- 1PCM2E, EA 6299 Université de Tours, Parc de Grandmont, Tours, France
- 2The Department of Materials Science and Nano-engineering, Mohammed VI Polytechnic University, Benguerir, Morocco
Lithium titanate (Li4Ti5O12, LTO) has emerged as an alternative anode material for rechargeable lithium ion (Li+) batteries with the potential for long cycle life, superior safety, better low-temperature performance, and higher power density compared to their graphite-based counterparts. LTO, being a “zero-strain” material, shows almost no volume change (<1%) during lithium ion insertion/extraction and hence offers excellent cycling stability (over 20,000 cycles). LTO anodes were popular initially on the belief that the anode electrolyte interface would be free of any solid electrolyte interphase (SEI) layer; however, this was found not to be the case. Rather, recent studies have reported different types of deposits and layer formations on the surface of LTO electrodes, and therefore this topic has received significant attention in recent years and has emerged as an important research direction. However, these anodes, being very active catalysts, are prone to undesirable reactions with electrolytes and problems such as gas evolution, and associated swelling of the battery pack may occur. Also, the electrolytes have been found to be one of the primary sources of problems, given that the electrolytes may react with both the anode and cathode, creating serious stability and safety concerns. The presence of moisture within the battery system, decomposition of the electrolyte solvents and solutes, and high catalytic activity of the anode are among the possible reasons behind the instability of LTO-based batteries. Development of an appropriate chemical composition for the electrolyte and/or modification of the electrode/electrolyte interface may overcome these issues.
Introduction
The importance of lithium ion (Li+) batteries (LIBs) has been established for several decades; however, efforts are ongoing to refine and improve the performance of the batteries. A high energy density and a high power density are required to cater for the diverse applications, ranging from miniaturized electronics, home appliances, to light and heavy electric vehicles (Arico et al., 2005; Armand and Tarascon, 2008; Kang and Ceder, 2009). The energy density of LIBs depends directly on the potential differences between the lithium ion intercalation potentials of the positive (cathode) and the negative (anode) electrodes (Park et al., 2008). In this context, graphite has traditionally been the preferred anode material for commercial use. The main benefit of graphite is its very low intercalation potential (~0.1 V vs. Li+/Li), which helps to obtain a high operating voltage and hence a high energy density (Sawai et al., 1994). However, this feature comes at the cost of several safety and stability issues. Such low intercalation potentials result in a much higher possibility of dendrite formation. The occurrence of dendrites eventually leads to short-circuiting of the battery, and this is a serious safety concern (Barai et al., 2017; Jana and García, 2017). At such a low potential, the electrolyte is reduced and a solid electrolyte interphase (SEI) layer is formed (Aurbach, 2002), which in one sense is beneficial as it stops further reaction of the anode with the electrolyte, but the layer also hinders lithium ion insertion/removal, leading to a poor cycle life and irreversible capacity during the first few cycles (Winter et al., 1998). In addition, graphite is prone to changes in volume due to the Li+ intercalation/deintercalation processes. This volume change compromises the electrode's mechanical stability, and due to this, electrode materials shred from the current collector, and this eventually compromises the battery life cycle (Jansen et al., 1999; Scrosati and Garche, 2010). At this juncture, the use of lithium titanate (Li4Ti5O12, LTO) as an anode turned out to be an excellent alternative to the graphite anode. The LTO anode operates at a much higher voltage (~1.55 V vs. Li+/Li) and hence provides superior safety features by eliminating the production of any type of dendrite formation. Moreover, the LTO anode has been demonstrated to have excellent cyclic stability thanks to its negligible volume change during intercalation, hence the good structural stability. In addition, the LTO has been shown to have a higher working temperature stability and higher charging rate capability in comparison to the graphite anode (Hernandez et al., 1996; Doh et al., 2002; Park et al., 2002; Yao et al., 2005). However, the LTO is highly active and because of its catalytic activity, the electrode can participate in many different types of reactions with electrolytes and create instability and safety concerns within the cell. Therefore, physical and chemical characterization of the electrode/electrolyte interface is of critical importance in terms of gaining an improved understanding of the stability and safety issues which affect LTO-based batteries. In this mini review, we will focus specifically on this interface and discuss the associated stability of LTO-based batteries.
The Chemistry of Lithium Titanate Anodes and the Mechanism of Operation
LTO is a spinel oxide, having a crystal structure with an Fd3m space group and cubic symmetry (Ohzuku, 1995; Sorensen et al., 2006). In this structure, each unit cell contains eight formula units of (Li)8a(Li1/3Ti5/3)16d. All 8a sites and 1/6 of the 16d octahedral sites are occupied by lithium ions. The rest of the octahedral 16d sites are occupied by tetravalent ions, and the ratio of lithium ions to titanium ions is 1:5. The oxygen ions are located at the 32e sites (Wang et al., 1999; Aldon et al., 2004). The octahedral 16c sites and the tetrahedral 8b and 48f sites are empty, and these sites can participate in Li+ insertion–extraction processes (Jovic et al., 2003; Shu, 2009). One formula unit of Li4Ti5O12, after accepting three Li+ insertions within the structure, gets converted into the rock-salt structure with the chemical formula Li7Ti5O12. These three Li+ insertions result in a theoretical capacity of 175 mAh/g. This electrochemical conversion from spinel-LTO to rock-salt-LTO is a two-phase process whereby a solid solution is formed between these two phases, and the phase transition corresponds to the voltage plateau at 1.55 V vs. Li+/Li. There is very little difference in the lattice parameters between the two phases, that is, 8.3595 Å for spinel-LTO and 8.3538 Å for rock-salt-LTO (Kubiak et al., 2003); therefore, the phase change results in an almost negligible volume change, which is 0.2% (Chen et al., 2013). In contrast, Li+ insertion within graphite gives a volume change of about ~10–13% (Schweidler et al., 2018). Therefore, LTO is known as a “zero-strain” material for Li+ insertion (Sun et al., 2015). It is worth mentioning that [TiO6] functions as the mainframe for the Li+ insertions/extractions, and the lithium ions, which had preoccupied the 8a sites (before the insertion process), move out and then occupy the 16c sites during the insertion process; at the same time, the three newly inserted lithium ions also fill-in other available 16c sites via the 8a sites. A mutual repulsive force is established between the newly inserted ions and the preexisting ions at the 8a sites. This force knocks off the 8a sites' ions and moves them out to the 16c sites. The extraction process happens exactly in the reverse direction; that is, three lithium ions from the 16c sites leave the crystal, and other ions move back to the 8a sites (Shu, 2009). It is also of interest to note that during the phase transition, three Ti4+ ions (spinel-LTO) are reduced to Ti3+ (rock-salt-LTO) ions (Ohzuku, 1995; Zaghib et al., 1999; Shu, 2009; Yi et al., 2010). The corresponding electrochemical reaction is as follows:
This Ti4+/Ti3+ redox couple gives the steady-state plateau at 1.55 V vs. Li+/Li, and therefore at this voltage, the LTO can accept three inserted lithium ions, and this is the plateau that LTO batteries utilize in their applications. However, it has been shown that if the discharge voltage is extended to 0V, then based on the reduction of all Ti4+ ions, the theoretical capacity of 293–296 mAhg−1 can be obtained (Ge et al., 2009; Shu, 2009; Hsieh and Lin, 2010). This extension in discharge voltage provides an opportunity to improve the energy density of LTO batteries; however, the enhancement has been attributed to the higher surface area derived from the nano-sized particles, or from the epitaxial thin film's specific planner orientations (Borghols et al., 2009; Cunha et al., 2019). However, in both situations, the higher surface area provides a higher risk of irreversible capacity, as indicated in a previous report (Borghols et al., 2009), and high irreversible capacity is undesirable for full cell configurations. Therefore, the standard potential was kept at the lower voltage of 1.55 V vs. Li+/Li. LTO anodes may be coupled to different cathodes, such as LiCoO2 (LCO), LiNiO2 (LNO), LiMn2O4 (LMO), or LiFePO4 (LFP) to construct lithium ion batteries.
Electrolyte and Electrode Interface of Lithium Titanate Batteries: Solid Electrolyte Interphase/Solid Electrolyte Interphase-Like Layers
Electrode and electrolyte interfaces have special importance for LTO anodes, as they behave differently compared with the interfaces for graphite anodes. For graphite interfaces, well-formed SEI layers are observed, but for LTO-based anodes, this is not always seen. LTO-based anodes have a charge–discharge plateau at 1.55 V vs. Li+/Li, and that value is higher than the reduction potential for most electrolytes. As a result, LTO anodes, unlike graphite, do not form stable SEI layers. Therefore, initially, it was assumed wrongly that the LTO anode is a completely SEI-free material. Later, however, many observations were made that suggested the existence/formation of SEI layers or SEI-like layers. These SEI layers are very important for LTO battery safety and stability, given that LTO electrodes are highly catalytic in nature. LTO anodes participate in several types of reactions with the electrolyte of the cell and produce many different types of composites and various associated gases. The bare surface of the LTO is a major source of gassing phenomena in LTO batteries (Ganapathy and Wagemaker, 2012; Lu et al., 2012). The outermost surface (~3 nm thick) of the LTO is highly reactive (He et al., 2012a), having active surface-terminating ions, such as Li+, O2−, and Ti4+ on the (111) crystal plane of the LTO anode; these ions have been proposed as catalysts for gaseous CO2 production. Other sources proposed include electronic holes on the oxygen-rich LTO surface; these holes can lead to electron exchange processes with electrolytes to generate CO2 (Kitta et al., 2014). Surface energy states of LTO anodes can control reactions such as dehydrogenation and decarbonylation (Qin et al., 2011; He et al., 2012a) and can generate H2 and CO. Thus, the LTO surface itself behaves as a catalyst for gas generation processes and produces many different gases; the associated detailed mechanisms for gas formation are described elsewhere (Qin et al., 2011; Ganapathy and Wagemaker, 2012; He et al., 2012a; Wu et al., 2013a; Guo et al., 2015; Lu et al., 2015; Tikhonov and Lin, 2015).
Clearly, it is very important to protect the electrolyte from this highly reactive LTO surface. As indicated above, however, the mechanical stabilities of these SEI-like layers are very different from each other, especially given that various cell configurations and different operating conditions including different temperatures, charging rates, etc., are used. It is worth noting that some of the layers consist of deposits, which form stable SEI-like layers. Occasionally, the layers have been found to consist of loosely bound material (Kitta et al., 2012), which was deposited on the anode surface. In some occasions, the deposits were not generated from the anode interfaces, but rather from the layers' constituent particles/components which were found to have migrated from the sides of the cathode. For example, in a series of studies, Dedryvère et al. (2010) showed that the SEI layers formed had two components, namely, an organic part and an inorganic part. The inorganic component (“LiF”) originated from the decomposition of LiPF6 and was formed on the top of the LTO-based anode surface. However, the organic counterpart, which was produced due to the oxidation of the organic solvents, originated from the sides of the cathode (Dedryvere et al., 2005; Dedryvère et al., 2010; El Ouatani et al., 2009). Similar observations were made when LTO anodes were cycled against two different cathodes with LTO/LMO and LTO/nickel manganese cobalt oxide (NMC) cell configurations. In both situations, the inorganic components of the SEI were observed on the top surface of the LTO and the organic components were present deep within the SEI. The bulk compositions of both SEI layers were similar, but the respective morphologies were dependent on the cathodes. For the LTO/LMO configuration, the layer was thicker and contained small amounts of manganese compounds which had originated from the positive electrode since the first cycle. The manganese was produced as a result of dissolution of the LMO positive electrode with subsequent migration of the manganese ions through the electrolyte to the negative electrode during the cycling. In addition, the presence of titanium (from dissolution of the LTO electrode) at a low level was detected on the surface of the positive electrode. Even if this layer at the surface of the positive electrode had only a minimal impact on the electrochemical performance of the cell, this was another example of interaction between the electrodes, that is, from the positive electrode to the negative electrode and vice versa (Gauthier et al., 2020a). Electrode interactions (crosstalk) were found to be responsible for a greater degree of parasitic reactions at the LTO electrode, relative to the LiNi0.5Mn1.5O4 (LNMO) electrode within an LTO/LNMO full cell. A greater amount of reactions resulted in lower coulombic efficiencies, which was again increased in an elevated temperature atmosphere (Aktekin et al., 2018). The interaction between the anode and the cathode was further confirmed in another study where the various upper cutoff voltages were used during constant current cycling. Below 4.3 V (potential of NMC electrode vs. Li+/Li), good capacity retention and low impedance were observed. However, at a higher cathode potential, serious capacity loss and a critical increase of electrochemical impedance were observed. These changes in performance occurred due to electrolyte degradation at the NMC cathode. The study indicated that the electrolyte (LiF and fluorophosphate) and solvent (–CO containing species) degradation products, and the NMC dissolution products, such as manganese or nickel species, were formed at the NMC electrodes which then migrated to the anode surface (Gauthier et al., 2020b). It was also observed that the formation of an SEI layer was dependent on the particle morphology and the discharge profile (He et al., 2012a, 2013). On some occasions, it has been observed that, even though the compositions of the formed SEI layers can be similar, the same SEI layer may affect battery performance to differing extents; in one scenario, an improvement in performance was observed, whereas in another situation, performance degradation became an issue (Belharouak et al., 2012). However, all these observations clearly indicate the presence of some type of surface layer and demonstrate that the assumption of an “SEI-free anode” was not correct. Given this situation, in-depth studies on the surface chemistry of LTO anodes have been performed in recent years, and interesting observations have been made. It can be stated categorically that the formation of SEI layers in the case of LTO anodes follows different mechanisms to that for graphite anodes, and both the anode and cathode contribute to the formation pathways.
It is worth mentioning that these SEI-like layers play very important roles within an LTO anode-based system. The SEI-like layers can be the key to protecting the anode, which would otherwise be prone to reacting with the different components of the electrolytes to generate gases. Such gases tend to create many safety issues for LTO batteries. It has been observed that in the first stage, gas generation processes resulted in a sharp increase in the internal pressure of an LTO/NMC battery pouch, resulting in detaching of the internal components of the battery. The active interface between the anode and the electrolyte got isolated by the gas layers. Such loss in direct contact severely affected the migration of lithium ions. In the second stage of gas generation, when internal pressure (pressure inside the pouch cell) equaled external pressures (pressure outside the pouch cell), a constant rate gas generation was observed. In the third stage, an SEI film was slowly formed on the surface of the LTO during the gassing process, which eventually reduced the direct contact of LTO with the electrolyte, and lowered the interaction between LTO and the electrolyte, and thus the gassing rate (Liu et al., 2017; Wang et al., 2019). It was also observed that the gassing mechanism was different in different stages of gas generations, resulting in different gas compositions at different stages (Liu et al., 2017; Wang et al., 2019). It was also observed in the gas generation process that the relative amount of the component gases was different during different stages of the cycled time, when the LTO/NMC pouch was cycled and gas compositions were considered for a time window of 75–553 h (Figure 1A) (Wang et al., 2019). In another work in a similar observation, it was found that LTO electrode initiated the side reactions to produce gases, and the deposits formed SEI film, which slowly covered the electrode/electrolyte interface. The film suppressed the direct contact in the interface, pushing the internal pressure to gently increase during the following cycles. The SEI layer eventually became denser and covered the interface completely, suppressing side reactions and stabilizing internal pressure (Wang Q. et al., 2017). It was also possible to distinguish between the irreversible pressure changes within a pouch cell due to gas formation and the reversible pressure changes caused by structural changes of the electrodes, namely, the deposition and dissolution of lithium (Schiele et al., 2017). It has been consistently noticed that at the elevated temperatures, gas generations have been accelerated and hence more instabilities can be observed at the elevated temperatures compared to the room temperatures (He et al., 2015; Lv et al., 2017; Xu et al., 2017; Wang et al., 2019).
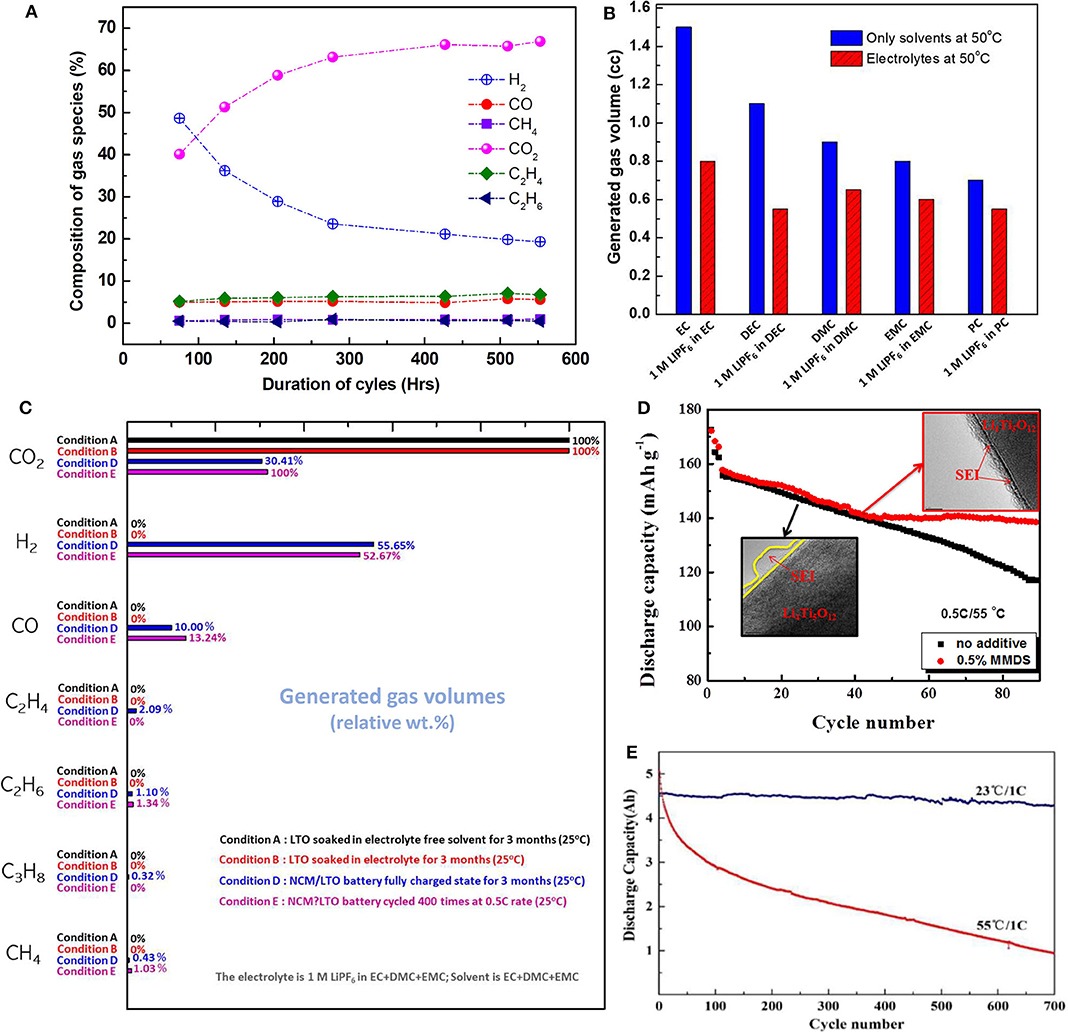
Figure 1. (A) Lithium tantanate (LTO)/nickel manganese cobalt oxide (NMC) pouch cell, the relative amount of the component gases during different stages of the cycled time. (A) is plotted from the data of He et al. (2012a), Wang et al. (2019). (B) Total emitted gas volumes from an NCM/LTO battery when LTO is soaked under conditions with only solvents (blue) and within the electrolytes (red) for 3 months. (C) Relative amount of gas emissions from an NCM/LTO cell under conditions (A,B,D,E) are plotted from the data of He et al. (2012a). (D) Comparison of capacity retentions and associated images of formed solid electrolyte interface (SEI) layers for the two systems; with and without additives. Reproduced from Wang R. et al. (2015). (E) Effect of temperature on cycling of an LTO electrode; samples were cycled at 23°C and an elevated 55°C. Reproduced from Wang et al. (2019).
Therefore, it is very important to understand the role of the electrolyte and the associated instabilities at the interfaces. Further, depending on the nature of the interaction between the electrode and the electrolyte, it is sometimes beneficial to construct artificially an SEI-like layer to promote assisted formation of a protective layer on the anode surface. These types of externally assisted artificial layers can be deposited/coated in different ways. Also, various surface modifications of the anode can induce the formation of stable SEI layers (Charles-Blin et al., 2019). It was observed that, for the purpose of suppressing the gas generation of LMO//LTO/C LIB systems, a high-potential formation protocol was developed, which resulted in a stable SEI film on the surfaces of LTO/C materials. A formation potential above 3.0–3.2 V was applied, and a larger portion of the flexible polyethylene oxide (PEO) component was formed in the interface layer, resulting in less gas generation and improved cycling performance (Wen et al., 2019). Further, there are several options for modifying the composition of the electrolytes with chemical additives to realize stable SEI layers. These procedures are discussed in the next section.
Electrolyte Composition and Stability of Lithium Titanate Batteries
The stability and safety of a battery system are largely dependent upon the composition of the electrolyte, and LTO batteries are no exception. The most common electrolyte used in LTO batteries is LiPF6 dissolved in various alkyl carbonates such as ethylene carbonate (EC), propylene carbonate (PC), dimethyl carbonate (DMC), diethyl carbonate (DEC), and ethyl-methyl carbonate (EMC) (Goodenough and Kim, 2010; Shkrob et al., 2013; Kalhoff et al., 2015). Here, both the solute and the solvent play key roles in influencing the stability of the electrolyte. In the case of LiPF6, the solute can dissociate in accord with the following equation: LiPF6 ⇋ PF5 + LiF. The equation has a high value for the equilibrium constant with PF5 being produced in the system. PF5 is highly reactive with organic solvents and, in addition, can react with different SEI components to produce gases (Sloop et al., 2001, 2003; Okamoto, 2013; Wilken et al., 2013; Wang R. et al., 2015). Also, alkyl carbonates including their decomposition products decompose faster in the presence of LiPF6 (Belharouak et al., 2012). PF5 has been reported to react with the SEI-like layer of the LTO anode and release CO2 gas (Wang R. et al., 2015).
The solvent perhaps plays an even bigger role in terms of the stability and safety of the LTO batteries. For example, a cell with DMC as solvent has shown larger swelling for a pouch cell battery compared to the situation when PC has been added to it. Also, the nature of the evolved gas is influenced highly by the solvent in use and on the relative amounts of the solvents present in a mixture. For example, when the mix ratio of PC–DMC and PC–DEC changed from 1:1 to 1:4, C3H6 evolution was observed instead of H2 due to the different solvent activities (Wu et al., 2013b). The type of solvent can also control the rate of gas evolution, as well as the total volume of gas evolution from a cell. EC and PC behaved very differently when they were mixed with EMC, DMC, and DEC. For instance, EC yielded a larger gas volume relative to that for PC. The mixture EC–DMC exhibited the highest gas volume, and PC–DMC showed the lowest gas volume, as a result of the high quality of the protective layers (Wu et al., 2013b). It was also observed that more gas was evolved when the cell was stored at a higher state of the charge, and the corresponding cell also demonstrated that the presence of PC had less gassing than cells stored in the presence of EC, which can be attributed to the fact that PC-based electrolyte formed a thicker and denser surface film on LTO compared to the EC-based electrolytes (Liu et al., 2015). In an LTO/LCO system, a similar observation was made. There, FEC-based electrolyte exhibited more generated gas than EC- and PC-based electrolytes, with PC-based electrolytes producing a minimum amount of gas (Li et al., 2017). In addition, the types of solvents control the nature of the emitted gases. Solvents with cyclic carbonate components, such as PC, emit alkene gases, while linear carbonates such as DMC emit H2, CH4, and other alkyl gases (Wu et al., 2013b). For example, in the case of EC-based cells, the dominant gases are CO2 and CO. Compositions of emitted gas mixtures change with change in the solvents such as for PC-based cells where the dominant gases are CO together with smaller amounts of CO2 and C3H8/C3H6. Figure 1, which is based on data reported elsewhere (He et al., 2012a), depicts such behavior for an LTO battery. For the same anode and cathode couple comprising LTO and Li(Ni1/3Co1/3Mn1/3)O2 (NCM), respectively, the amount of total emitted gas varied widely depending upon the nature of the solvent used. Also, it was observed that the amount of emitted gas varied when only solvent and electrolyte (solvent with the salt) were used. Figure 1B shows how battery chemistry and conditions can influence the nature and amount of different gas species (He et al., 2012a).
Sulfone-based electrolytes find application with more stable LTO anodes for high-energy battery applications which require the highest levels of safety. Sulfone-based solvents are electrochemically more stable at higher voltages, being less prone to oxidation at higher voltages compared to that of carbonate solvents and can provide better high-voltage stability for cathodes (Demeaux et al., 2013). In an LTO/LNMO cell, the flammability and electrochemical performance of a tetramethyl sulfone (TMS)-based electrolyte was tested. The exceptional electrochemical stability of the sulfone electrolytes and their compatibility with the LTO is the main reason behind the outstanding electrochemical performance observed with high-voltage spinel cathode materials (Abouimrane et al., 2009). Electrolytes such as lithium bis[(trifluoromethyl)sulfonyl]imide (LiTFSI) in glutaronitrile (GLN) or in 2-methylglutaronitrile (MGLN) were investigated for use in the Li4Ti5O12/LiNi1/3Co1/3Mn1/3O2 (LTO/NMC) battery. These electrolytes provided alkyl carbonate and LiPF6-free LTO batteries, as well as larger operational temperatures (Farhat et al., 2019).
The water content within the system is a very critical factor regarding the stability of the LTO battery. Trace amounts of water can be present within both the electrodes and the electrolyte. This water can be detrimental in high-voltage window aprotic electrolytes (free water can be highly reactive to LiPF6 salt and lithium alkyl carbonates) (Kawamura et al., 2006). Water can cause the hydrolysis of the LiPF6 salt present in the electrolyte and, thereafter, can decompose the electrolyte solvents (Aurbach, 2000; Verma et al., 2010). Synthesis of nanostructured LTO-based electrode materials, for example, the Li2O–TiO2 composition line, often requires water-based synthesis routes. Processes such as hydrothermal and sol-gel are used and, therefore, there can be some reactive intermediate composites, like Li2O–TiO2-H2O (lithium titanate hydrates or LTH), which can have water trapped within their structure. This trapped water can be categorized as two different groups, the loosely bound group which includes crystallographic water and adsorbed water and the other group consisting of deeply trapped water inside LTHs or pseudohydrates (i.e., hydroxide or hydroxonium ions or –OH and –H groups) (Franks et al., 1973; Cho et al., 2003). Recent research has clearly demonstrated that deeply trapped water is not problematic in terms of the stability of the LTO batteries, rather the work has demonstrated the advantages of such trapped water, which can promote a structural diversity of hydrated crystals (2D layered), and dehydration-induced phase transformation and nanostructure refinement (Wang S. et al., 2017).
However, loosely bound water and trace amounts of water present in the electrolyte are the main sources of the problems. The water itself can lead to water splitting reactions due to the higher working potential range of the LTO batteries (Belharouak et al., 2012; Bernhard et al., 2014). In addition, the LTO surface can work as a catalyst and promote water splitting, as observed for TiO2 which acted as a photocatalyst for water splitting in the production of H2 (Khan et al., 2002). It was found that the amount of gas generation was a direct function of the trace levels of water present within the electrolyte. In an experiment, where different LTO batteries with different controlled amounts of trace levels of water were annealed, a 99% increase in the cell volume was observed when the trace amounts of water were increased from 15 to 20,000 ppm (Wu et al., 2013b).
Assisted Formation of Solid Electrolyte Interphase Layers Including Modifications And/Or Coatings on the Surface of the Lithium Titanate
The gas evolution takes place mainly at the electrode/electrolyte interfaces of the LTO battery system. Therefore, the main function of a stable SEI or SEI-like layer on the LTO surface is to prevent or at least to reduce direct contact between the electrodes and the electrolytes. Coatings on the LTO surfaces can be a promising way to form a stable layer on the LTO surfaces which would prevent the electrode surface from the direct contact of the electrolyte. Coatings on the LTO anodes are preferably made of chemically inactive materials to avoid unwanted side reactions. These materials should be good conductors for Li+ transport and have high electron mobility, and these materials should provide enough protection to the electrode from the electrolyte, especially for operation at high temperatures.
Carbon materials are an excellent choice for coating purposes where the LTO surface is concerned (Jung et al., 2011; Zhao et al., 2011; Li et al., 2013; Sun et al., 2015). Carbon sources are not only chemically inactive but also good electrical conductors; in addition, the porosity of carbon can enhance Li+ transport significantly (Jung et al., 2011). It has been shown that LTO electrodes with carbon coatings can show resistance to gas formation and ensure higher stability for the battery. The electrolyte/electrode interface was coated with amorphous carbon, which successfully covered the catalytically active gassing sites on the LTO anodes. In this way, the anode surfaces, which were covered by the amorphous carbon, further suppressed the gassing phenomena, and hence provided an enhanced stability. It was reported that these amorphous coatings were stable and provided a stable SEI-protective layer (He et al., 2012a,b). As a result, the NCM111/(LTO/C) battery exhibited better cycling stability than the NCM111/LTO battery over 400 cycles (He et al., 2012a). Carbon coatings are beneficial due to their good conductivity, low-temperature performance, and good rate capabilities, and it is worth emphasizing that the thickness of the coating plays a key role in terms of controlling the performance of such electrodes (Yuan et al., 2010; Jung et al., 2011; Zhao et al., 2011; Li et al., 2013; Sun et al., 2015; Cheng et al., 2017).
Also, other ceramics and oxide materials may be used to coat the LTO surface for the purpose of suppression of gas generation (Han et al., 2015; Xiao et al., 2015; Charles-Blin et al., 2019). An SiO2-coated LTO has shown better Li+ diffusion and greater cyclic stability over 100 cycles. The improved electrochemical performance and stability were attributed to the coated layer on the surface of LTO, which prevented further decomposition of the electrolyte on the LTO surface, and thus stopped formation of unwanted solid deposits on the surface (Li et al., 2015). Similar observations were made when an ultrathin Al2O3 oxide layer was formed on the LTO surface, serving as a passivation film stabilizing LTO structure, and simultaneously suppressing unwanted chemical reactions (Ahn and Xiao, 2011). In another work, ZrO2 was coated on the LTO surface, which similarly suppressed the formation of unwanted interphase layers and improved electron transport through the ultrathin coating (Liu et al., 2013). Aluminum-doped zinc oxide (AZO) films were coated on the surface of LTO particles via atomic layer deposition, and the coated film with an optimal composition and film thickness exhibited higher capacity and better capacity retention, exhibiting a high capacity at 55°C after 250 cycles, which was 61% higher than that of uncoated LTO (Jin et al., 2019). A zinc oxide (ZnO; 2 wt.%) coating was tested, and it was shown to provide stability for 200 cycles, whereas in the absence of the coating, the stability lasted only 50 cycles (Han et al., 2015). A chemically formed TiO2 coating was used to obtain a high discharge capacity of 212 mAhg−1 at 10C rate while the bare-LTO delivers only 138 mAhg−1, along with ultra-high rate capability (150C) and a long cyclic stability of 1,000 cycles (with 80% retention). Moreover, the TiO2 coating was helpful in decreasing charge transfer resistance, and there was no phase transition observed on the surface even at an elevated temperature (Gangaja et al., 2019).
Other than coating, surface modifications and controlling other parameters were the ways to achieve stability of the LTO electrodes. To generate an SEI layer, fluorination of the LTO surface was performed using XeF2, which resulted in suppression of side reactions with the electrolyte. Moreover, the “Li-F” environment on the LTO surface did not alter the LTO structure, which retained its “zero-strain” characteristics (Charles-Blin et al., 2019). Variation of the temperature of the charge–discharge cycle is another way to control the quality and structure of the SEI layers. An LTO half-cell was cycled at different temperatures, namely, room temperature, 60°C, and 85°C, and it was observed that a thicker SEI layer was obtained at a higher temperature. It was also confirmed that the thicker electrode gradually passivated the LTO surface compared to the low-temperature cycled anodes (Gieu et al., 2016). A similar result was obtained for a full cell configuration (LTO/LMO system), where higher temperature cycling provided a thicker SEI layer; also, differences in the chemical composition and in-depth spatial distribution of the SEI layers were observed for different temperatures (Gauthier et al., 2020c). Table 1 (section A) summarizes some of the performance improvements after surface treatments/formation of coatings.
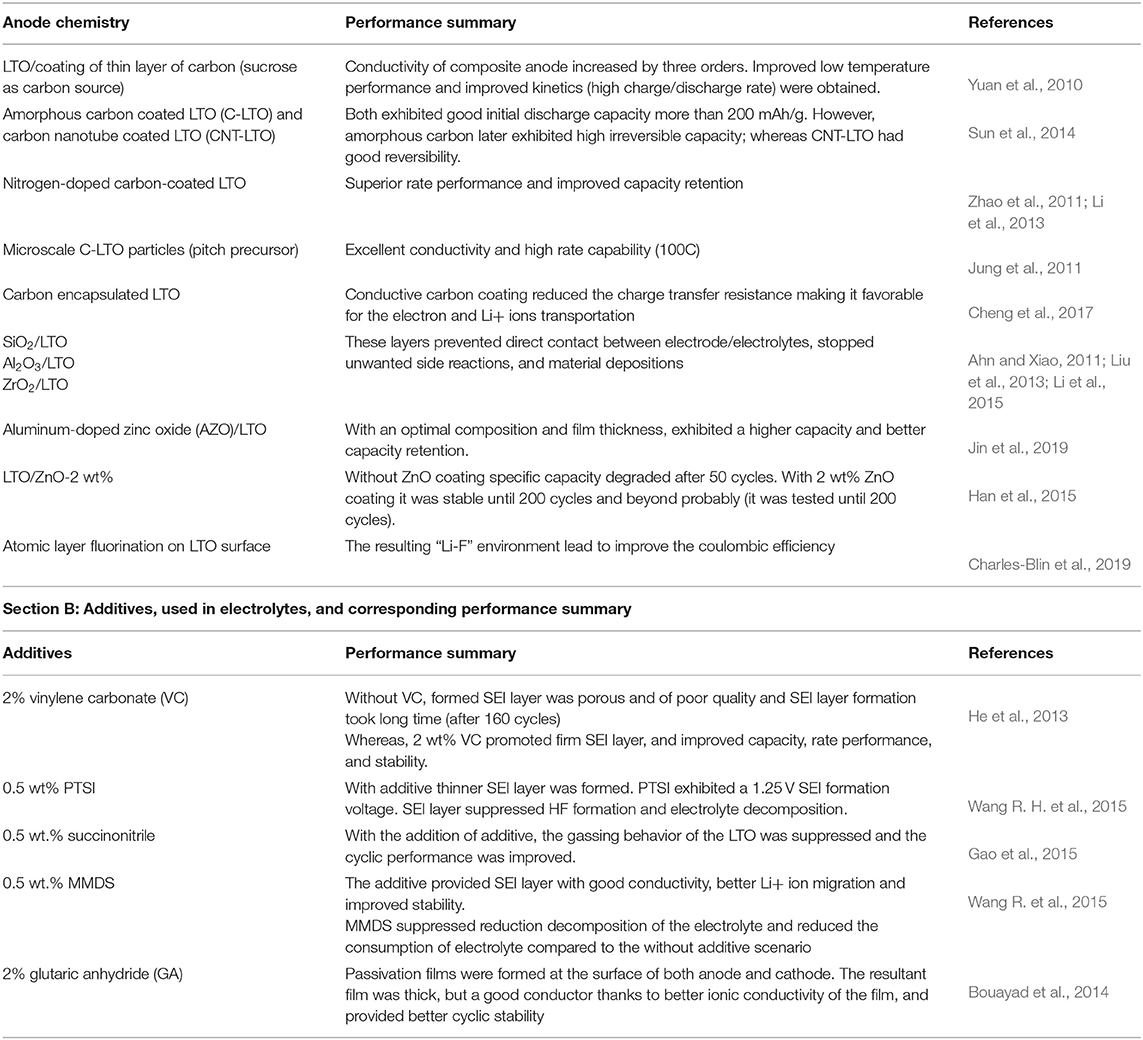
Table 1. Section A: modification and/or coating on the LTO anodes, and corresponding performance summary.
Additives in the Electrolyte
It has been observed that the addition of specific additives to the electrolyte has greatly influenced the performance of LTO batteries by facilitating the formation of stable, protective layers on the electrode surface, such layers being considered as a form of SEI. For instance, it was found that without additives, lithium acetate-based LTO (LA-LTO) initially did not form a stable SEI film until the 160th cycle (He et al., 2013). However, after the addition of 2% vinylene carbonate (VC) in the electrolyte composed of 1 M LiPF6 in EC/DMC/EMC, the SEI layer gained the required stability, and the total charge storage capacity was improved. However, it is of interest to note that lithium hydroxide-based LTO (LH-LTO) did not require any additive and exhibited excellent stability (He et al., 2013). The addition of VC increased the relative amount of organic species (at the expense of LiF) within the SEI layer formed on the surface of the LTO electrode compared to the scenario for the electrolyte without VC (Gieu et al., 2017). P-toluenesulfonyl isocyanate (PTSI) was used to form a thin and stable SEI layer, which improved the cycling performance of a Li/Li4Ti5O12 cell (with 0.5 wt.% PTSI) after 400 cycles (Wang R. H. et al., 2015). Succinonitrile (SN), an additive with a nitrile group, formed a barrier layer as a result of the reaction between Ti3+ on the LTO and the nitrile group (Gao et al., 2015). Methylene methanedisulfonate (MMDS) formed a thin SEI layer with good conductivity, and this provided improved stability and better Li+ diffusion (Okamoto, 2013). Schiff base [1,8-diazabicyclo[5.4.0]undec-7-ene (DBU)] additive was used to induce polymerization of the cyclic carbonates in the electrolyte, which resulted in the formation of an SEI layer on the surface of LTO, then leading to reduced gas formation. However, the addition of DBU also reduced cell capacity. Therefore, the concentration of the additive was reduced and a compromise between capacity retention and gas generation was achieved (Daigle et al., 2017). Therefore, addition of additives was not always a straightforward beneficial event. Such as, in an LTO/LMO system, when the additives lithium bisoxalato borate (LiBOB) and lithium difluorooxalato borate (LiDFOB) were incorporated, it resulted in the generation of a thicker surface film, which prevented H2 evolution and produced less H2. However, at the same time, an increase in CO2 evolution was observed in the system due to the incorporation of oxalato borates, and a net increase in gas evolution upon long-term cycling was observed. Whereas in the same system, incorporation of Tris(trimethylsilyl)borate (TMSB) resulted in a larger reduction in cell gassing, but that was accompanied by the lowest capacity retention (Milien et al., 2018). Therefore, an optimization of the performance and gas reduction turned out to be important. Other additives studied have included glutaric anhydride (GA) (Bouayad et al., 2014), phosphazene (Tan et al., 2012), chloro[2-[2-(2-methoxyethoxy)ethoxy]ethoxy] dimethylsilane (Chen et al., 2012), and chlorosilane (Qin et al., 2011), all of which have had a positive impact in suppressing gassing. Table 1 (section B) summarizes a few key observations and performance attributes for the additives used for obtaining stable SEI layers in LTO battery chemistry.
Outlook
LTO anodes provide the most promising alternative chemistries to graphite-based anodes. Even though in the above discussion we have highlighted several stability issues, most of the problems can be addressed by taking appropriate corrective measures. It was also found that suitable surface modifications or coatings on the LTO surfaces can prevent unwanted contact between the electrolytes and the electrodes, and this aspect can significantly improve battery stability and resolve safety issues. We also observed that modifications of the electrolyte chemistry via suitable additives are the key to forming stable SEI layers, which can prevent gassing phenomena and enhance the stability of the LTO anodes. Electrolytes, which may operate at very high potential, provide an option for operating the cathode at very high voltage, and this has the potential for improving the overall energy density of LTO-based batteries. Developing new additives is a promising future research direction for LTO batteries, and additives which reduce the flammability of solvents are the key to operating cathodes at a high voltage. Even though LTO batteries are discharged mainly at the 1.55 V vs. Li+/Li plateau, the discharge potential can be lowered to improve capacity. However, the lower discharge potential should be achieved by avoiding dendrite formation and improving the associated irreversibility. It is worth emphasizing that the absence of dendrite formation is one of the key advantages of LTO batteries. Nevertheless, the fast charging ability, safety, and very high cycle life of LTO batteries make them an ideal choice for electric vehicles (EVs, PHEV, etc.) and stationary energy storage applications. Also, the very high cycle life makes them an ideal candidate for second life battery applications.
Author Contributions
The authors listed have made a substantial, direct, and intellectual contribution to the work and approved it for publication.
Conflict of Interest
The authors declare that the research was conducted in the absence of any commercial or financial relationships that could be construed as a potential conflict of interest.
Acknowledgments
The authors would like to thank Le Studium Loire Valley Institute for Advanced Studies and Région Center Val de Loire for financial support to the researchers involved in this study.
References
Abouimrane, A., Belharouak, I., and Amine, K. (2009). Sulfone-based electrolytes for high-voltage Li-Ion batteries. Electrochem. Commun. 11, 1073–1076. doi: 10.1016/j.elecom.2009.03.020
Ahn, D., and Xiao, X. (2011). Extended lithium titanate cycling potential window with near zero capacity loss. Electrochem. Commun. 13, 796–799. doi: 10.1016/j.elecom.2011.05.005
Aktekin, B., Lacey, M. J., Nordh, T., Younesi, R., Tengstedt, C., Zipprich, W., et al. (2018). Understanding the capacity loss in LiNi 0.5 Mn 1.5 O 4 –Li 4 Ti 5 O 12 lithium-Ion cells at ambient and elevated temperatures. J. Phys. Chem. C 122, 11234–11248. doi: 10.1021/acs.jpcc.8b02204
Aldon, L., Kubiak, P., Womes, M., Jumas, J. C., Olivier-Fourcade, J., Tirado, J. L., et al. (2004). Chemical and electrochemical Li-insertion into the Li4Ti5O12 spinel. Chem. Mater. 16, 5721–5725. doi: 10.1021/cm0488837
Arico, A. S., Bruce, P., Scrosati, B., Tarascon, J. M., and van Schalkwijk, W. (2005). Nanostructured materials for advanced energy conversion and storage devices. Nat. Mater. 4, 366–377. doi: 10.1038/nmat1368
Armand, M., and Tarascon, J. M. (2008). Building better batteries. Nature 451, 652–657. doi: 10.1038/451652a
Aurbach, D. (2000). Review of selected electrode–solution interactions which determine the performance of Li and Li Ion batteries. J. Power Sources 89, 206–218. doi: 10.1016/S0378-7753(00)00431-6
Aurbach, D. (2002). A short review of failure mechanisms of lithium metal and lithiated graphite anodes in liquid electrolyte solutions. Solid State Ionics 148, 405–416. doi: 10.1016/S0167-2738(02)00080-2
Barai, P., Higa, K., and Srinivasan, V. (2017). Lithium dendrite growth mechanisms in polymer electrolytes and prevention strategies. Phys. Chem. Chem. Phys. 19, 20493–20505. doi: 10.1039/C7CP03304D
Belharouak, I., Koenig, G. M., Tan, T., Yumoto, H., Ota, N., and Amine, K. (2012). Performance degradation and gassing of Li4Ti5O12/LiMn2O4 lithium-Ion cells. J. Electrochem. Soc. 159, A1165–A1170. doi: 10.1149/2.013208jes
Bernhard, R., Meini, S., and Gasteiger, H. A. (2014). On-line electrochemical mass spectrometry investigations on the gassing behavior of Li4Ti5O12electrodes and its origins. J. Electrochem. Soc. 161, A497–A505. doi: 10.1149/2.013404jes
Borghols, W. J., Wagemaker, M., Lafont, U., Kelder, E. M., and Mulder, F. M. (2009). Size effects in the Li(4+x)Ti(5)O(12) spinel. J. Am. Chem. Soc. 131, 17786–17792. doi: 10.1021/ja902423e
Bouayad, H., Wang, Z., Dupré, N., Dedryvère, R., Foix, D., Franger, S., et al. (2014). Improvement of electrode/electrolyte interfaces in high-voltage spinel lithium-Ion batteries by using glutaric anhydride as electrolyte additive. J. Phys. Chem. C 118, 4634–4648. doi: 10.1021/jp5001573
Charles-Blin, Y., Flahaut, D., Ledeuil, J.-B., Guérin, K., Dubois, M., Deschamps, M., et al. (2019). Atomic layer fluorination of the Li4Ti5O12 surface: a multiprobing survey. ACS Appl. Energy Mater. 2, 6681–6692. doi: 10.1021/acsaem.9b01191
Chen, Z., Amine, K., and Belharouak, I. (2012). Surface Modification Agents for Lithium Batteries. Chicago, IL: US Patent 8187746 B2.
Chen, Z., Belharouak, I., Sun, Y. K., and Amine, K. (2013). Titanium-based anode materials for safe lithium-Ion batteries. Adv. Funct. Mater. 23, 959–969. doi: 10.1002/adfm.201200698
Cheng, Q., Tang, S., Liang, J., Zhao, J., Lan, Q., Liu, C., et al. (2017). High rate performance of the carbon encapsulated Li4Ti5O12 for lithium Ion battery. Results Phys. 7, 810–812. doi: 10.1016/j.rinp.2017.01.040
Cho, G., Wu, Y., and Ackerman, J. L. (2003). Detection of hydroxyl ions in bone mineral by solid-state NMR spectroscopy. Science 300, 1123–1127. doi: 10.1126/science.1078470
Cunha, D. M., Hendriks, T. A., Vasileiadis, A., Vos, C. M., Verhallen, T., Singh, D. P., et al. (2019). Doubling reversible capacities in epitaxial Li4Ti5O12 thin film anodes for microbatteries. ACS Appl. Energy Mater. 2, 3410–3418. doi: 10.1021/acsaem.9b00217
Daigle, J.-C., Asakawa, Y., Hovington, P., and Zaghib, K. (2017). Schiff base as additive for preventing gas evolution in Li 4 Ti 5 O 12 -based lithium-Ion battery. ACS Appl. Mater. Interfaces 9, 41371–41377. doi: 10.1021/acsami.7b15112
Dedryvère, R., Foix, D., Franger, S., Patoux, S., Daniel, L., and Gonbeau, D. (2010). Electrode/electrolyte interface reactivity in high-voltage spinel LiMn1.6Ni0.4O4/Li4Ti5O12 lithium-Ion battery. J. Phys. Chem. C 114, 10999–11008. doi: 10.1021/jp1026509
Dedryvere, R., Gireaud, L., Grugeon, S., Laruelle, S., Tarascon, J. M., and Gonbeau, D. (2005). Characterization of lithium alkyl carbonates by X-ray photoelectron spectroscopy: experimental and theoretical study. J. Phys. Chem. B 109, 15868–15875. doi: 10.1021/jp051626k
Demeaux, J., De Vito, E., Lemordant, D., Le Digabel, M., Galiano, H., Caillon-Caravanier, M., et al. (2013). On the limited performances of sulfone electrolytes towards the LiNi0.4Mn1.6O4 spinel. Phys. Chem. Chem. Phys. 15, 20900–20910. doi: 10.1039/c3cp53941e
Doh, C.-H., Jin, B.-S., Lim, J.-H., and Moon, S.-I. (2002). Electrochemical characteristics of lithium transition-metal oxide as an anode material in a lithium secondary battery. Korean J. Chem. Eng. 19, 749–755. doi: 10.1007/BF02706963
El Ouatani, L., Dedryvère, R., Siret, C., Biensan, P., and Gonbeau, D. (2009). Effect of vinylene carbonate additive in Li-Ion batteries: comparison of LiCoO2/C, LiFePO4/C, and LiCoO2/Li4Ti5O12 systems. J. Electrochem. Soc. 156, A468–A477. doi: 10.1149/1.3111891
Farhat, D., Lemordant, D., Jacquemin, J., and Ghamouss, F. (2019). Alternative electrolytes for Li-Ion batteries using glutaronitrile and 2-methylglutaronitrile with lithium bis(trifluoromethanesulfonyl) imide. J. Electrochem. Soc. 166, A3487–A3495. doi: 10.1149/2.1261914jes
Franks, F., Falk, M., and Knop, O. (1973). Water in Stoichiometric Hydrates. (Boston, MA: Springer), 55–113. doi: 10.1007/978-1-4757-6958-6_2
Ganapathy, S., and Wagemaker, M. (2012). Nanosize storage properties in spinel Li4Ti5O12 explained by anisotropic surface lithium insertion. ACS Nano 6, 8702–8712. doi: 10.1021/nn302278m
Gangaja, B., Nair, S., and Santhanagopalan, D. (2019). Surface-engineered Li4Ti5O12 nanoparticles by TiO2 coating for superior rate capability and electrochemical stability at elevated temperature. Appl. Surf. Sci. 480, 817–821. doi: 10.1016/j.apsusc.2019.03.061
Gao, J., Gong, B., Zhang, Q., Wang, G., Dai, Y., and Fan, W. (2015). Study of the surface reaction mechanism of Li4Ti5O12 anode for lithium-Ion cells. Ionics 21, 2409–2416. doi: 10.1007/s11581-015-1435-x
Gauthier, N., Courrèges, C., Demeaux, J., Tessier, C., and Martinez, H. (2020a). Probing the in-depth distribution of organic/inorganic molecular species within the SEI of LTO/NMC and LTO/LMO batteries: a complementary ToF-SIMS and XPS study. Appl. Surf. Sci. 501:144266. doi: 10.1016/j.apsusc.2019.144266
Gauthier, N., Courrèges, C., Demeaux, J., Tessier, C., and Martinez, H. (2020b). Influence of the cathode potential on electrode interactions within a Li4Ti5O12 vs LiNi3/5Mn1/5Co1/5O2 Li-Ion battery. J. Electrochem. Soc. 167:040504. doi: 10.1149/1945-7111/ab7116
Gauthier, N., Courrèges, C., Demeaux, J., Tessier, C., and Martinez, H. (2020c). Impact of the cycling temperature on electrode/electrolyte interfaces within Li4Ti5O12 vs LiMn2O4 cells. J. Power Sources 448:227573. doi: 10.1016/j.jpowsour.2019.227573
Ge, H., Li, N., Li, D., Dai, C., and Wang, D. (2009). Study on the theoretical capacity of spinel lithium titanate induced by low-potential intercalation. J. Phys. Chem. C 113, 6324–6326. doi: 10.1021/jp9017184
Gieu, J.-B., Courrèges, C., Ouatani, L. E., Tessier, C., and Martinez, H. (2017). Influence of vinylene carbonate additive on the Li4Ti5O12 electrode/electrolyte interface for lithium-Ion batteries. J. Electrochem. Soc. 164, A1314–A1320. doi: 10.1149/2.0111707jes
Gieu, J. B., Courrèges, C., El Ouatani, L., Tessier, C., and Martinez, H. (2016). Temperature effects on Li4Ti5O12 electrode/electrolyte interfaces at the first cycle: a X-ray photoelectron spectroscopy and scanning auger microscopy study. J. Power Sources 318, 291–301. doi: 10.1016/j.jpowsour.2016.04.007
Goodenough, J. B., and Kim, Y. (2010). Challenges for rechargeable li batteries†. Chem. Mater. 22, 587–603. doi: 10.1021/cm901452z
Guo, J., Zuo, W., Cai, Y., Chen, S., Zhang, S., and Liu, J. (2015). A novel Li4Ti5O12-based high-performance lithium-Ion electrode at elevated temperature. J. Mater. Chem. A 3, 4938–4944. doi: 10.1039/C4TA05660D
Han, C., He, Y.-B., Li, H., Li, B., Du, H., Qin, X., et al. (2015). Suppression of interfacial reactions between Li4Ti5O12 electrode and electrolyte solution via zinc oxide coating. Electrochim. Acta 157, 266–273. doi: 10.1016/j.electacta.2014.12.080
He, M., Castel, E., Laumann, A., Nuspl, G., Novák, P., and Berg, E. J. (2015). In situ gas analysis of Li 4 Ti 5 O 12 based electrodes at elevated temperatures. J. Electrochem. Soc. 162, A870–A876. doi: 10.1149/2.0311506jes
He, Y.-B., Liu, M., Huang, Z.-D., Zhang, B., Yu, Y., Li, B., et al. (2013). Effect of solid electrolyte interface (SEI) film on cyclic performance of Li4Ti5O12 anodes for Li Ion batteries. J. Power Sources 239, 269–276. doi: 10.1016/j.jpowsour.2013.03.141
He, Y.-B., Ning, F., Li, B., Song, Q.-S., Lv, W., Du, H., et al. (2012b). Carbon coating to suppress the reduction decomposition of electrolyte on the Li4Ti5O12 electrode. J. Power Sources 202, 253–261. doi: 10.1016/j.jpowsour.2011.11.037
He, Y. B., Li, B., Liu, M., Zhang, C., Lv, W., Yang, C., et al. (2012a). Gassing in Li(4)Ti(5)O(12)-based batteries and its remedy. Sci. Rep. 2:913. doi: 10.1038/srep00913
Hernandez, V. S., Martinez, L. M. T., Mather, G. C., and West, A. R. (1996). Stoichiometry, structures and polymorphism of spinel-like phases, Li1.33x Zn2−2x Ti1+0.67x O4. J. Mater. Chem. 6, 1533–1536. doi: 10.1039/jm9960601533
Hsieh, C.-T., and Lin, J.-Y. (2010). Influence of Li addition on charge/discharge behavior of spinel lithium titanate. J. Alloys Compd. 506, 231–236. doi: 10.1016/j.jallcom.2010.06.183
Jana, A., and García, R. E. (2017). Lithium dendrite growth mechanisms in liquid electrolytes. Nano Energy 41, 552–565. doi: 10.1016/j.nanoen.2017.08.056
Jansen, A. N., Kahaian, A. J., Kepler, K. D., Nelson, P. A., Amine, K., Dees, D. W., et al. (1999). Development of a high-power lithium-Ion battery. J. Power Sources 81–82, 902–905. doi: 10.1016/S0378-7753(99)00268-2
Jin, Y., Yu, H., Gao, Y., He, X., White, T. A., and Liang, X. (2019). Li4Ti5O12 coated with ultrathin aluminum-doped zinc oxide films as an anode material for lithium-Ion batteries. J. Power Sources 436:226859. doi: 10.1016/j.jpowsour.2019.226859
Jovic, N., Antic, B., Kremenovic, A., Spasojevic-de Bire, A., and Spasojevic, V. (2003). Cation ordering and order–disorder phase transition in Co-substituted Li4Ti5O12 spinels. Phys. Status Solidi 198, 18–28. doi: 10.1002/pssa.200306451
Jung, H.-G., Myung, S.-T., Yoon, C. S., Son, S.-B., Oh, K. H., Amine, K., et al. (2011). Microscale spherical carbon-coated Li4Ti5O12 as ultra-high power anode material for lithium batteries. Energy Environ. Sci. 4, 1345–1351. doi: 10.1039/c0ee00620c
Kalhoff, J., Eshetu, G. G., Bresser, D., and Passerini, S. (2015). Safer electrolytes for lithium-Ion batteries: state of the art and perspectives. ChemSusChem 8, 2154–2175. doi: 10.1002/cssc.201500284
Kang, B., and Ceder, G. (2009). Battery materials for ultrafast charging and discharging. Nature 458, 190–193. doi: 10.1038/nature07853
Kawamura, T., Okada, S., and Yamaki, J.-I. (2006). Decomposition reaction of LiPF6-based electrolytes for lithium Ion cells. J. Power Sources 156, 547–554. doi: 10.1016/j.jpowsour.2005.05.084
Khan, S. U., Al-Shahry, M., and Ingler, W. B. Jr. (2002). Efficient photochemical water splitting by a chemically modified n-TiO2. Science 297, 2243–2245. doi: 10.1126/science.1075035
Kitta, M., Akita, T., Maeda, Y., and Kohyama, M. (2012). Study of surface reaction of spinel Li4Ti5O12 during the first lithium insertion and extraction processes using atomic force microscopy and analytical transmission electron microscopy. Langmuir 28, 12384–12392. doi: 10.1021/la301946h
Kitta, M., Matsuda, T., Maeda, Y., Akita, T., Tanaka, S., Kido, Y., et al. (2014). Atomistic structure of a spinel Li4Ti5O12 (111) surface elucidated by scanning tunneling microscopy and medium energy Ion scattering spectrometry. Surf. Sci. 619, 5–9. doi: 10.1016/j.susc.2013.09.026
Kubiak, P., Garcia, A., Womes, M., Aldon, L., Olivier-Fourcade, J., Lippens, P.-E., et al. (2003). Phase transition in the spinel Li4Ti5O12 induced by lithium insertion. J. Power Sources 119–121, 626–630. doi: 10.1016/S0378-7753(03)00186-1
Li, H., Shen, L., Yin, K., Ji, J., Wang, J., Wang, X., et al. (2013). Facile synthesis of N-doped carbon-coated Li4Ti5O12 microspheres using polydopamine as a carbon source for high rate lithium Ion batteries. J. Mater. Chem. A 1, 7270–7276. doi: 10.1039/c3ta10623c
Li, W., Chen, M., Jiang, J., Wu, R., Wang, F., Liu, W., et al. (2015). Structural and electrochemical characteristics of SiO2 modified Li4Ti5O12 as anode for lithium-Ion batteries. J. Alloys Compd. 637, 476–482. doi: 10.1016/j.jallcom.2015.03.049
Li, X., Zhao, X., Huang, P., Wang, M., Huang, Y., Zhou, Y., et al. (2017). Enhanced electrochemical performance of SrF2-modified Li4Ti5O12 composite anode materials for lithium-Ion batteries. J. Alloys Compd. 693, 61–69. doi: 10.1016/j.jallcom.2016.09.089
Liu, J., Bian, P., Li, J., Ji, W., Hao, H., and Yu, A. (2015). Gassing behavior of lithium titanate based lithium Ion batteries with different types of electrolytes. J. Power Sources 286, 380–387. doi: 10.1016/j.jpowsour.2015.03.172
Liu, J., Li, X., Cai, M., Li, R., and Sun, X. (2013). Ultrathin atomic layer deposited ZrO2 coating to enhance the electrochemical performance of Li4Ti5O12 as an anode material. Electrochim. Acta 93, 195–201. doi: 10.1016/j.electacta.2012.12.141
Liu, W., Liu, H., Wang, Q., Zhang, J., Xia, B., and Min, G. (2017). Gas swelling behaviour at different stages in Li4Ti5O12/LiNi1/3Co1/3Mn1/3O2 pouch cells. J. Power Sources 369, 103–110. doi: 10.1016/j.jpowsour.2017.10.001
Lu, X., Gu, L., Hu, Y. S., Chiu, H. C., Li, H., Demopoulos, G. P., et al. (2015). New insight into the atomic-scale bulk and surface structure evolution of Li4Ti5O12 anode. J. Am. Chem. Soc. 137, 1581–1586. doi: 10.1021/ja5115562
Lu, X., Zhao, L., He, X., Xiao, R., Gu, L., Hu, Y. S., et al. (2012). Lithium storage in Li4Ti5O12 spinel: the full static picture from electron microscopy. Adv. Mater. Weinheim 24, 3233–3238. doi: 10.1002/adma.201200450
Lv, W., Gu, J., Niu, Y., Wen, K., and He, W. (2017). Review—gassing mechanism and suppressing solutions in Li 4 Ti 5 O 12 -based lithium-Ion batteries. J. Electrochem. Soc. 164, A2213–A2224. doi: 10.1149/2.0031712jes
Milien, M. S., Hoffmann, J., Payne, M., and Lucht, B. L. (2018). Effect of electrolyte additives on Li 4 Ti 5 O 12 cycling performance and gas evolution. J. Electrochem. Soc. 165, A3925–A3931. doi: 10.1149/2.0741816jes
Ohzuku, T. (1995). Zero-strain insertion material of Li[Li 1/3Ti 5/3]O 4 for rechargeable lithium cells. J. Electrochem. Soc. 142:1431. doi: 10.1149/1.2048592
Okamoto, Y. (2013). Ab initio calculations of thermal decomposition mechanism of LiPF6-based electrolytes for lithium-Ion batteries. J. Electrochem. Soc. 160, A404–A409. doi: 10.1149/2.020303jes
Park, K. S., Benayad, A., Kang, D. J., and Doo, S. G. (2008). Nitridation-driven conductive Li4Ti5O12 for lithium ion batteries. J. Am. Chem. Soc. 130, 14930–14931. doi: 10.1021/ja806104n
Park, S. H., Park, K. S., Cho, M. H., Sun, Y. K., Nahm, K. S., Lee, Y. S., et al. (2002). The effects of oxygen flow rate and anion doping on the performance of the LiNiO2 electrode for lithium secondary batteries. Korean J. Chem. Eng. 19, 791–796. doi: 10.1007/BF02706969
Qin, Y., Chen, Z., and Amine, K. (2011). Functionalized Surface Modification Agents to Suppress Gassing Issue of Li4Ti5O12-Based Lithium-Ion Chemistry. Lemont, IL: Argonne National Laboratory, FY 2011 Annual Progress Report for Energy Storage R&D.
Sawai, K., Iwakoshi, Y., and Ohzuku, T. (1994). Carbon materials for lithium-ion (shuttlecock) cells. Solid State Ionics 69, 273–283. doi: 10.1016/0167-2738(94)90416-2
Schiele, A., Hatsukade, T., Berkes, B. B., Hartmann, P., Brezesinski, T., and Janek, J. (2017). High-throughput in situ pressure analysis of lithium-Ion batteries. Anal. Chem. 89, 8122–8128. doi: 10.1021/acs.analchem.7b01760
Schweidler, S., de Biasi, L., Schiele, A., Hartmann, P., Brezesinski, T., and Janek, J. (2018). Volume changes of graphite anodes revisited: a combined operando X-ray diffraction and in situ pressure analysis study. J. Phys. Chem. C 122, 8829–8835. doi: 10.1021/acs.jpcc.8b01873
Scrosati, B., and Garche, J. (2010). Lithium batteries: Status, prospects and future. J. Power Sources 195, 2419–2430. doi: 10.1016/j.jpowsour.2009.11.048
Shkrob, I. A., Zhu, Y., Marin, T. W., and Abraham, D. (2013). Reduction of carbonate electrolytes and the formation of solid-electrolyte interface (SEI) in lithium-Ion batteries. 1. spectroscopic observations of radical intermediates generated in one-electron reduction of carbonates. J. Phys. Chem. C 117, 19255–19269. doi: 10.1021/jp406274e
Shu, J. (2009). Electrochemical behavior and stability of Li4Ti5O12 in a broad voltage window. J. Solid State Electrochem. 13, 1535–1539. doi: 10.1007/s10008-008-0723-z
Sloop, S. E., Kerr, J. B., and Kinoshita, K. (2003). The role of Li-ion battery electrolyte reactivity in performance decline and self-discharge. J. Power Sources 119–121, 330–337. doi: 10.1016/S0378-7753(03)00149-6
Sloop, S. E., Pugh, J. K., Wang, S., Kerr, J. B., and Kinoshita, K. (2001). Chemical reactivity of PF[sub 5] and LiPF[sub 6] in ethylene carbonate/dimethyl carbonate solutions. Electrochem. Solid-State Lett. 4. A42–A44. doi: 10.1149/1.1353158
Sorensen, E. M., Barry, S. J., Jung, H.-K., Rondinelli, J. M., Vaughey, J. T., and Poeppelmeier, K. R. (2006). Three-dimensionally ordered macroporous Li4Ti5O12: effect of wall structure on electrochemical properties. Chem. Mater. 18, 482–489. doi: 10.1021/cm052203y
Sun, X., Hegde, M., Zhang, Y., He, M., Gu, L., Wang, Y., et al. (2014). Structure and electrochemical properties of spinel Li4Ti5O12 nanocomposites as anode for lithium-Ion battery. Int. J. Electrochem. Sci. 9:1583. Available online at: http://www.electrochemsci.org/papers/vol9/90401583.pdf
Sun, X., Radovanovic, P. V., and Cui, B. (2015). Advances in spinel Li4Ti5O12 anode materials for lithium-ion batteries. N. J. Chem. 39, 38–63. doi: 10.1039/C4NJ01390E
Tan, T., Yumoto, H., Zhang, Q., and Taggougui, M. (2012). Lithium Titanate Cell With Reduced Gassing. Indianapolis, IN: US Patent 8168330 B2.
Tikhonov, K., and Lin, T. Y. (2015). Surface Modification of Battery Materials and Method for Making a Battery. Waltham, MA: US Patent 8999009 B2.
Verma, P., Maire, P., and Novák, P. (2010). A review of the features and analyses of the solid electrolyte interphase in Li-ion batteries. Electrochim. Acta 55, 6332–6341. doi: 10.1016/j.electacta.2010.05.072
Wang, G. X., Bradhurst, D. H., Dou, S. X., and Liu, H. K. (1999). Spinel Li[Li1/3Ti5/3]O4 as an anode material for lithium ion batteries. J. Power Sources 83, 156–161. doi: 10.1016/S0378-7753(99)00290-6
Wang, Q., Zhang, J., Liu, W., Xie, X., and Xia, B. (2017). Quantitative investigation of the gassing behavior in cylindrical Li4Ti5O12 batteries. J. Power Sources 343, 564–570. doi: 10.1016/j.jpowsour.2017.01.073
Wang, R., Li, X., Zhang, B., Wang, Z., and Guo, H. (2015). Effect of methylene methanedisulfonate as an additive on the cycling performance of spinel lithium titanate electrode. J. Alloys Compd. 648, 512–520. doi: 10.1016/j.jallcom.2015.06.225
Wang, R. H., Li, X. H., Wang, Z. X., Guo, H. J., and He, Z. J. (2015). Electrochemical analysis for enhancing interface layer of spinel Li4Ti5O12: p-toluenesulfonyl isocyanate as electrolyte additive. ACS Appl. Mater. Interfaces 7, 23605–23614. doi: 10.1021/acsami.5b07047
Wang, S., Liu, J., Rafiz, K., Jin, Y., Li, Y., and Lin, Y. S. (2019). An on-line transient study on gassing mechanism of lithium titanate batteries. J. Electrochem. Soc. 166, A4150–A4157. doi: 10.1149/2.0631916jes
Wang, S., Quan, W., Zhu, Z., Yang, Y., Liu, Q., Ren, Y., et al. (2017). Lithium titanate hydrates with superfast and stable cycling in lithium ion batteries. Nat. Commun. 8:627. doi: 10.1038/s41467-017-00574-9
Wen, L., Wu, Z., Zhao, P., Liang, J., Luo, H., Liu, G., et al. (2019). Effect of formation potentials on gassing of LiMn 2 O 4 //Li 4 Ti 5 O 12 /C batteries. J. Electrochem. Soc. 166, A5033–A5037. doi: 10.1149/2.0081903jes
Wilken, S., Treskow, M., Scheers, J., Johansson, P., and Jacobsson, P. (2013). Initial stages of thermal decomposition of LiPF6-based lithium ion battery electrolytes by detailed raman and NMR spectroscopy. RSC Adv. 3, 16359–16364. doi: 10.1039/c3ra42611d
Winter, M., Besenhard, J. O., Spahr, M. E., and Novák, P. (1998). Insertion electrode materials for rechargeable lithium batteries. Adv. Mater. 10, 725–763. doi: 10.1002/(SICI)1521-4095(199807)10:10<725::AID-ADMA725>3.0.CO;2-Z
Wu, K., Yang, J., Liu, Y., Zhang, Y., Wang, C., Xu, J., et al. (2013b). Investigation on gas generation of Li4Ti5O12/LiNi1/3Co1/3Mn1/3O2 cells at elevated temperature. J. Power Sources 237, 285–290. doi: 10.1016/j.jpowsour.2013.03.057
Wu, K., Yang, J., Qiu, X.-Y., Xu, J.-M., Zhang, Q.-Q., Jin, J., et al. (2013a). Study of spinel Li4Ti5O12 electrode reaction mechanism by electrochemical impedance spectroscopy. Electrochim. Acta 108, 841–851. doi: 10.1016/j.electacta.2013.07.048
Xiao, X., Verbrugge, M. W., and Wang, J. S. (2015). Multifunctional Hybrid Coatings for Electrodes Made by Atomic Layer Deposition Techniques. Detroit, MI: US Patent 20150180023 A1.
Xu, G., Han, P., Dong, S., Liu, H., Cui, G., and Chen, L. (2017). Li 4 Ti 5 O 12 -based energy conversion and storage systems: status and prospects. Coord. Chem. Rev. 343, 139–184. doi: 10.1016/j.ccr.2017.05.006
Yao, X. L., Xie, S., Chen, C. H., Wang, Q. S., Sun, J. H., Li, Y. L., et al. (2005). Comparisons of graphite and spinel Li1.33Ti1.67O4 as anode materials for rechargeable lithium-ion batteries. Electrochim. Acta 50, 4076–4081. doi: 10.1016/j.electacta.2005.01.034
Yi, T.-F., Jiang, L.-J., Shu, J., Yue, C.-B., Zhu, R.-S., and Qiao, H.-B. (2010). Recent development and application of Li4Ti5O12 as anode material of lithium ion battery. J. Phys. Chem. Solids 71, 1236–1242. doi: 10.1016/j.jpcs.2010.05.001
Yuan, T., Yu, X., Cai, R., Zhou, Y., and Shao, Z. (2010). Synthesis of pristine and carbon-coated Li4Ti5O12 and their low-temperature electrochemical performance. J. Power Sources 195, 4997–5004. doi: 10.1016/j.jpowsour.2010.02.020
Zaghib, K., Simoneau, M., Armand, M., and Gauthier, M. (1999). Electrochemical study of Li4Ti5O12 as negative electrode for Li-ion polymer rechargeable batteries. J. Power Sources 81–82, 300–305. doi: 10.1016/S0378-7753(99)00209-8
Keywords: lithium titanate battery, lithium ion battery, stability, electrolyte, anode, solid electrolyte interphase layer
Citation: Ghosh A and Ghamouss F (2020) Role of Electrolytes in the Stability and Safety of Lithium Titanate-Based Batteries. Front. Mater. 7:186. doi: 10.3389/fmats.2020.00186
Received: 31 March 2020; Accepted: 20 May 2020;
Published: 10 July 2020.
Edited by:
Lorenzo Malavasi, University of Pavia, ItalyCopyright © 2020 Ghosh and Ghamouss. This is an open-access article distributed under the terms of the Creative Commons Attribution License (CC BY). The use, distribution or reproduction in other forums is permitted, provided the original author(s) and the copyright owner(s) are credited and that the original publication in this journal is cited, in accordance with accepted academic practice. No use, distribution or reproduction is permitted which does not comply with these terms.
*Correspondence: Fouad Ghamouss, Zm91YWQuZ2hhbW91c3NAdW5pdi10b3Vycy5mcg==