, suggesting the existence of an active site for the complex binding. Four water-soluble dyes, including riboflavin, sodium fluorescein, rhodamine B, and stilbene 420, were used to develop fluorescent silk nanofibers (FSNs) mat through the electrospinning method. This red-green-blue (RGB) fluorescent nanofibrous mats were successfully used for acid vapor detection and nutrition delivery (Min et al., 2017).
Although several methods, including feeding, genetic modification, and post-processing, have been successfully applied to obtain florescent SF, there are some advantages and disadvantages of each method. The feeding method using a dye-containing diet is simple but not conclusive regards to the addition of the dyes into SF. Numerous studies have been effectively utilized the genetic modification method to obtain fluorescent SF as this method offers the addition of the desired properties correctly. However, this transgenic method suffers from several limitations, including low efficiency, high cost, and the complexity of the process. Moreover, transgenic silkworms sometime fail to spin silks (Zhang et al., 1999). The post-processing technique offers several advantages, for example, a profoundly productive and effectively reproducible procedure with low cost in contrast with the dye feeding method or transgenic strategy. However, the post-processing method also suffers from some drawbacks due to the release of some toxic ions from the inserted molecules into the SF such as QDs (Chu and Liu, 2008).
Applications of Fluorescent Silk Materials
SF-based biomaterials have been extensively explored in biomedical fields, including cartilage and bone, drug delivery, vascular grafts, skin tissue, wound dressings, ligaments, cornea, tympanic membrane, and artificial kidney. SF has been incorporated with different materials or been modified by chemically/genetically to produce fluorescence SF materials for a wide range of applications (Table 1). There have been numerous studies on the applications of fluorescent SF in the biomedical and biotechnological fields.
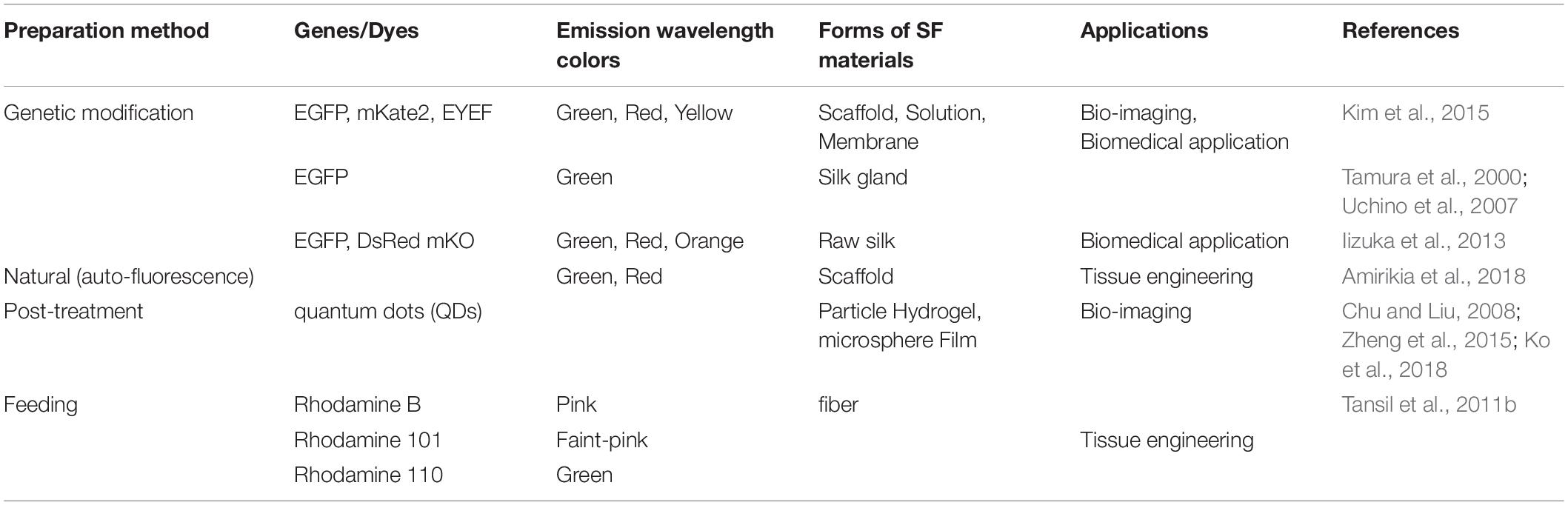
Table 1. A brief summary of fluorescent SF-based materials and their potential biomedical applications.
Bio-Imaging
The distribution or degradation of biomaterials tracking is vital for tissue engineering and drug delivery. In the recent past, the use of fluorescent polymeric nanoparticles (PNPs) for bio-imaging has received ample attention for non-invasive tracking (Win et al., 2015). In an early study, QDs containing SF hydrogels were prepared from CdTe QDs (MPA-QDs) incorporated SF solution (Zheng et al., 2015). The integration of QDs into SF hydrogels considerably reduced the cytotoxicity with the significantly stable fluorescent property. This QDs containing silk hydrogels with established and robust fluorescence have prospective applications in tracking the distribution and degradation of SF-based biomaterials, and also as a suitable diagnostic tool. Nanodiamonds (NDs) have also been applied for bio-imaging due to its bright extensive fluorescence emission feature (Khalid et al., 2014; Xu et al., 2019).
In recent times, the use of Two-Photon Fluorescence (TPF) microscopy for bio-imaging has gained an ample interest due to numerous significant benefits over currently existing detection technologies, including and transmission electron microscopy (TEM) and scanning electron microscopy (SEM) (He et al., 2008). In biological imaging, silk films integrated with TPF properties could be applied as a film scaffold. To achieve this objective, the uniform distribution of the TPF QDs are essential in the silk film. The silk films composite with QDs could be used to observe activities underneath the deep tissue in a non-invasive and real-time manner (Lin et al., 2015). Lin et al. (2015) have also been developed a novel and versatile material-assembly approach to achieve fluorescent release SF biocomposite materials that could be used in non-invasive scaffold imaging.
Kim et al. (2015) have shown, for the first time, a fluorescent SF solution preparation method for biomedical applications (Figure 2). This work stated an approach of preparing a fluorescent SF solution and various forms, including membrane, sponge, particle, and electrospun fiber (Figure 3). The transgenic fluorescent cocoons exhibited the fluorescent protein’s color when exposed to a blue LED light. The fluorescent cocoons were treated at 60°C overnight in an aqueous solution of 3% NaHCO3 with a proteolytic enzyme alcalase and then washed several times with distilled water to remove the sericin proteins. Then the extracted silk was melted in 1 mM DTT containing 9.5 M LiBr solution at 40°C. After that, the solution was filtered through a miracloth and dialyzed against distilled water to remove the salt for 2 days. The final concentration of the aqueous SF solution was 4 wt.%. The fluorescence studies were performed with the cocoons and the SF solution, and the fluorescence was captured at excitation wavelengths of 488 nm for EGFP, 514 nm for EYFP) and 588 nm for mKate2 and maximum emission wavelengths were detected at 507 nm for EGFP, 527 nm for EYFP, and 633 nm for mKate2, respectively. Similar excitation and emission characteristics were detected for the cocoons and solution. Soluble fluorescent SF was conjugated with anti p53 antibodies and used to detect the p53 (an early cancer biomarker) expression in HeLa cells (Figure 4). Cells were adhered to and grew well on the membranes, which were fabricated from the fluorescence SF solutions by casting them on polystyrene plates. The sponges prepared from the SF solutions were subcutaneously transplanted into the dorsal regions of the nude mice and shown to retain their fluorescence over 1 year, demonstrating the long-term biocompatibility of these biomaterials (Figure 5). Intraoperative use of EGFP fluorescent SF was also found in animal models to identify small esophageal and stomach perforation (Figure 6). Therefore, it can be imagining that functional fluorescent SF materials can be applied in a wide range of areas, such as optoelectronics, biomedical, and chemical science, in the future.
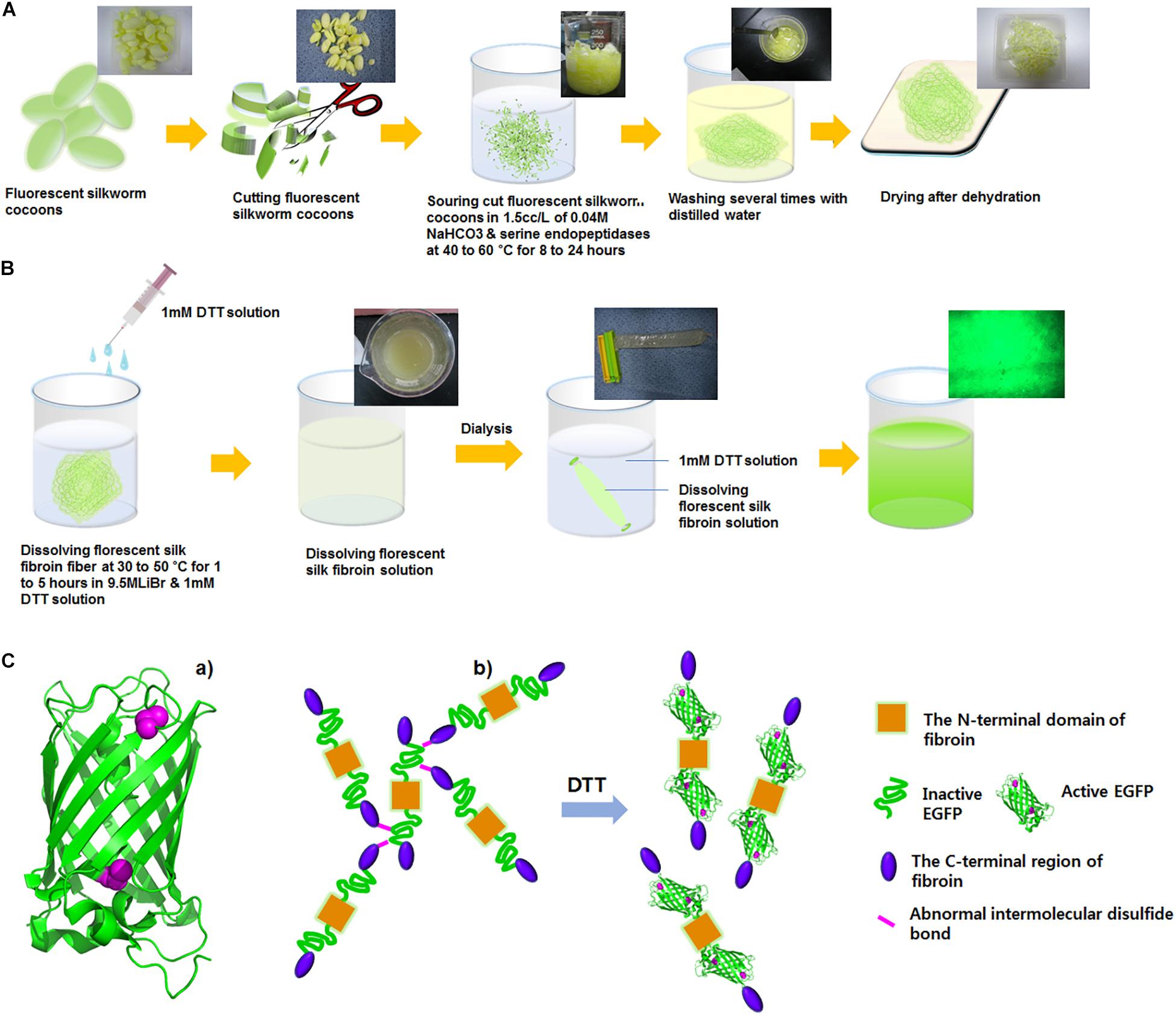
Figure 2. Preparation of fluorescent silk fibroin solution. (A) Fluorescent silk was degummed with 0.5% (w/w) Na2CO3 with 1 mM DTT solution at 45°C for overnight and then washed with distilled water. (B) Degummed fluorescent SF was dissolved in 9.5 M LiBr for 4 h at 45°C and dialyzed to remove salts in a cellulose tube against distilled water for 2 days at room temperature. (C) The critical role of DTT in the improvement of fluorescent activity of the fluorescent fibroin. (a) The EGFP structure (green ribbons) with two cysteine residues (magenta spheres). EGFP, EYFP, and mKate2 contain cysteine residues that are not involved in disulfide bonds. (b) A model for the improvement of fluorescence activity of the fluorescent fibroin in the presence of DTT. DTT would allow EGFP to be properly folded and to exert fluorescent activity by preventing the formation of abnormal intermolecular disulfide bonds. Reproduced from Kim et al. (2015) with permission from ELSEVIER.
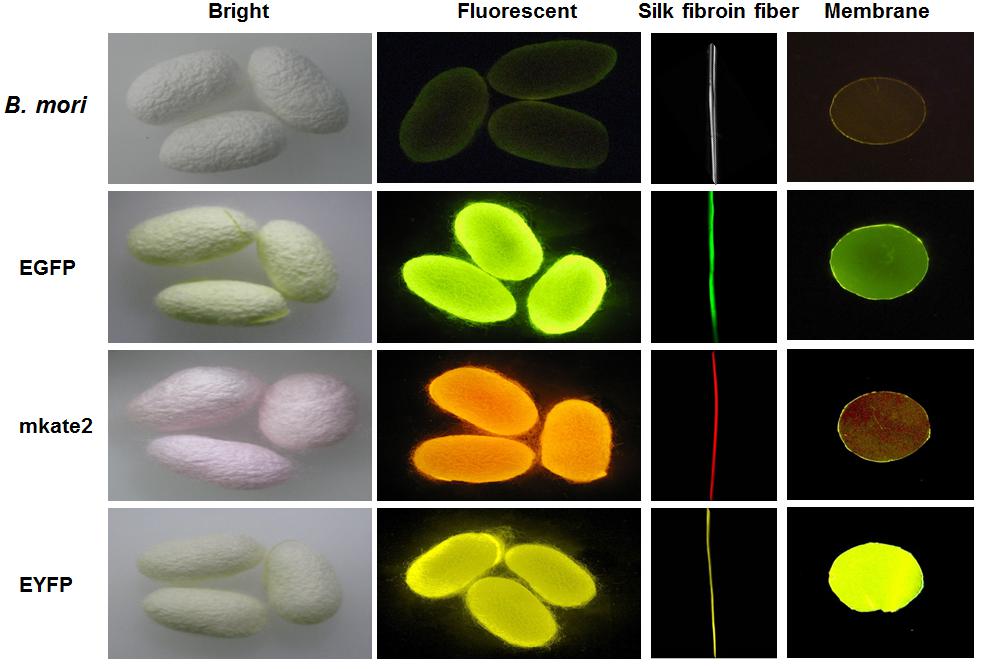
Figure 3. Fluorescent cocoons, silk fibroin fibers, and membranes. The fluorescent cocoons produced by transgenic silkworms had various fluorescent proteins, including EGFP, mKate2, and EYFP. Reproduced from Kim et al. (2015) with permission from ELSEVIER.
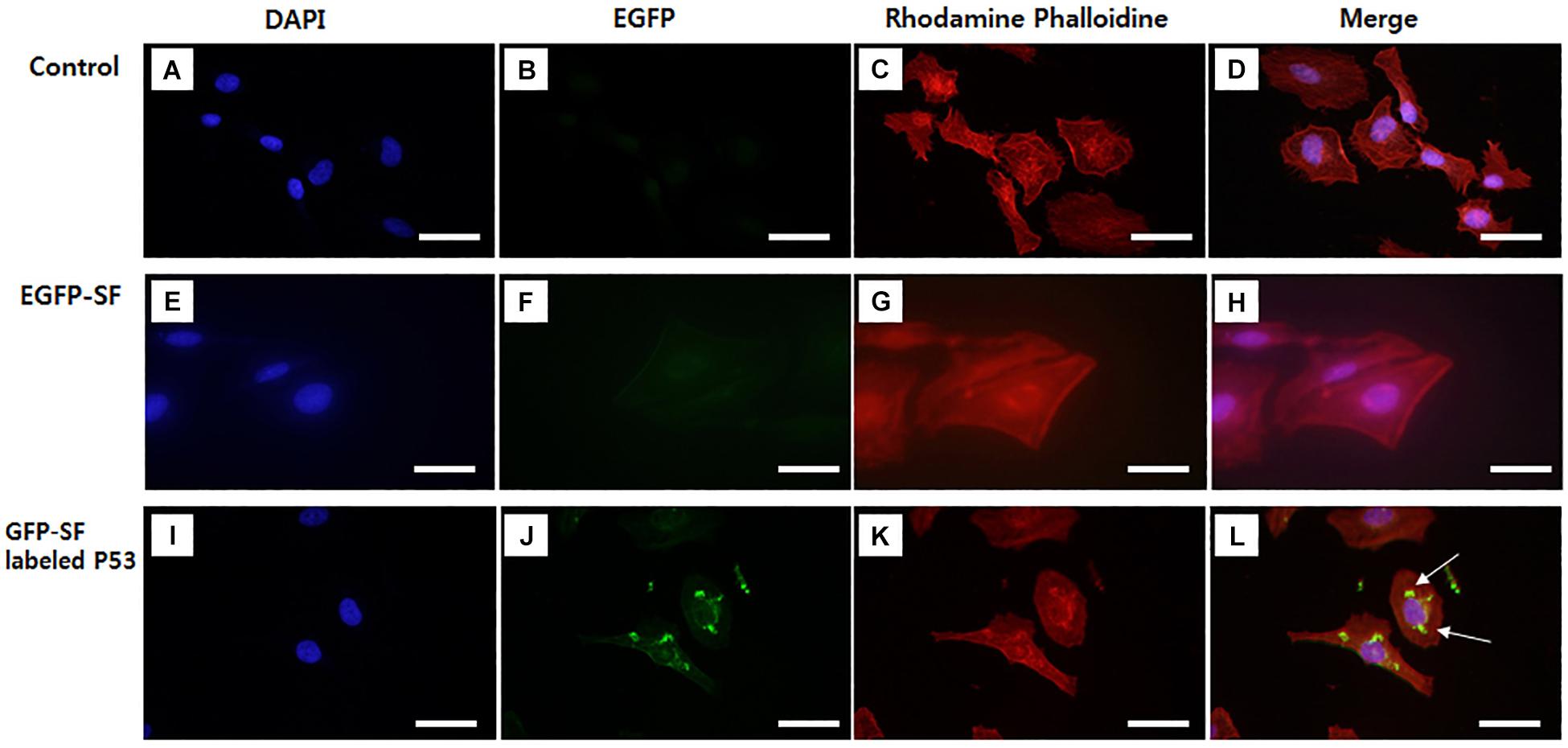
Figure 4. Images of p53 localization using EGFP fluorescent SF labeled p53 antibody in HeLa cell. (A–D) Control, (E–H) EGFP fluorescent SF solution, (I–L) EGFP fluorescent SF labeled p53. HeLa cells were stained with (A,E,I) DAPI and (C,G,K) Rhodamine Phalloidin and imaged under fluorescence microscopy, (D,H,L) DAPI, GFP, and Rhodamine Phalloidin images were merged. White arrows are the image of EGFP-SF labeled P53 in Hela cell. Scale bar = 50 μm. Not published.
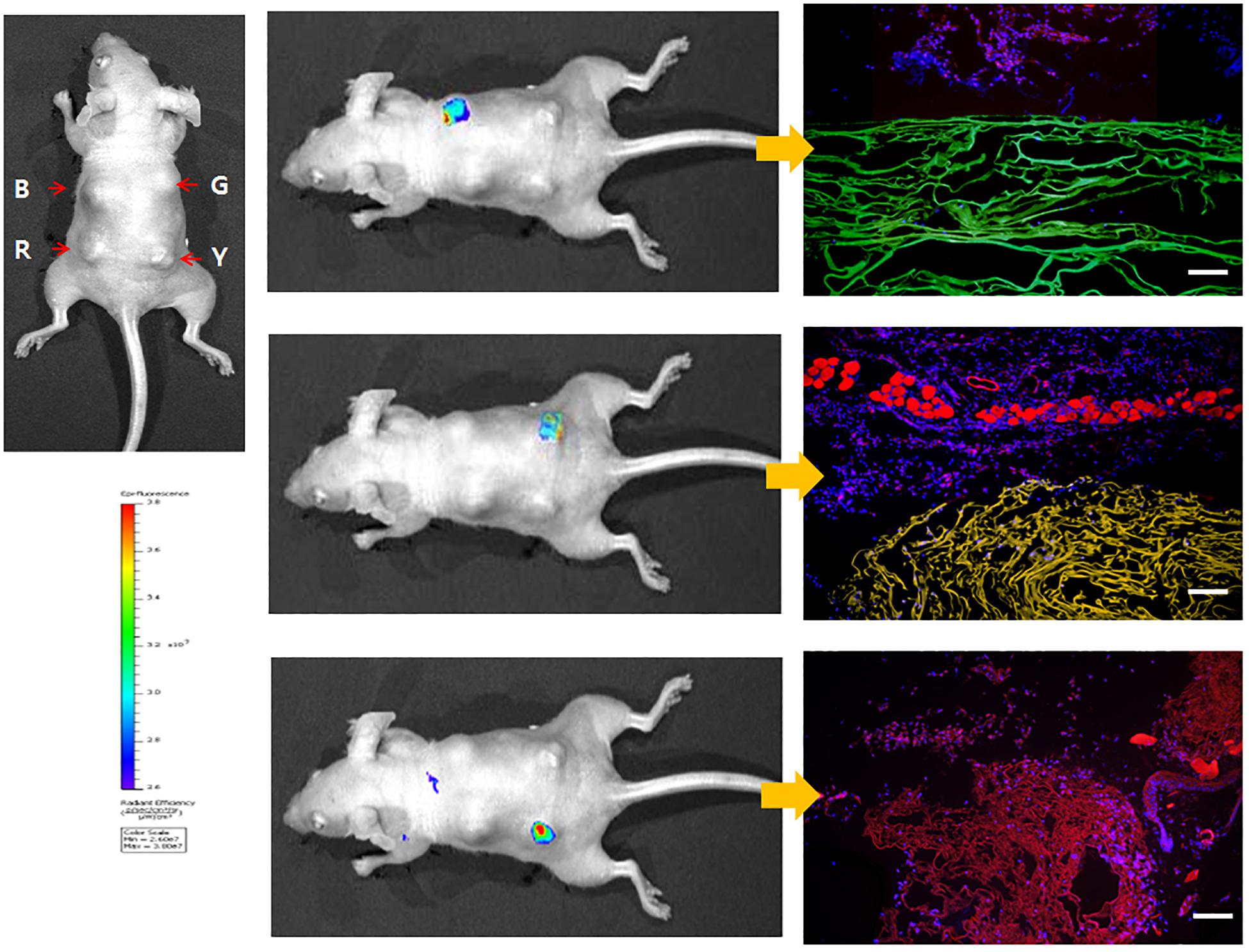
Figure 5. Whole-body imaging of fluorescent SF sponges in the dorsal region of nude mouse at 7 days. The color bar indicates relative signal intensity. (B) B. mori, (G) EGFP, (R) mKate2, (Y) EYFP. Reproduced from Kim et al. (2015) with permission from ELSEVIER.
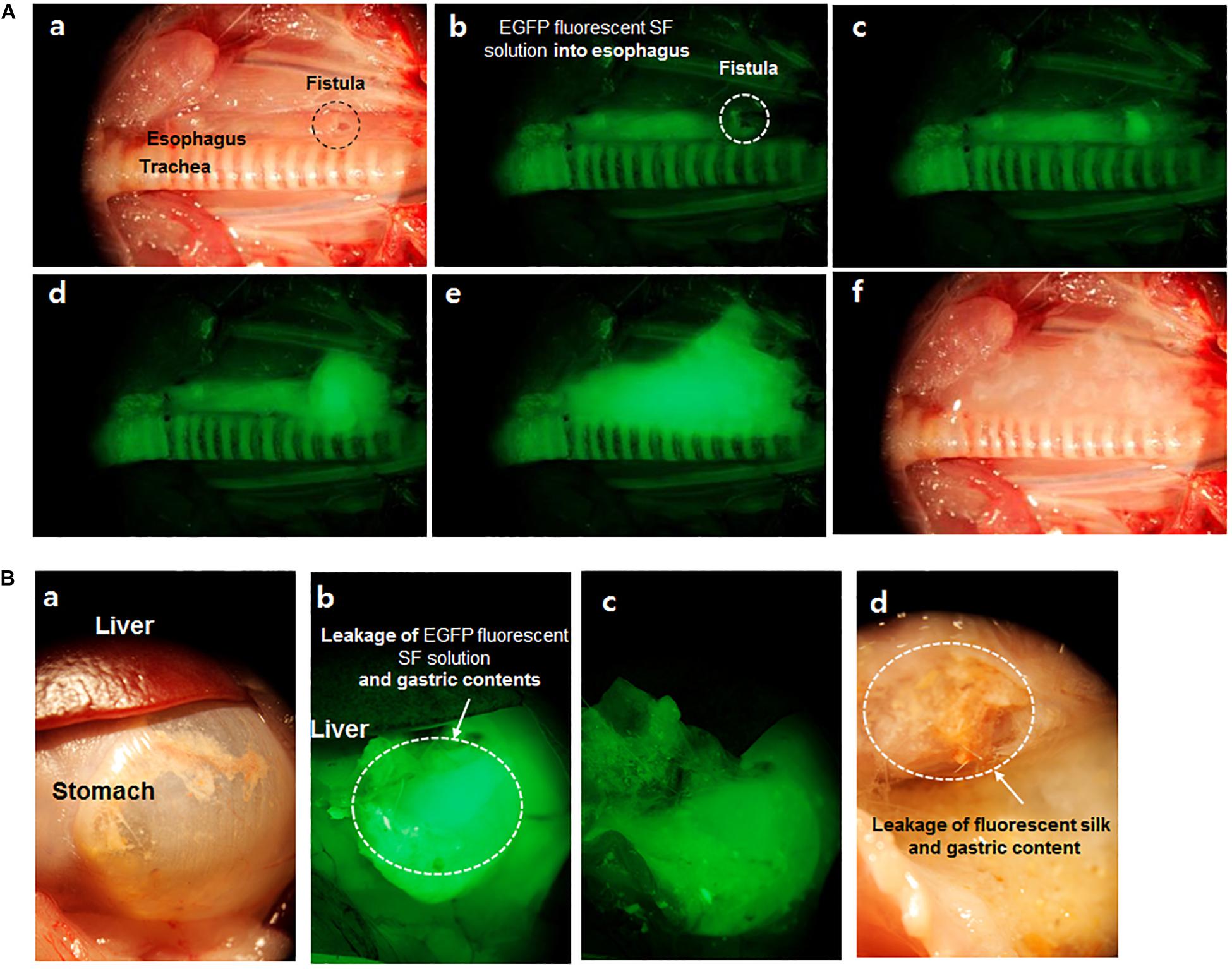
Figure 6. Images of EGFP fluorescent SF solution in esophageal (A) and stomach (B) fistula of the rat. (a) Esophageal fistula (0.5 mm) image with white light reflectance, (b–e) EGFP fluorescent captured images per 0.3 s. EGFP fluorescent was detected at esophageal fistula according to time passed under fluorescent light, (f) Image of leaked EGFP fluorescent SF from fistula with white light reflectance. (B,a) Stomach and liver image with white light reflectance light (b,c) Leakage image of EGFP fluorescent SF solution and gastric contents from fistula site after feeding of EGFP fluorescent SF solution under fluorescent light, (d) Leakage image of EGFP fluorescent SF solution and gastric contents with white light reflectance light. Reproduced from Kim et al. (2015) with permission from ELSEVIER.
Sensing Materials
Biosensing devices with excellent biocompatibility, sensitivity, multi-stimuli-responsivity, and low weight having great attention since health monitoring and early diagnosis are more critical than the treatment of diseases. Silk-derived materials have been applied as the support material to create pressure sensors with very high sensitivity. SF can be fabricated into numerous forms of hydrogels, ultra-fine, and thick films (Parker et al., 2009) and also can form flexible interfaces with biological recognition elements such as enzymes, and aptamers while retaining its functional properties for sensing applications (Pal et al., 2016). SF protein has been used as a sensor material for the detection of vitamin B12 by Chakravarty et al. Their SF-based sensing platform was developed for the dual-mode tracking of B12 in human blood serum (HBS) and solution using photoluminescence (PL) and electrical methods to enhances the strength and operative lifespan of the device. The autofluorescence of SF solution exhibited a high fluorescence quenching, while a micro-patterned film of SF showed a sharp increase in current intensity in the presence of B12 (Chakravarty et al., 2018).
In a previous study, electrospun fluorescent silk nanofibers (FSNs) have been applied as a very sensitive chemosensor and a nutrient releaser with a physically transient form. Among these, the sodium fluorescein doped FSN could be used as an extremely sensitive fluorescent chemosensor for acid fumes in the air. In this study, FSN sensors reacted to the acid vapor of the hydrochloric (HCl) instantly at a lethal concentration of > 300 ppm and could also be detected at low concentrations of ∼5 ppm. FSNs could be disappeared at a set period by regulating the silk fibers’ crystallinity. Depend on this property, FSNs mat was used as an indicator or water-disposable skin-type patch to be attached to protective equipment and clothing. The reduction of the fluorescence of nanofiber mats was defined to measure the quantity of vitamin B2 transported to the skin (Min et al., 2017). Complete control of fluorescence release by introducing mesoscopic ordered structures and its applications will be significantly extended the usefulness of fluorescence in several fields, including optical systems, optoelectronic technology, and biological sensors. Furthermore, considering that SF-based sensors emerged only in recent years, innovative sensors with highly sensitive, flexible, durable, biocompatible, and portable can be predicted in the future.
Drug Delivery
Silk is well known as a suitable carrier for the delivery of different drugs due to its excellent biocompatibility, non-toxicity, high binding capacity, controlled drug release properties, and relatively slow degradability in vivo (Hofmann et al., 2006; Murphy and Kaplan, 2009). Fluorescent dyes have been applied to monitor and visualize the internalization of carriers or measure the loading and release of drugs in controlled release/delivery systems (Wang et al., 2007; Ren et al., 2011). Khalid et al. have been demonstrated the drug release kinetics of the nanodiamond-SF-doxorubicins (NDSX) spheres by loading spheres with anthracycline DoX. Degradable SF spheres stabilize and release the drug in a precise manner while ND provides a fluorescent modality for imaging. Degradability of SF and the following release of DoX was monitored through the fluorescence of ND inside the spheres. These NDSX spheres have the potential to a role as dual-functional nanomaterials for drug delivery and succeeding imaging within biological structures and could be useful in targeted drug delivery (Khalid et al., 2016).
A versatile FSN has been prepared using a novel combination of optically active organic dyes and silk polymer. FSNs produced through the electrospinning approach shows striking functions of the fixed organic dyes along with encoding the system that physically disappears at a preset time. Fluorescent nanofibrous mats with red-green-blue (RGB) color, disposable membranes for nutrition delivery, and ecofriendly and transient fluorescent chemosensors for acid vapor recognition, were adequately demonstrated using FSNs. A drug delivery model using membranes of silk nanofiber fixed with vitamin B2 was also recommended (Min et al., 2017).
Antibacterial Effect
SF with novel properties can be fabricated by the immobilization of nanomaterials to SF materials. Silver nanoparticles (AgNPs) applied as antimicrobial agents are effective against approximately 650 strains of bacteria, including antibiotic-resistant strains, have gained enormous interest in the functionalization of silk. Recently, antibacterial SF scaffolds with green synthesized AgNPs have been developed for human mesenchymal stem cell differentiation and osteoblast proliferation. The antimicrobial activity of AgNPs immobilized-SF films was evaluated against gram-negative bacteria and antibiotic-resistant bacteria, and it was found to be effective against both (Nadiger and Shukla, 2016). Fluorescent proteins often result in cytotoxicity and phototoxicity due to the production and release of reactive oxygen species (ROS) by some red fluorescent proteins. The photo-generation of ROS is actively used for removing cancerous tissue. It has been demonstrated that silk with a transgenic fusion of far-red fluorescent probe in (mKate2) offers a photosensitizer hybridization platform for photo inducible control of ROS. ROS are active radicals for breaking down organic contaminants that were generated when the resulting hybrid was illuminated with a green light. The survival rate of E. coli dropped to 45 percent when E. coli on the fluorescent silk was activated for 60 min under a weak green light (Figure 7; Leem et al., 2018).
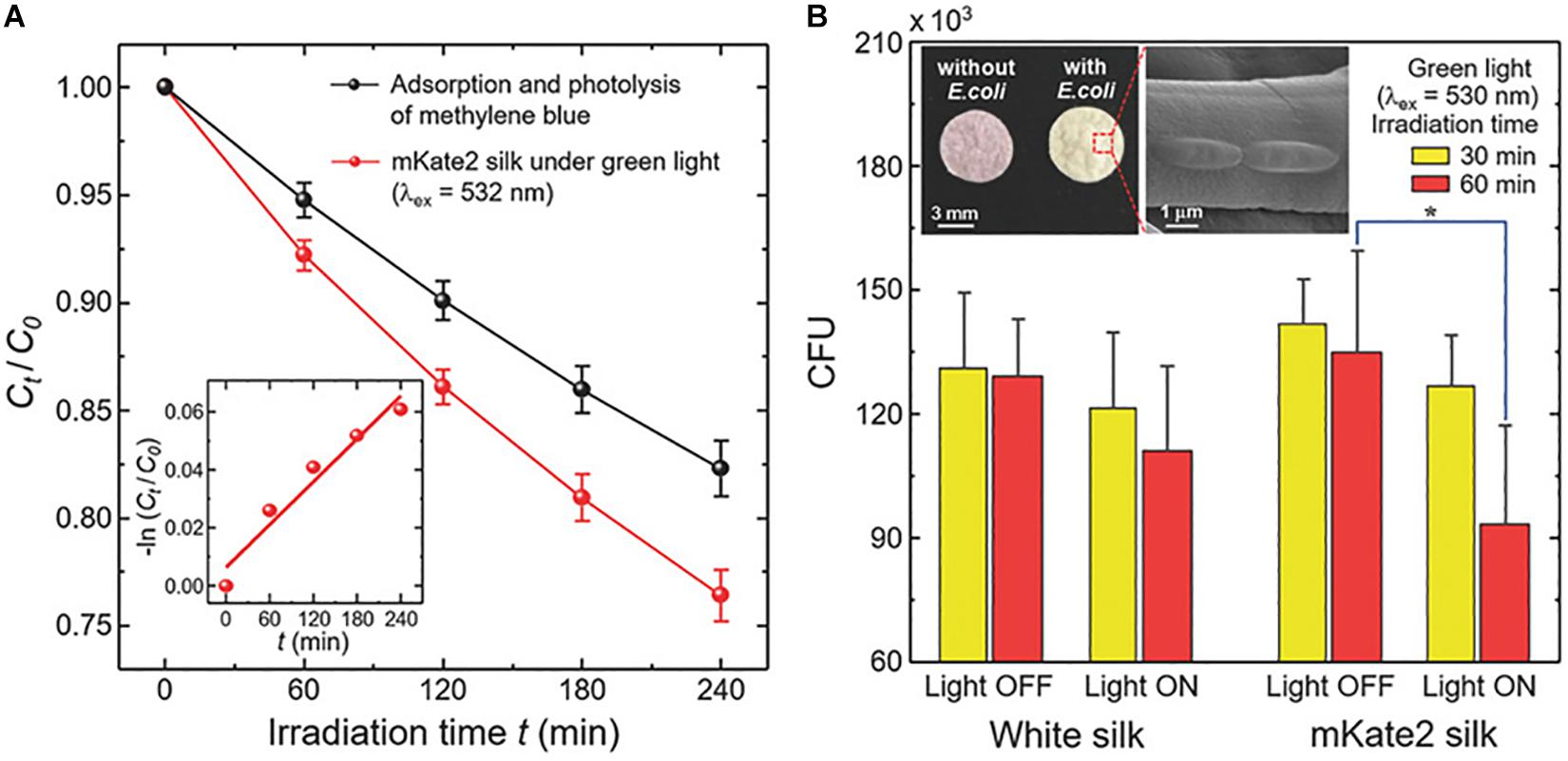
Figure 7. Photocatalytic activity of mKate2 silk for degrading methylene blue and deactivating bacteria under green light excitation at ambient temperature. (A) Photodegradation of methylene blue in aqueous solutions by mKate2 silk under green light activation. (B) Colony-forming units (CFU) of live E. coli (DH5α). Reproduced from Leem et al. (2018) with permission from WILEY-VCH.
Optical Systems
SF in film form has been applied for numerous high-performance optical devices (Omenetto and Kaplan, 2008; Parker et al., 2009; Ling et al., 2016). Based on several vital physical properties, biocompatible SF has potential as an optical biomaterial (Perry et al., 2008). The optical properties of SF films can be adjusted by changing the coating rate and varying their response to humidity (Li et al., 2017), which would make SF as a suitable humidity indicator. SF has been utilized to develop an ultrathin active moiré chiral metamaterials (MCMs) as a novel optical sensor in which SF use as a spacer layer (Wu et al., 2018). However, there is no study about the application of fluorescence SF in the optical systems. Therefore, further research needed to be performed to explore the applicability of fluorescent SF in the optical system.
Conclusion
Silkworm silks have been applied as a luxurious textile material for over 4000 years. Typically, raw silkworm silk comprises two kinds of self-assembled proteins, fibroin and sericin. The fibroin is a significant constituent of silk fiber serving as the core, while the sericin is a minor constituent serving as a coating protein. SF, which has undergone the degumming for removal of sericin, provides an essential set of material options for biomaterials such as coatings for cell culture and drug delivery matrices and scaffolds for tissue engineering, including bone, cartilage, skin, tympanic membrane, vasculature, and the esophagus because of the impressive mechanical properties, biocompatibility, and biodegradability. SF can be transformed into various forms, including films, hydrogels, nanoparticles, nanofibers for various biomedical applications. Transformed SF into these different forms depends on formulating a solution form of SF as a precursor. Several properties of fluorescent SF are similar to those of natural SF. Iizuka et al. (2013) revealed that the mechanical properties of the fluorescent SF regards to the maximum strain tolerated and Young’s modulus was similar to those of ordinary silk. However, the maximum stress value of the natural silk was slightly higher than that of the fluorescent silk (Iizuka et al., 2013). Super strong fluorescent silk, which was produced through feeding Bombyx mori silkworms with carbon nanodots, showed super-strong mechanical properties than that of the natural silk (Chu and Liu, 2008). In our previous study, we exhibited similar cell viability on both the fluorescent SF membrane and wild type SF membrane (Kim et al., 2015). This finding indicated that the fluorescent properties did not alter the biocompatibility of the fluorescent SF. Photostability is one of the fundamental properties of the fluorescent SF for functional silk fiber for fabricating fabrics and for biomedical applications. An early study showed that the fluorescent color of the fabrics with the recombinant silk persisted for over 2 years. Therefore, if fluorescent SF can be produced in various forms, it can also be used in various biomedical fields. The multifunctional SF-based materials with fluorescent can use to in vitro and in vivo imaging purposes and other biomedical applications due to their excellent fluorescent properties and biocompatibility. Aside from the biomedical applications, the derivatives of fluorescence SF, such as films, gels, fibers offer the possibility in electronics and photonics. In the future, we believe fluorescent SF will be created by the fusion of various advanced technology and will further expand the biomedical applications.
Author Contributions
OL and MS wrote the manuscript. HH, YL, JL, HL, and SK contributed to collect references. CP designed and supervised to organize the manuscript. All authors carefully read the manuscript and commented on the article.
Funding
This work was supported by the National Research Foundation of South Korea (NRF) grant funded by the Korea Government (MSIP; Grant Nos. NRF-2015R1D1A3A01020100 and NRF-2018R1D1A1B07050927), Republic of Korea and by the Hallym University Research Fund.
Conflict of Interest
The authors declare that the research was conducted in the absence of any commercial or financial relationships that could be construed as a potential conflict of interest.
References
Altman, G. H., Diaz, F., Jakuba, C., Calabro, T., Horan, R. L., Chen, J., et al. (2003). Silk-based biomaterials. Biomaterials 24, 401–416.
Amirikia, M., Shariatzadeh, S. M. A., Jorsaraei, S. G. A., and Mehranjani, M. S. (2018). Auto-fluorescence of a silk fibroin-based scaffold and its interference with fluorophores in labeled cells. Eur. Biophys. J. 47, 573–581. doi: 10.1007/s00249-018-1279-1
Arai, T., Freddi, G., Innocenti, R., Kaplan, D. L., and Tsukada, M. (2001a). Acylation of silk and wool with acid anhydrides and preparation of water-repellent fibers. J. Appl. Polym. Sci. 82, 2832–2841. doi: 10.1002/app.2137
Arai, T., Ishikawa, H., Freddi, G., Winkler, S., and Tsukada, M. (2001b). Chemical modification of Bombyx mori silk using isocyanates. J. Appl. Polym. Sci. 79, 1756–1763. doi: 10.1002/1097-4628(20010307)79:10<1756::aid-app30>3.0.co;2-g
Arai, T., Freddi, G., Innocenti, R., and Tsukada, M. (2003). Preparation of water-repellent silks by a reaction with octadecenylsuccinic anhydride. J. Appl. Polym. Sci. 89, 324–332. doi: 10.1002/app.12081
Bergonzo, P., Patel, P., Boyd, I. W., and Kogelschatz, U. (1992). Development of a novel large area excimer lamp for direct photo deposition of thin films. Appl. Surf. Sci. 54, 424–429. doi: 10.1016/0169-4332(92)90081-8
Bhardwaj, N., Devi, D., and Mandal, B. B. (2015). Tissue-engineered cartilage: the crossroads of biomaterials, cells and stimulating factors. Macromol. Biosci. 15, 153–182. doi: 10.1002/mabi.201400335
Cai, Z., Jiang, G., and Yang, S. (2001). Chemical finishing of silk fabric. Color. Technol. 117, 161–165. doi: 10.1111/j.1478-4408.2001.tb00056.x
Campbell, F. L. (1932). Preliminary experiments on the toxicity of certain coal-tar dyes for the silkworm. J. Econ. Entomol. 25, 905–917. doi: 10.1093/jee/25.4.905
Chakravarty, S., Gogoi, B., Mandal, B. B., Bhardwaj, N., and Sarma, N. S. (2018). Silk fibroin as a platform for dual sensing of vitamin B12 using photoluminescence and electrical techniques. Biosens. Bioelectron 112, 18–22. doi: 10.1016/j.bios.2018.03.057
Chu, M., and Liu, G. (2008). Fluorescent silkworm silk prepared via incorporation of green, yellow, red, and near-infrared fluorescent quantum dots. IEEE Trans. Nanotechnol. 7, 308–315. doi: 10.1109/TNANO.2008.917836
Daimon, T., Hirayama, C., Kanai, M., Ruike, Y., Meng, Y., Kosegawa, E., et al. (2010). The silkworm Green b locus encodes a quercetin 5-O-glucosyltransferase that produces green cocoons with UV-shielding properties. Proc. Natl. Acad. Sci. U.S.A. 107, 11471–11476. doi: 10.1073/pnas.1000479107
Davarpanah, S., Mahmoodi, N. M., Arami, M., Bahrami, H., and Mazaheri, F. (2009). Environmentally friendly surface modification of silk fiber: chitosan grafting and dyeing. Appl. Surf. Sci. 255, 4171–4176. doi: 10.1016/j.apsusc.2008.11.001
Font Tellado, S., Bonani, W., Balmayor, E. R., Foehr, P., Motta, A., Migliaresi, C., et al. (2017). (∗) Fabrication and characterization of biphasic silk fibroin scaffolds for tendon/ligament-to-bone tissue engineering. Tissue Eng. Part A 23, 859–872. doi: 10.1089/ten.TEA.2016.0460
Georgakoudi, I., Tsai, I., Greiner, C., Wong, C., Defelice, J., and Kaplan, D. (2007). Intrinsic fluorescence changes associated with the conformational state of silk fibroin in biomaterial matrices. Opt. Express 15, 1043–1053. doi: 10.1364/oe.15.001043
Harizuka, M. (1953). Physiological genetics of the carotenoids in Bombyx mori, with special reference to the pink cocoon. Bull. Seric Exp. Stn. Jpn. 14, 141–156.
He, G. S., Tan, L. S., Zheng, Q., and Prasad, P. N. (2008). Multiphoton absorbing materials: molecular designs, characterizations, and applications. Chem. Rev. 108, 1245–1330. doi: 10.1021/cr050054x
Heslot, H. (1998). Artificial fibrous proteins: a review. Biochimie 80, 19–31. doi: 10.1016/s0300-9084(98)80053-9
Hino, R., Tomita, M., and Yoshizato, K. (2006). The generation of germline transgenic silkworms for the production of biologically active recombinant fusion proteins of fibroin and human basic fibroblast growth factor. Biomaterials 27, 5715–5724. doi: 10.1016/j.biomaterials.2006.07.028
Hodgkinson, T., Yuan, X. F., and Bayat, A. (2014). Electrospun silk fibroin fiber diameter influences in vitro dermal fibroblast behavior and promotes healing of ex vivo wound models. J. Tissue Eng. 5, 2041731414551661. doi: 10.1177/2041731414551661
Hofmann, S., Foo, C. T., Rossetti, F., Textor, M., Vunjak-Novakovic, G., Kaplan, D. L., et al. (2006). Silk fibroin as an organic polymer for controlled drug delivery. J. Control Release 111, 219–227. doi: 10.1016/j.jconrel.2005.12.009
Iizuka, T., Sezutsu, H., Tatematsu, K. I., Kobayashi, I., Yonemura, N., Uchino, K., et al. (2013). Colored fluorescent silk made by transgenic silkworms. Adv. Funct. Mater. 23, 5232–5239. doi: 10.1002/adfm.201300365
Jucci, N., and Ponsevernoi (1930). Migration in to cocoon and eggs of dyes administered to silkworms. Boll. Soc. Italiana Biol. Speriment. 5, 1056–1060.
Kaneko, M., Iwahata, S., and Asakura, T. (1991). Luminescence from excited tris(2,2’-bipyridine)-ruthenium(II) incorporated into a silk fibroin membrane. J. Photochem. Photobiol. A Chem. 61, 373–380. doi: 10.1016/1010-6030(91)90020-T
Khalid, A., Lodin, R., Domachuk, P., Tao, H., Moreau, J. E., Kaplan, D. L., et al. (2014). Synthesis and characterization of biocompatible nanodiamond-silk hybrid material. Biomed. Opt. Express 5, 596–608. doi: 10.1364/BOE.5.000596
Khalid, A., Mitropoulos, A. N., Marelli, B., Tomljenovic-Hanic, S., and Omenetto, F. G. (2016). Doxorubicin loaded nanodiamond-silk spheres for fluorescence tracking and controlled drug release. Biomed. Opt. Express 7, 132–147. doi: 10.1364/BOE.7.000132
Kikuchi, Y., Mori, K., Suzuki, S., Yamaguchi, K., and Mizuno, S. (1992). Structure of the Bombyx mori fibroin light-chain-encoding gene: upstream sequence elements common to the light and heavy chain. Gene 110, 151–158. doi: 10.1016/0378-1119(92)90642-3
Kim, D. W., Lee, O. J., Kim, S. W., Ki, C. S., Chao, J. R., Yoo, H., et al. (2015). Novel fabrication of fluorescent silk utilized in biotechnological and medical applications. Biomaterials 70, 48–56. doi: 10.1016/j.biomaterials.2015.08.025
Kim, J., Kim, C. H., Park, C. H., Seo, J. N., Kweon, H., Kang, S. W., et al. (2010). Comparison of methods for the repair of acute tympanic membrane perforations: silk patch vs. paper patch. Wound Repair Regen. 18, 132–138. doi: 10.1111/j.1524-475X.2009.00565.x
Kim, S. W., Yun, E. Y., Choi, K.-H., Kim, S. R., Park, S. W., Kang, S. W., et al. (2012). Construction of fluorescent red silk using fibroin H-chain expression system. J. Seric Entomol. Sci. 50:8792.
Kim, S. W., Yun, E. Y., Choi, K.-H., Kim, S. R., Park, S. W., Kang, S. W., et al. (2013). Production of fluorescent green silk using fibroin H-chain expression system. J. Seric Entomol. Sci. 51, 153–158. doi: 10.7852/jses.2013.51.2.153
Kim, S. W., Yun, E. Y., Choi, K.-H., Kim, S. R., Park, S. W., Kang, S. W., et al. (2014). Expression of the blue fluorescent protein in fibroin H-chain of transgenic silkworm. J. Seric Entomol. Sci. 52, 25–32. doi: 10.7852/jses.2014.52.1.25
Ko, N. R., Nafiujjaman, M., Cherukula, K., Lee, S. J., Hong, S.-J., Lim, H.-N., et al. (2018). Microwave-assisted synthesis of biocompatible silk fibroin-based carbon quantum dots. Part. Part. Syst. Charact. 35:1700300. doi: 10.1002/ppsc.201700300
Kundu, S. C., Kundu, B., Talukdar, S., Bano, S., Nayak, S., Kundu, J., et al. (2012). Invited review nonmulberry silk biopolymers. Biopolymers 97, 455–467. doi: 10.1002/bip.22024
Kusurkar, T. S., Tandon, I., Sethy, N. K., Bhargava, K., Sarkar, S., Singh, S. K., et al. (2013). Fluorescent silk cocoon creating fluorescent diatom using a “Water glass-fluorophore ferry”. Sci. Rep. 3:3290. doi: 10.1038/srep03290
Lee, J. M., Sultan, M. T., Kim, S. H., Kumar, V., Yeon, Y. K., Lee, O. J., et al. (2017). Artificial auricular cartilage using silk fibroin and polyvinyl alcohol hydrogel. Int. J. Mol. Sci. 18:E1707. doi: 10.3390/ijms18081707
Lee, M. S., Lee, M., Tokuyama, T., Wakida, T., Inoue, G., and Ishida, S. (2006). Ammonia-gas and liquid ammonia treatments of silk fabric. J. Appl. Polym. Sci. 101, 3487–3492. doi: 10.1002/app.24520
Lee, O. J., Ju, H. W., Kim, J. H., Lee, J. M., Ki, C. S., Kim, J. H., et al. (2014). Development of artificial dermis using 3D electrospun silk fibroin nanofiber matrix. J. Biomed. Nanotechnol. 10, 1294–1303. doi: 10.1166/jbn.2014.1818
Leem, J. W., Park, J., Kim, S. W., Kim, S. R., Choi, S. H., Choi, K. H., et al. (2018). Green-light-activated photoreaction via genetic hybridization of far-red fluorescent protein and silk. Adv. Sci. (Weinh) 5:1700863. doi: 10.1002/advs.201700863
Li, G., Liu, H., Li, T., and Wang, J. (2012). Surface modification and functionalization of silk fibroin fibers/fabric toward high performance applications. Mater. Sci. Eng. C 32, 627–636. doi: 10.1016/j.msec.2011.12.013
Li, Q., Qi, N., Peng, Y., Zhang, Y., Shi, L., Zhang, X., et al. (2017). Sub-micron silk fibroin film with high humidity sensibility through color changing. RSC Adv. 7, 17889–17897. doi: 10.1039/C6RA28460D
Li, S., and Jinjin, D. (2007). Improvement of hydrophobic properties of silk and cotton by hexafluoropropene plasma treatment. Appl. Surf. Sci. 253, 5051–5055. doi: 10.1016/j.apsusc.2006.11.027
Lin, N., Meng, Z., Toh, G. W., Zhen, Y., Diao, Y., Xu, H., et al. (2015). Engineering of fluorescent emission of silk fibroin composite materials by material assembly. Small 11, 1205–1214. doi: 10.1002/smll.201402079
Ling, S., Li, C., Jin, K., Kaplan, D. L., and Buehler, M. J. (2016). Liquid exfoliated natural silk nanofibrils: applications in optical and electrical devices. Adv. Mater. 28, 7783–7790. doi: 10.1002/adma.201601783
Liu, J. M., David, W. C., Ip, D. T., Li, X. H., Li, G. L., Wu, X. F., et al. (2009). High-level expression of orange fluorescent protein in the silkworm larvae by the Bac-to-Bac system. Mol. Biol. Rep. 36, 329–335. doi: 10.1007/s11033-007-9183-2
Lombardi, P. L. (1920). The duration of the stay of the ingested leaf in the intestine of the silkworm. Ann. Scuola Agr. Portici 2, 1–10.
Maclaren, J. A. (1981). Wool Science: The Chemical Reactivity of the Wool Fibre/John A. Maclaren and Brian Milligan. Marrickville, NSW: Science Press.
Mandal, B. B., and Kundu, S. C. (2009). Cell proliferation and migration in silk fibroin 3D scaffolds. Biomaterials 30, 2956–2965. doi: 10.1016/j.biomaterials.2009.02.006
Marsh, R. E., Corey, R. B., and Pauling, L. (1955). An investigation of the structure of silk fibroin. Biochim. Biophys. Acta 16, 1–34.
Min, K., Kim, S., Kim, C. G., and Kim, S. (2017). Colored and fluorescent nanofibrous silk as a physically transient chemosensor and vitamin deliverer. Sci. Rep. 7:5448.
Murphy, A. R., and Kaplan, D. L. (2009). Biomedical applications of chemically-modified silk fibroin. J. Mater. Chem. 19, 6443–6450. doi: 10.1039/b905802h
Myung, S. J., Kim, H.-S., Kim, Y., Chen, P., and Jin, H.-J. (2008). Fluorescent silk fibroin nanoparticles prepared using a reverse microemulsion. Macromol. Res. 16, 604–608. doi: 10.1007/bf03218567
Nadiger, V. G., and Shukla, S. R. (2016). Antibacterial properties of silk fabric treated with silver nanoparticles. J. Text. Inst. 107, 1543–1553. doi: 10.1080/00405000.2015.1129756
Omenetto, F. G., and Kaplan, D. L. (2008). A new route for silk. Nat. Photon. 2:641. doi: 10.1038/nphoton.2008.207
Otterburn, M. S. (1977). “The chemistry and reactivity of silk,” in Chemistry of Natural Protein Fibers, ed. R. S. Asquith (Boston, MA: Springer US), 53–80. doi: 10.1007/978-1-4613-4109-3_2
Pal, R. K., Farghaly, A. A., Wang, C., Collinson, M. M., Kundu, S. C., and Yadavalli, V. K. (2016). Conducting polymer-silk biocomposites for flexible and biodegradable electrochemical sensors. Biosens. Bioelectron. 81, 294–302. doi: 10.1016/j.bios.2016.03.010
Park, B. J., Takatori, K., Sugita-Konishi, Y., Kim, I.-H., Lee, M.-H., Han, D.-W., et al. (2007). Degradation of mycotoxins using microwave-induced argon plasma at atmospheric pressure. Surf. Coat. Technol. 201, 5733–5737. doi: 10.1016/j.surfcoat.2006.07.092
Park, Y. R., Sultan, M. T., Park, H. J., Lee, J. M., Ju, H. W., Lee, O. J., et al. (2018). NF-kappaB signaling is key in the wound healing processes of silk fibroin. Acta Biomater. 67, 183–195. doi: 10.1016/j.actbio.2017.12.006
Parker, S. T., Domachuk, P., Amsden, J., Bressner, J., Lewis, J. A., Kaplan, D. L., et al. (2009). Biocompatible silk printed optical waveguides. Adv. Mater. 21, 2411–2415. doi: 10.1002/adma.200801580
Periyasamy, S., Gulrajani, M. L., and Gupta, D. (2007a). Preparation of a multifunctional mulberry silk fabric having hydrophobic and hydrophilic surfaces using VUV excimer lamp. Surf. Coat. Technol. 201, 7286–7291. doi: 10.1016/j.surfcoat.2007.01.038
Periyasamy, S., Gupta, D., and Gulrajani, M. L. (2007b). Modification of one side of mulberry silk fabric by monochromatic VUV excimer lamp. Eur. Polym. J. 43, 4573–4581. doi: 10.1016/j.eurpolymj.2007.07.014
Perry, H., Gopinath, A., Kaplan, D. L., Dal Negro, L., and Omenetto, F. G. (2008). Nano- and micropatterning of optically transparent, mechanically robust, biocompatible silk fibroin films. Adv. Mater. 20, 3070–3072. doi: 10.1002/adma.200800011
Ren, D., Kratz, F., and Wang, S. W. (2011). Protein nanocapsules containing doxorubicin as a pH-responsive delivery system. Small 7, 1051–1060. doi: 10.1002/smll.201002242
Rockwood, D. N., Preda, R. C., Yucel, T., Wang, X., Lovett, M. L., and Kaplan, D. L. (2011). Materials fabrication from Bombyx mori silk fibroin. Nat. Protoc. 6, 1612–1631. doi: 10.1038/nprot.2011.379
Sakudoh, T., Sezutsu, H., Nakashima, T., Kobayashi, I., Fujimoto, H., Uchino, K., et al. (2007). Carotenoid silk coloration is controlled by a carotenoid-binding protein, a product of the Yellow blood gene. Proc. Natl. Acad. Sci. U.S.A. 104, 8941–8946. doi: 10.1073/pnas.0702860104
Sargunamani, D., and Selvakumar, N. (2006). A study on the effects of ozone treatment on the properties of raw and degummed mulberry silk fabrics. Polym. Degrad. Stabil. 91, 2644–2653. doi: 10.1016/j.polymdegradstab.2006.05.001
Sultan, M. T., Lee, O. J., Kim, S. H., Ju, H. W., and Park, C. H. (2018). Silk fibroin in wound healing process. Adv. Exp. Med. Biol. 1077, 115–126. doi: 10.1007/978-981-13-0947-2_7
Sultan, M. T., Moon, B. M., Yang, J. W., Lee, O. J., Kim, S. H., Lee, J. S., et al. (2019). Recirculating peritoneal dialysis system using urease-fixed silk fibroin membrane filter with spherical carbonaceous adsorbent. Mater. Sci. Eng. C Mater. Biol. Appl. 97, 55–66. doi: 10.1016/j.msec.2018.12.021
Tamura, T., Thibert, C., Royer, C., Kanda, T., Abraham, E., Kamba, M., et al. (2000). Germline transformation of the silkworm Bombyx mori L. using a piggyBac transposon-derived vector. Nat. Biotechnol. 18, 81–84. doi: 10.1038/71978
Tamura, Y., Nakajima, K., Nagayasu, K., and Takabayashi, C. (2002). Flavonoid 5-glucosides from the cocoon shell of the silkworm, Bombyx mori. Phytochemistry 59, 275–278. doi: 10.1016/s0031-9422(01)00477-0
Tang, L., Yang, Y., Li, Y., Yang, G., Luo, T., Xu, Y., et al. (2018). Knitted silk mesh-like scaffold incorporated with sponge-like regenerated silk fibroin/collagen I and seeded with mesenchymal stem cells for repairing Achilles tendon in rabbits. Acta Bioeng. Biomech. 20, 77–87.
Tansil, N. C., Li, Y., Koh, L. D., Peng, T. C., Win, K. Y., Liu, X. Y., et al. (2011a). The use of molecular fluorescent markers to monitor absorption and distribution of xenobiotics in a silkworm model. Biomaterials 32, 9576–9583. doi: 10.1016/j.biomaterials.2011.08.081
Tansil, N. C., Li, Y., Teng, C. P., Zhang, S., Win, K. Y., Chen, X., et al. (2011b). Intrinsically colored and luminescent silk. Adv. Mater. 23, 1463–1466. doi: 10.1002/adma.201003860
Teule, F., Miao, Y. G., Sohn, B. H., Kim, Y. S., Hull, J. J., Fraser, M. J., et al. (2012). Silkworms transformed with chimeric silkworm/spider silk genes spin composite silk fibers with improved mechanical properties. Proc. Natl. Acad. Sci. U.S.A. 109, 923–928. doi: 10.1073/pnas.1109420109
Tomita, M. (2011). Transgenic silkworms that weave recombinant proteins into silk cocoons. Biotechnol. Lett. 33, 645–654. doi: 10.1007/s10529-010-0498-z
Tomita, M., Munetsuna, H., Sato, T., Adachi, T., Hino, R., Hayashi, M., et al. (2003). Transgenic silkworms produce recombinant human type III procollagen in cocoons. Nat. Biotechnol. 21, 52–56. doi: 10.1038/nbt771
Uchino, K., Imamura, M., Shimizu, K., Kanda, T., and Tamura, T. (2007). Germ line transformation of the silkworm, Bombyx mori, using the transposable element Minos. Mol. Genet. Genomics 277, 213–220. doi: 10.1007/s00438-006-0176-y
Vepari, C., and Kaplan, D. L. (2007). Silk as a biomaterial. Prog. Polym. Sci. 32, 991–1007. doi: 10.1016/j.progpolymsci.2007.05.013
Wang, X., Hu, X., Daley, A., Rabotyagova, O., Cebe, P., and Kaplan, D. L. (2007). Nanolayer biomaterial coatings of silk fibroin for controlled release. J. Control Release 121, 190–199. doi: 10.1016/j.jconrel.2007.06.006
Win, K. Y., Teng, C. P., Ye, E., Low, M., and Han, M. Y. (2015). Evaluation of polymeric nanoparticle formulations by effective imaging and quantitation of cellular uptake for controlled delivery of doxorubicin. Small 11, 1197–1204. doi: 10.1002/smll.201402073
Wu, Z., Chen, X., Wang, M., Dong, J., and Zheng, Y. (2018). High-performance ultrathin active chiral metamaterials. ACS Nano 12, 5030–5041. doi: 10.1021/acsnano.8b02566
Xu, Z., Shi, L., Yang, M., and Zhu, L. (2019). Preparation and biomedical applications of silk fibroin-nanoparticles composites with enhanced properties – A review. Mater. Sci. Eng. C Mater. Biol. Appl. 95, 302–311. doi: 10.1016/j.msec.2018.11.010
Yamao, M., Katayama, N., Nakazawa, H., Yamakawa, M., Hayashi, Y., Hara, S., et al. (1999). Gene targeting in the silkworm by use of a baculovirus. Genes Dev. 13, 511–516. doi: 10.1101/gad.13.5.511
Yanagisawa, S., Zhu, Z., Kobayashi, I., Uchino, K., Tamada, Y., Tamura, T., et al. (2007). Improving cell-adhesive properties of recombinant Bombyx mori silk by incorporation of collagen or fibronectin derived peptides produced by transgenic silkworms. Biomacromolecules 8, 3487–3492. doi: 10.1021/bm700646f
Yang, Y., Shao, Z., Chen, X., and Zhou, P. (2004). Optical spectroscopy to investigate the structure of regenerated Bombyx mori silk fibroin in solution. Biomacromolecules 5, 773–779. doi: 10.1021/bm0343848
Yeon, Y. K., Park, H. S., Lee, J. M., Lee, J. S., Lee, Y. J., Sultan, M. T., et al. (2018). New concept of 3D printed bone clip (polylactic acid/hydroxyapatite/silk composite) for internal fixation of bone fractures. J. Biomater. Sci. Polym. Ed. 29, 894–906. doi: 10.1080/09205063.2017.1384199
Ying Zhang, J., Esrom, H., Kogelschatz, U., and Emig, G. (1993). Large area photochemical dry etching of polyimide with excimer UV lamps. Appl. Surf. Sci. 69, 299–304. doi: 10.1016/0169-4332(93)90522-D
Zhang, F., Zhao, Y., Chen, X., Xu, A., Huang, J., and Lu, C. (1999). Fluorescent transgenic silkworm. Acta Biochim. Biophys. Sin. 31, 119–123.
Zhang, Y., Fan, W., Ma, Z., Wu, C., Fang, W., Liu, G., et al. (2010). The effects of pore architecture in silk fibroin scaffolds on the growth and differentiation of mesenchymal stem cells expressing BMP7. Acta Biomater. 6, 3021–3028. doi: 10.1016/j.actbio.2010.02.030
Zhao, Y., Chen, X., Peng, W. P., Dong, L., Huang, J. T., and Lu, C. D. (2001). Altering fibroin heavy chain gene of silkworm Bombyx mori by homologous recombination. Sheng Wu Hua Xue Yu Sheng Wu Wu Li Xue Bao (Shanghai) 33, 112–116.
Zheng, Z. Z., Liu, M., Guo, S. Z., Wu, J. B., Lu, D. S., Li, G., et al. (2015). Incorporation of quantum dots in silk biomaterials for fluorescence imaging. J. Mater. Chem. B 3, 6509–6519. doi: 10.1039/C5TB00326A
Keywords: fluorescent, silk, bio-imaging, sensing materials, medical application
Citation: Lee OJ, Sultan MT, Hong H, Lee YJ, Lee JS, Lee H, Kim SH and Park CH (2020) Recent Advances in Fluorescent Silk Fibroin. Front. Mater. 7:50. doi: 10.3389/fmats.2020.00050
Received: 07 December 2019; Accepted: 18 February 2020;
Published: 20 March 2020.
Edited by:
Antonella Motta, University of Trento, ItalyReviewed by:
Keiji Numata, RIKEN, JapanVamsi Yadavalli, Virginia Commonwealth University, United States
Copyright © 2020 Lee, Sultan, Hong, Lee, Lee, Lee, Kim and Park. This is an open-access article distributed under the terms of the Creative Commons Attribution License (CC BY). The use, distribution or reproduction in other forums is permitted, provided the original author(s) and the copyright owner(s) are credited and that the original publication in this journal is cited, in accordance with accepted academic practice. No use, distribution or reproduction is permitted which does not comply with these terms.
*Correspondence: Chan Hum Park, hlpch@paran.com
†These authors have contributed equally to this work