- 1School of Civil Engineering, Faculty of Engineering, Universiti Teknologi Malaysia, Skudai, Malaysia
- 2Department of Civil Engineering, College of Engineering, University of Kufa, Kufa, Iraq
- 3Department of Civil Engineering, Faculty of Engineering and Built Environment, Universiti Kebangsaan Malaysia, Selangor, Malaysia
In recent years, the proliferation of plastic waste has become a global problem. A potential solution to this problem is the dry process, which incorporates plastic waste into asphalt mixtures. However, the dry process often has inconsistent performance due to poor interaction with binder and improper distribution of plastic waste particles in the mixture skeleton. This inconsistency may be caused by inaccurate mixing method, shredding size, mixing temperature and ingredient priorities. Thus, this study aims to improve the consistency of the dry process by comparing the control asphalt mixture and two plastic waste-modified asphalt mixtures prepared using the dry process. This study used crushed granite aggregate with the nominal maximum aggregate size of 14 mm whereas the shredded plastic bag is in the range of 5–10 mm. Quantitative sieving analysis and performance tests were carried out to examine the effects of plastic waste added into the asphalt mixture. The volumetric and performance properties combined with image analysis of the modified mixtures were obtained and compared with the control mixture. In addition, the moisture damage, resilient modulus, creep deformation and rutting were evaluated. This study also highlighted in detail the distribution of plastic particles in the final skeleton of the asphalt mixture. Based on the analysis, an enhanced dry process of mixing procedure was proposed and evaluated. Results showed that the addition of plastic particles using the conventional dry process leads to the deviation in the aggregate structure as high plastic content is added. Furthermore, the enhanced dry process developed in this study presents substantial enhancement in the asphalt performance, particularly with plastic waste that accounts for 20% of the weight of the asphalt binder.
Introduction
The significant increase in plastic production and usage creates severe health, economic and territorial issues. Thus, these issues have driven a strong movement toward the adoption of sustainable construction technology to reduce plastic wastes. Plastics are low-density (lightweight), durable, formable, and low-cost materials that are widely used in a variety of applications due to their properties. Several studies have upcycled existing plastic wastes to develop better quality asphalt mixtures. The behavior of asphalt mixture onsite varies and it relies on two key parameters, namely, the ambient temperature and the traffic speed. Asphalt binder behaves more like an elastic solid at high speeds and low temperatures. In this case, fatigue cracks are the most common type of deterioration that occurs under high vehicle speeds and low temperature conditions. On the other hand, the asphalt binder behaves as high viscous material at low speeds and high temperatures, and the most common type of deterioration is rutting deformation. Thus, asphalt binder is commonly used in a modified form to reduce the effects of rising traffic volumes and bad weather (Köfteci et al., 2014; Bera and Babadagli, 2015). The wet process is currently the most widely used method for polymer asphalt modification because of its improved thermal behavior (Mohammad et al., 2011). Numerous studies proved the efficacy and reliability of modified mixtures prepared using the wet process. During the wet process, the polymers are directly added to the hot asphalt before being used in the production of the modified asphalt mixtures (Huang et al., 2007). The modified mixture has lower fatigue, longer life and higher resistance to permanent deformations (Huang et al., 2000; Attaelmanan et al., 2011; González et al., 2012; Oliviero Rossi et al., 2015). Although the wet process is widely used, this process has some significant limitations. Usage of inadequate and incompatible polymers leads to heterogeneous asphalt mixtures without cohesion and ductility (Polacco et al., 2005; Yu et al., 2015). Nevertheless, if the polymer-modified asphalt prepared using the wet method is stockpiled at high temperatures without agitation, then some storage stability problems could appear (Brasileiro et al., 2019). Although the use of special tanks equipped with a stabilizing agent could avoid problems related to asphalt binder’s storage, they will increase the manufacturing cost of the asphalt mixtures (Becker et al., 2001; Yu et al., 2015). Thus, the use of the proper and compactable polymer in the wet process must be maintained under strict conditions to produce a properly modified asphalt binder (Lo Presti, 2013). The mixing method must be done in a high-speed mixer at a high temperature to produce a high-performance asphalt binder with the desired properties (Montanelli and srl, 2013; Al-Adham and Al-Abdul Wahhab, 2018). Such a complicated mixing process greatly increases the production cost, as a specially designed mixing plant was required to fulfill a completely homogenous modified binder with a proper melting and digestion of plastic throughout the binder (Lastra-González et al., 2016; Fernandes et al., 2017). Moreover, the dry process does not have such double handling problems. During the dry process mixing, plastic waste is added to the hot aggregate before producing the asphalt binder using the existing facilities. The combination of plastic and the aggregate creates a thin layer of plastic-coated particles with improved properties (Huang et al., 2007; Lastra-González et al., 2016). The interaction between the plastic particles and the rest of the mixture depends on the mixing time and the plastic’s particle size and softening point of the plastic particles (Huang et al., 2007; Ahmadinia et al., 2012). Many previous studies that used the dry process with low and high-density polyethene reported that Marshall stability, indirect tensile strength (ITS) and indirect tensile strength ratio (TSR) improved (Huang et al., 2000; Zoorob and Suparma, 2000; Ahmadinia et al., 2011; Yin and Wu, 2018). However, the dry process performs inconsistently, especially when using a high plastic content in the asphalt mixtures (Lastra-González et al., 2016; Mishra and Gupta, 2018). This performance fluctuation may be due to the preparation method, mixing method, temperature and other factors that regulate the produced mixtures. These factor contribute to the poor interaction between the plastic waste and binder during mixing process. The mixture performance prepared through the dry process can be optimized by controlling these factors.
After considering the advantages and disadvantages of the dry and wet processes, this study aims to develop an enhanced method that incorporate the plastic waste into the asphalt mixtures. The study used a modified dry procedure to optimize the interaction between aggregate, binder, and plastic. For the conventional dry method, the plastic waste was added to the hot aggregate before mixing with the asphalt binder. In this study, the plastic waste is added to the coarse aggregate and then a portion of the optimum asphalt content (OAC) was mixed with the coated plastic aggregate for 2 min. This process was conducted to ensure a high digestion rate of the thin melted layer on the aggregate with the asphalt binder. Once the asphalt binder comes into contact with the hot aggregate, the high aggregate temperature can boost the plastic-asphalt digestion process and creates a thin layer of modified binder on the resulting aggregate surface. The significance of this study is the high percentage of plastic waste used in this newly proposed modified method in addition to the high digestion between the asphalt and plastic. The plastic waste content of 20% by weight of the asphalt binder was used to enhance the modified mixture’s properties compared with the control mixture.
Materials and Methods
Asphalt Binder and Aggregates
An asphalt binder with a 60/70 penetration grade was used in this study, and Table 1 shows the list of the properties of the binder. Granite aggregate types were obtained from Hanson Quarry Products Sdn. Bhd., a local quarry in Johor, Malaysia, which were then sieved and packed. The samples were prepared in accordance with Malaysian Standard Specification for Road Works (JKR) (Jabatan Kerja Raya Malaysia (JKR), 2008) to obtain the aggregate gradation envelope of asphaltic concrete with 14 mm (AC14). The combined gradation for each aggregate particle size, which consists of coarse aggregate, fine aggregate, and mineral filler for AC14 are further discussed in Gradation Analysis of Aggregate and Plastic Mixture. The mineral filler used in this study is a combination of quarry dust and 2% of hydrated lime based on the total weight of the mixture as specified in Malaysian Standard Specification for Road Works (Jabatan Kerja Raya Malaysia (JKR), 2008). A hydrated lime of 2% by designated weight of the aggregates was added into the asphalt mixture with accordance to JKR specification as an anti-stripping agent to improve the performance of the asphalt mixture.
Plastic Waste
This study utilized a polymer-based waste material (low-density polyethylene), which is in the form of plastic bags. The plastic bags were shredded to an approximate size of 5–10 mm, as shown in Figure 1. This small size range was chosen to increase the homogeneity of the mixture during the blending process (Movilla-Quesada et al., 2019a). The density of the shredded plastic was determined using a gas pycnometer (Micrometrics Accupyc II TEC). The gas displacement method was used to perform this test. Then, helium gas was used as the displacement medium to measure the volume. The calculated specific gravity of the plastic waste sample was 1.0770. Moreover, the plastic was observed to completely melt when mixed with aggregate at 180°C for 4 min. Thus, 180°C was selected as the mixing temperature for preparing plastic waste-modified mixtures in this study. This study used two plastic waste content (0.5%, 1%) based on the weight of total aggregate as recommended by previous studies (Movilla-Quesada et al., 2019b; Sasidharan et al., 2019).
Mixture Preparation
The study prepared two plastic waste mixtures through the dry process and one control mixture. The mixtures were prepared with OAC of 5% based on Marshall test procedure and volumetric analysis. The plastic waste-modified mixtures were prepared using the conventional dry process (PMIX-C) and a modified dry process (PMIX-E). PMIX-C involved heating the aggregate at 180°C for 2 h before gradually adding the plastic particles to the entire aggregate (as new materials). Then, the plastic and aggregate were mixed with the asphalt binder. On the other hand, the preparation procedure of PMIX-E mixtures involves three steps, as outlined in Figure 2. The first step includes heating the coarse aggregate (>3.35 mm) to a temperature of 180 ± 5°C before gradually adding the shredded plastics to the aggregate. The mixture was properly mixed for 4 min to ensure that the plastic particles had melted and coated the aggregate. The next step includes mixing the coated plastic aggregate with a portion of the OAC (17% of the total weight of asphalt content, 5%) for 2 min. This procedure was conducted to ensure high digestion of the thin melted layer on the aggregate using the asphalt binder. Once the asphalt binder comes into contact with the hot aggregate, the high aggregate temperature can boost the plastic–asphalt digestion process and creates a thin layer of modified binder on the resulting aggregate surface. The 17% of the total weight of asphalt content, 5% was calculated based on the ratio of surface area of coarse aggregate to the total area of aggregate. The area of aggregate particles surface was calculated based on the factors developed by Anochie-Boateng (Anochie-Boateng et al., 2011). The last step was to add the rest of the hot fine aggregate and binder to the produced mixture and mix it until a homogenous asphalt mixture is produced.
Test Methods
This study was divided into three phases. In the first phase, three asphalt mixtures were prepared, namely, a control mix (CMIX) and two plastic waste-modified asphalt mixtures prepared using two different procedures of the dry process (PMIX-C and PMIX-E). During the second phase, the gradation of produced aggregate before and after adding the shredded plastic was analyzed. In the last phase, the volumetric properties and the performance properties of the produced mixture were evaluated.
Aggregate Gradation Analysis
Gradation analysis helps to determine the particle size distribution of the coarse and fine aggregates for the produced mixtures. The combined gradation for each aggregate particle size, which consists of coarse aggregate, fine aggregate and mineral filler for AC14, was sieved and weighed carefully before adding the plastic waste to prepare PMIX-C and PMIX-E. Next, the specified aggregate gradation was heated to 180°C and mixed with 0.5 and 1.0% plastic. After mixing, the combined aggregate was sieved and weighed again to determine the effect of the plastic particles added on the final gradation for both modification methods.
Stability and Volumetric Properties
In the laboratory, the aggregate, binder and plastic wastes were mixed and compacted at 180 ± 5°C and 170 ± 5°C, respectively. The samples were compacted to 75 blows for each side of the samples. A total of five different mixture blends were prepared for this study with each blend consisting of 20 samples. The air voids content, density and voids filled with asphalt (VFA) of each sample were determined in accordance with ASTM D2726. The stability test and the volumetric properties were utilized to determine the optimum binder content of the mixtures according to the Marshall procedure specified by the Malaysian Public Works Department (2008) (Jabatan Kerja Raya Malaysia (JKR), 2008). That is, samples are dry-weighted and then saturated underwater, and the bulk density was calculated using the water volume displaced. The maximum theoretical density was determined according to ASTM D2041(American Society for Testing Materials. ASTM D2041/D2041M-11, 2011). The air void content of the samples was determined according to ASTM D3203-11 specification. The samples were then conditioned at 60°C for 45 min to determine the stability and flow values by using the Marshall stability test as stated in the ASTM D1559 (American Society for Testing Materials ASTM D1559-89, 1989). The obtained stability corresponds to the maximum load, in N, and the flow, in mm, that the sample recorded when reaching the maximum load value.
Moisture Damage
A total of 30 compacted Marshall samples were exposed to 50 blows per side to void content matching, which is anticipated in the field (approximately 7% air void content). The preparation of asphalt concrete samples and the analysis of moisture damage upon tensile strength were performed according to the ASTM D4867/D4867M (ASTM D4867, 2014). According to the specification, six samples were prepared for each of the three combinations, which were divided into two groups, wet conditioned and unconditioned samples. The former samples were vacuumed at an absolute pressure of 30 mmHg for 5 min for partial saturation before being sent to a 24 h water bath at 60°C, followed by another 2 h water bath at 20°C before the test. The saturation level was calculated by dividing the volume of water absorbed by the volume of voids, with the result expressed as a percentage. According to ASTM standards D4867/D4867M-09 (ASTM D4867, 2014), the saturation level must be between 55 and 80%. After conditioning, all samples were tested under the indirect tensile strength (ITS) test at a displacement rate of 50.8 mm/min until the maximum load. The ITS test was performed to create tensile stresses along the diametric axis of the test sample. The maximum load at fracture was measured to determine the ITS using Eq. 1:
where StD is the (ITS) (kPa); P is the peak load (N); D and t are the diameter and the height of the sample (mm), respectively. The TSR value was calculated using the ratio between the ITS of wet conditioned and unconditioned sample groups, as shown in Eq. 2:
where TSR is the conserved tensile strength ratio (%); StW is the ITS calculated for wet samples (kPa); StD is the ITS calculated for unconditioned samples (kPa).
Resilient Modulus Test
The elastic behavior of the control and modified mixtures under repetitive loading was evaluated through the MR test using a universal material testing apparatus based on the ASTM D4123–82 (ASTM D4123-82, 1995) standard. The MR test was considered the key aspect of the mechanistic design of pavement structure. The test examined the asphalt pavement mixture’s elastic deformation resistance and its ability to absorb the applied energy without any deformation and revert to its initial condition after loading. For each mixture design, three samples were conditioned at the tested temperature for at least 3 h before testing. During the standardized test, the samples were subjected to compressive loads (stress) of 1,000 N with a loading wave defined by the haversine function. The MR test instrument was set to apply a pulse repetition period of up to 1 s at 25 and 40°C. The test was conducted at 25 and 40°C to estimate the potential of fatigue cracking and rutting, respectively. The applied load was diametrically vertical to the plane of the cylindrical sample with a duration of 0.1 s and a rest period of 0.9 s. Two tests were run for each conditioned sample in different orientations. The load and deformation were automatically recorded using a computer connected to the test instrument, which subsequently calculated the modulus of resilience based on the assumed Poisson ratio. For samples tested at 25°C and 0.40, respectively (Zak et al., 2014).
Dynamic Creep Test
The dynamic creep test is a destructive test conducted to estimate the rutting potential of the asphalt mixture by using the UTM machine. The test was carried out following the BS EN 12697-25 (BS EN 12697-25, 2013). The samples were conditioned at 40°C for at least 3 h in dry condition before the test. Prior to the actual test, the pre-condition parameters were set up as follows: preloaded stress of 150 kPa, preloading time of 30 s and loading frequency of 0.5 Hz with a loading time of 0.2 s. During the actual test, the applied axial stress and load cycles were set up at 300 kPa and 3,600 cycles, respectively. The 3,600 cycles were set up to analyze the deformation trends of the asphalt mixture sample under repetitive axial loading stress.
From this test, the creep stiffness modulus and creep strain slope were computed using Eqs 3,4.
where E = creep stiffness modulus (MPa), σ = applied stress (kPa), ε = cumulative axial strain at 3,600 cycles (mm), CSS = creep strain slope, ε3600 = strain at 3,600 cycles, ε1200 = Strain at 1,200 cycles.
Wheel Tracking Test
The wheel tracking test was used to evaluate the susceptibility of asphalt mixtures to deformation under a dynamic load by quantifying the rut depth formed by the cycles of passes of a loaded wheel at a fixed temperature. Asphalt mixture samples (64 mm height and 150 mm diameter) were prepared based on the designed asphalt content and 7 ± 1% air voids using a gyratory compactor. The air void content of sample was determined based on ASTM D3203-11 () specification Two samples were prepared for each mixture and were conditioned inside double wheel tracker equipment at 50°C, for 5 h to be confirmed with the EN 12697-22 standard (BS EN 12697-22, 2013) as well as to evaluate the potential of asphalt mixture deformation at high temperature. Later, the samples were exposed to 10,000 cycles at a speed of 26.5 cycles/min and 70 N wheel loading. Raw data were recorded automatically for rut depth vs. the number of cycles. Test termination criteria were limited to 10,000 cycles or a maximum rut depth of 20 mm. The criterion of the rutting potential was based on the cumulative rut depth.
Result and Discussion
Gradation Analysis of Aggregate and Plastic Mixture
Table 2 presents the list of the weights of each sieve size of PMIX-C and PMIX-E. Specifically, Table 2 shows a high deviation in the designed gradation when plastic was added to PMIX-C. For PMIX-C, the retained weight on sieve size from 14 to 1.18 mm increased by 15%, whereas the retained weight on sieve size from 0.425 mm to pan decreased by almost the same percentage. The increase was due to the accumulation of plastic waste and fine aggregate to produce new plastic, as indicated by the red arrows in Figure 3A. Moreover, another form of clusters of plastic with fine aggregate is observed during the sieve analysis, and these clusters are generated due to the affinity of melted plastic to adhere to the filler (fine aggregate-based sieve size of 0.075 mm). Thus, the generated plastic particles have external textures of this filler, which prevent the plastic particles from melting inside the asphalt mixtures, as indicated in Figure 3A. These clusters reduce the weight of fine aggregate by 24 and 30% for PMIX-C with 0.5 and 1%, respectively. Moreover, the plastic particles melted on the heated aggregate tended to adhere to other particles to produce larger ones, as shown in Figure 3B. Based on visual examination, these new particles generated are fragile and can easily shatter under loads. Thus, this new particle can create weak spots in the skeleton of the modified asphalt mixtures.
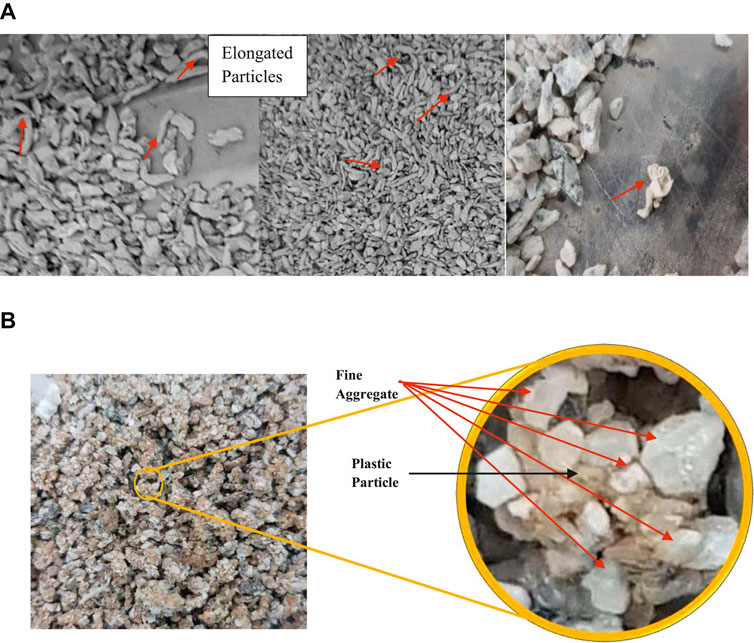
FIGURE 3. Plastic waste particle transformation in final gradation. (A) Plastic waste particles transformation into elongated shape retained on sieves size (5–3.35 mm); (B) cluster of plastic waste particles and fine aggregate retained on sieves size (1.18 mm).
According to the gradation curve diagram shown in Figure 4, the Malaysian Public Works Department has specified the percentages of aggregate for each sieve size and calculated the retained aggregate mass according to the percentage passing through at each sieve size. In addition, the aggregate grading for AC14 conformed to the gradation limit, being precisely centered between the upper and lower limits. Figure 4 also depicts the combined aggregate gradation for the PMIX-C and PMIX-E. The figure clearly showed that the combined aggregate for PMIX-C with 0.5 and 1% plastic content has deviated under the lower limit specified by the Malaysian Public Works Department standard (2008). This change in mixture gradation can lead to a decrease in the performance of the asphalt mixtures. The change in the size of aggregate due to the presence of fragile plastic particles reduces the mixture’s ability to withstand deformation under loading.
In addition, for PMIX-E, the sieve size of 14 and 5 mm increased slightly by about 15 and 8%, respectively, whereas that of 5 and 3.35 mm decreased by 2 and 9%, respectively. The deviation in gradation was due to the adhesion of small and large particles of aggregate. The deviation in PMIX-E was temporary because adding the asphalt directly to the modified aggregate caused the adhered particles to separate. As shown in Figure 5, after applying the waste plastic, the modified aggregate reveals a shine surface due to the formation of a plastic layer around the aggregate particles.
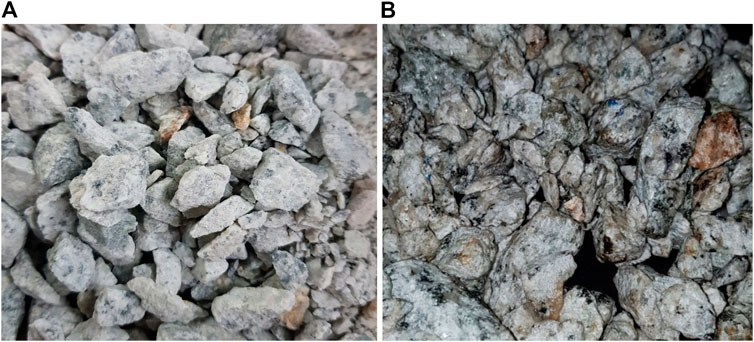
FIGURE 5. Aggregate. (A) Aggregate before modification; (B) Optimal plastic-coated aggregate (shine surface).
Marshall Stability and the Volumetric Properties
The Marshall stability and volumetric properties results depicted in Table 3 showed that the mixtures that incorporated 1% plastic waste had higher stability values by approximately 18% compared with the control mixture. The increase in stability value was caused by the high stiffness of plastic at high temperatures (60°C) as the softening point of plastic was higher than asphalt (Huang et al., 2000). High softening point of waste plastic contributes in increasing the shear force and reduce the fluidity for the part of the binder interacted with plastic coat (Ahmadinia et al., 2011; Yin and Wu, 2018). The stability of the asphalt mixture was affected by the adhesion of the asphalt binder with the plastic-modified aggregate and the cohesion of the asphalt binder itself. Therefore, having high stability values for PMIX-E seems logical as the presence of melted plastic around the aggregate surface was found to improve the adhesion properties of the asphalt binder with the aggregate, as revealed by (Khurshid et al., 2019).
The volumetric properties of plastic waste-modified mix serve as a performance indicator to detect improper gradients or mixing temperature. The result clearly shows that the variation associated with the dry process of adding plastic waste is primarily due to mixing control problems. Table 3 shows the effect of incorporating plastic waste on the volumetric properties of the asphalt mixtures. Moreover, the control mixture showed expected air void content of 3.4%. Modified mixtures, PMIX-C and PMIX-E showed an increase in the air void content by about 25%. However, the values of air voids for all mixtures were within the limit specified in the specification (3–5%). As the content of plastic waste in the asphalt mixtures increases, the content of air void increased. This case can be justified for PMIX-E, where the presence of the melted coating of plastic waste surrounding the aggregate particles can prevent the polymer from filling the voids in the mineral aggregate. Moreover, the interaction between the melted layer of plastic and asphalt binder can increase the viscosity and stiffness of the asphalt binder, thereby consequently decreasing the workability of the modified mixtures. Knowing that the decrease in workability leads to an increase in the compaction effort required for reaching the target air void content (Radhi, 2015). For PMIX-C, the results of air void for PMIX-C increase with the increase in plastic content due to a decrease in the workability behavioral of mixtures during the compaction (Movilla-Quesada et al., 2019b). The decrease in workability is greater when a high plastic content was used in the asphalt mixtures. Moreover, the higher affinity of plastic waste to adhere to the fine aggregate and forming clusters of plastic particles and fine aggregate can reduce the homogeneity and workability of mixtures. The flow values are mainly related to the value of air void content of asphalt mixtures, where a higher air void content produces mixtures with a high tendency to deform under loading (Moghaddam et al., 2013; Radeef et al., 2021a; Radeef et al., 2021b; Abdul Hassan et al., 2019). The flow increased slightly with the increment in the plastic content. The flow value of PMIX-C with 0.5 and 1% plastic content has increased by 5 and 16%, respectively, whereas the flow value has increased by 1.7 and 7% for PMIX-E with 0.5 and 1% plastic content, respectively. The increase in the flow value of PMIX-C and PMIX-E can be attributed to a high air void content for PMIX-C mixtures or to extra asphalt content in the mixture due to the presence of plastic-coated aggregate for PMIX-E. Extra asphalt content leads to an increase in softening behavior of the asphalt mixtures. The VFA are decreased by increasing the plastic content for PMIX-C. An increase in the plastic content produces more plastic particles, thereby increasing the surface area to be covered with asphalt. Moreover, the VFA for PMIX-E decreased slightly with the increase of the plastic content compared with the control mixture. This behavior was acceptable because the effect of the plastic content in PMIX-E was particularly limited to a low surface area of aggregate (coarse aggregate). In addition, as the plastic content increases, more aggregate surface will be covered with plastic coating. Such results agree with other studies that have investigated the impact of plastic waste on the volumetric properties of asphalt mixtures (Movilla-quesada et al., 2019b).
Moreover, the table presents the stiffness values of the control and modified mixtures. The stiffness of mixtures with 0.5 and 1% plastic waste material (PMIX-E) increased by 7 and 12%, respectively (Ahmadinia et al., 2012; Modarres and Hamedi, 2014; Yin and Wu, 2018). The highest values were obtained with a 1% plastic content for both modified mixtures. PMIX-E was found to produce greater stiffness values for both plastic contents followed by the control and PMIX-C, respectively. Although PMIX-C presented high stability values, the stiffness value was lower due to the high flow recorded during the stability test. These results are concurrent with the findings obtained by previous studies.
Performance Evaluation
The performance tests were conducted on the control mixture and the highest plastic waste content (1%) mixtures of PMIX-C and PMIX-E mixing methods. This procedure was conducted because the modified mixtures with a 1% plastic content have passed the standard requirement of the volumetric properties whilst containing the highest plastic waste content, which is preferred for upcycling more plastic waste.
Resilient Modulus
Figure 6 shows the results of the MR test at 25 and 40°C. As shown in the figure, the MR of PMIX-E was slightly higher than PMIX-C and the control mixture by 2 and 20%, respectively. This may be due to the high adhesion of asphalt with coated aggregated, which can also contribute to the asphalt film modified by the melted plastic on the aggregate surface. Moreover, plastic coatings on the aggregate might potentially contribute as damping layers for the applied stress, which leads to the absorption of more deformation energy into elastic deformation rather than plastic deformation. These observations were in agreement with those of Vila-Cortavitarte et al. (2019), Movilla-Quesada et al. (2019b). Temperature was found to significantly influence the resilient modulus of asphalt mixtures. The difference in MR was more prominent as the temperature increases, with a decline in stiffness at 40°C. For each mixture, the MR at 40°C is lower than that at 25°C by about 60%. Such behavior are typically related to the thermal properties of asphalt mixtures. The higher the temperature of the asphalt mixture, the lower the resilient modulus values. On the other hand, the lower the temperature imposed on the asphalt mixture, the higher the resilient modulus values. Nevertheless, a similar trend was observed, such that the highest MR was 1,257 MPa for PMIX-E, whereas the lowest was for PMIX-C. These results can be justified based on the role of plastic in the asphalt mixture. For PMIX-E, the high softening value of plastic can contribute to increasing the strength of mixtures under the load. However, for PMIX-C, softening of asphalt binder due to the high testing temperature can increase the relative aggregate movement, consequently, disintegrate the adhered particles of plastic and fine aggregate leading to more permanent deformation in the asphalt mixtures. At high temperatures, the asphalt mixtures sustain most of the applied compression load by the interlocking force between mixtures component rather than the adhesive and adhesion force.
Moisture Damage
The presence of moisture in asphalt pavements causes a reduction in stiffness, strength and durability. A higher tensile strength ensures that the asphalt pavement can withstand higher stresses before experiencing failure. In addition, the susceptibility to moisture can be measured in terms of the TSR by comparing the tensile strength of asphalt mixtures after exposure to wet conditions (ITS-Wet) with those of dry conditions (ITS-Dry). Figure 7 illustrates the results of the ITS test for unconditioned and wet-conditioned mixtures. Generally, the ITS values of the dry condition at the end of the loading test were higher for PMIX-E and the control mixture compared with the wet condition. Similar findings are discovered by previous researches, which indicates the decrease in ITS was attributed to the loss of adhesion of the mixture and/or loss of cohesion of the binder (Aguiar-Moya et al., 2015; Kakar et al., 2017). However, PMIX-E displayed a higher value of TSR and ITS in dry and wet conditions by about 6, 8, and 12%, respectively. This observation indicated that PMIX-E was less susceptible to moisture damage due to the influence of the plastic coat on the aggregate surface, where the plastic coat prevented the diffusion of water between the aggregate and the asphalt binder. Notably, these modified mixtures have higher porosity than the control mixture that will easily permit the water to access the internal structure and increase the potential for stripping. However, the effect of plastic coating on aggregate (in PMIX-E) is better than the plastic formation as extra particles (in PMIX-C) in the asphalt mixtures. The adhered plastic on aggregate particles can reduce the binder-aggregate interface that resulted in a reduction in stripping. Moreover, de-bonding may not have been exclusively responsible for the decline in tensile strength values, where moisture-damaging factors such as asphalt matrix softening may also have been responsible for the decline in tensile strength (Kamaruddin et al., 2016). Thus, for PMIX-E mixtures, the modified asphalt around the aggregate can reduce the softening behavior of the conventional asphalt binder due to moisture conditions. The plastic coat thereby increases the stiffness of asphalt binder around the aggregate, which increases the interlocking force between aggregate particles. High interlocking increases the tensile strength and the cracking resistance of the modified asphalt mixtures (Modarres and Hamedi, 2014; Aliha et al., 2015; Movilla-Quesada et al., 2019b). In summary, failure in asphalt mixtures is mainly initiated and propagated around the coarse aggregate of the mixtures (Xu et al., 2020; Radeef et al., 2021b). Thus, the presence of the plastic coat with modified asphalt can lead to a decrease in the rate of cracking propagated throughout.
For PMIX-C, the presence of non-molten particles of plastic waste increases the air void content, which leads to a less satisfactory response to moisture action. Moreover, extra particles can increase the available area to be covered by the asphalt film, thereby leading to improper asphalt aggregate covering. Moreover, a high air void content infiltrates the water into the macropores and washes away of the asphalt binder film that coated the aggregate. According to AASHTO T283 (AASHTO T283, 2014) standard specification, asphalt mixtures with TSR less than 80% have a low resistance to moisture damage. Such results are observed for mixtures prepared using the conventional dry method PMIX-C (78%). For further investigation, ImageJ was used to quantify the fracture surface of the control and modified mixtures in terms of other parameters selected in this study; the ratio of breakage aggregate (RBA) (yellow color zones in Figure 8) and the ratio of washed-out aggregate (RWA) (green color zones). The images were analyzed using the threshold color method. The threshold color allows image segmentation based on the histological RGB, saturation and brightness settings. This option allows for image analysis based on the number of pixels (px) or pre-defined scale in the imaging software. Figure 8 displays the impact of moisture conditioning on the fracture surface of the modified and control mixtures. PMIX-E presents the lowest RWA followed by the control mixtures, whereas PMIX-C showed the highest RWA by increasing the RWA by about 25%. This finding indicates that the film asphalt on the aggregate was significantly washed out due to water diffusion into the sample. The aggregate washed away by moisture conditioning is indicated in the green color zones. Moreover, the PMIX-E presented the highest RBA followed by the control and PMIX-C, respectively. The ratio of breakage area and washed-out aggregate can refer to high bonding and cohesive properties between the aggregate and binder (Aguiar-Moya et al., 2015; Hamzah et al., 2017).
Dynamic Creep
The excellent performance of the asphalt mixture to resist permanent deformation was identified by the creep stiffness. Figures 9, 10 depict the results of the permanent strain, dynamic creep stiffness and creep strain slope (CSS) tested at 40°C. The results show that PMIX-E provided better results than the control and PMIX-C mixtures. Figure 9 presents the permanent strain vs. the number of cycles. From the graph, PMIX-E exhibited a low permanent strain (3650 µε) compared with the control (5120 µε) and PMIX-C (5315 µε) mixtures. This result indicated that PMIX-E has a high resistance to deformation under repeated loads at high-temperature conditions, whereas the permanent strain represents the amount of plastic deformation of asphalt mixtures, which mainly take place in the mastic. In addition, the resistance to permanent deformation can contribute to the effect of plastic waste on increasing the softening point of the asphalt binder. This case can promote high resistance to deformation at high-temperature testing. Moreover, incorporating plastic waste by the conventional dry method can have a reversible effect on the mixture’s resistance specifically under repeated loading. The weak bonding of the cluster particles generated due to adhering plastic particles with fine aggregate can easily crush under loading. The same behavior was observed in the dynamic indirect resilient modulus of PMIX-C. The plastic particles that are not melted can decrease mixtures’ bonding properties. Then, non-molten plastic particles have a low affinity to construct bonding with aggregate and asphalt binder. A different trend was observed for the results of the creep strain slope presented in Figure 10. The result demonstrated that plastic waste can decrease the deformation rate of the asphalt mixtures, and both PMIX-C and PMIX-E present a low creep strain slope by about 13 and 24%, respectively, which indicates high resistance to permanent deformation. The creep strain slope can indicate the behavior of materials to resist the permanent deformation in the second third of the permanent strain curve. Thus, the materials can have an initial deformation at the first third and simultaneously have a low deformation rate at the second. This case was clearly observed in the behavior of the PMIX-C mixtures. Moreover, the values of creep stiffness showed a similar trend to the value of permanent strain results. The values of the creep strain slope indicated that PMIX-E had higher mixture stiffness compared with the control and PMIX-C mixtures. The result of the dynamic creep test was in good agreement with the MR and TSR test values (Angelone et al., 2016).
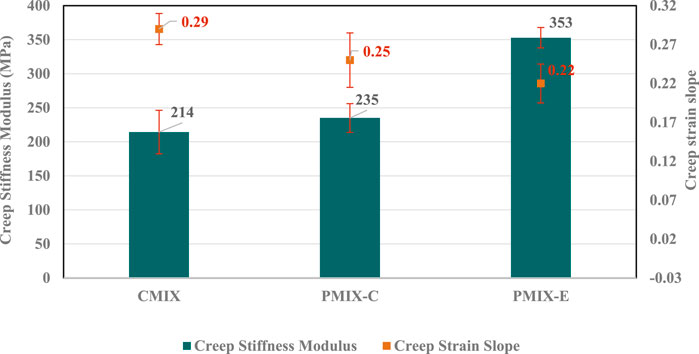
FIGURE 10. Values of creep stiffness modulus and creep strain slope of the control, PMIX-C and PMIX-E mixtures.
Rutting Resistance
The severity of permeant deformation in asphalt mixtures is primarily due to the thermal viscoelastic-plastic behavior of asphalt mixture. As a result, increasing the test temperature or loading cycle speeds up the permeant deformation of asphalt mixtures. The permeant deformation induced during the rutting test is a function of the loading cycle, rate of loading, and temperature, and is related to the asphalt binder’s role in the asphalt mixture. Figure 11 reveals the development of rut depths for the control and modified mixtures. As observed, the rut depth increased with increment in passes. The maximum rut depth for the control was 2.33 mm after 10,000 cycle passes. The inclusion of plastic waste into the mixtures had enhanced the rutting resistance for both methods of mixing, whereby PMIX-E presented a maximum rut depth value of 0.92 mm, followed by PMIX-C (1.97 mm) and control mixtures (2.3 mm). The maximum rut depth of the modified mixtures has decreased by about 60 and 15% for PMIX-E and PMIX-C, respectively. This result can be contributed to the effect of plastic waste on decreasing the vital impact of asphalt at a high temperature of 50°C. At this temperature, the permanent deformation was reduced as the plastic is still in a rigid form. The same behavior was demonstrated for permanent creep and stability tests. Moreover, this case can be due to the enhancement in adhesive and adhesion properties, which can increase the mechanical friction between asphalt mixture’s components, and consequently, the enhancement of bonding between asphalt and aggregate. The high adhesion between binder and aggregate has a significant contribution in increasing the resistance of materials to rutting. Rutting deformation was declared to be caused by a failure of mastic or loss of bond between aggregate particles and the asphalt binder. In both cases, the asphalt binder plays a substantial role in increasing the ability of asphalt mixture to resist rutting deformation. The failure of mastic was observed in the cross-section of the tested samples under the wheel path as shown in Figure 12. The images were processed using ImageJ software. The red color refers to the cracks and voids generated in the mastic after 10,000 cycles. The findings obtained from the wheel tracking test are in agreement with the outcomes retrieved from the MR test (Resilient Modulus), whereby PMIX-E has the lowest permanent deformation under the dynamic loads. The results were also in agreement with the outcomes reported by Khurshid et al. (2019), which refer to a decrement in rutting depth after adding the waste plastic.
Conclusion
From the analysis, it can be concluded that the aggregate gradation was highly affected by the method of adding plastic waste. An inappropriate adding procedure reduced the optimistic effect of plastic waste modification on the asphalt mixtures, which led to low-performance mixtures. The enhanced method proposed in this study showed significant improvement in the performance of modified asphalt mixtures. Adding part of the asphalt content after mixing the aggregate with plastic had a significant impact on the performance of the produced mixture. The results showed improvement in moisture damage, tensile strength and permanent deformation. This result can be contributed to the effect of hot aggregate on boosting the digestion process of plastic and asphalt coating the aggregate particles. PMIX-C showed a high deviation in the gradation for all sieves sizes by approximately 20%, whereas the PMIX-E effect was limited to the coarse aggregate. Moreover, the PMIX-E effect was temporary as the aggregate returned to its normal gradation after mixing with the asphalt binder in the second step (as shown in Figure 2). The volumetric properties of PMIX-C were comparable to PMIX-E with several important differences that reflected the role of plastic distribution in each mixture. Both methods showed increased flow and air voids, which is due to the decrease in the workability of plastic-modified mixtures. The plastic coat for PMIX-E helped in clogging the voids at the aggregate surface and reducing the VFA. Asphalt mixtures modified with 1% waste plastic using the enhanced dry method showed significant enhancement in the performance properties. The PMIX-E outperformed PMIX-C and the control mixtures. PMIX-E showed low susceptibility to moisture damage and high resistance to elastic and permanent deformation. The plastic layer-coated aggregate surface prevented water penetration between the asphalt and aggregate and consequently reduced the stripping of aggregate. The modified asphalt around the coated aggregate enhanced the adhesive and cohesive properties of the mixtures, which lead to an improvement in the resistance to permeant strain of the produced mixtures up to 35%. Under the dynamic loads, incorporating plastic waste using the modified method enhances the rutting resistance of the control mixture up to 60%. Therefore, the results of the laboratory testing confirm that the improved method of the dry process produces a better-modified asphalt mixture containing plastic waste.
Data Availability Statement
The original contributions presented in the study are included in the article/Supplementary Material, further inquiries can be directed to the corresponding author.
Author Contributions
The authors contributed to the present study as follows: HR and NH: methodology; HR, NH, AA, MM, and NY: validation; HR: formal analysis, NH, MM, NY, and AA: investigation and editing; HR: resources, software, and visualization; HR and NH: data curation; NH, AA, MM, MS, and MW: supervision. All authors have read and agreed to the published version of the manuscript.
Funding
This work was supported/funded by the Ministry of Higher Education (MOHE) under Fundamental Research Grant Scheme (FRGS) FRGS/1/2019/TK01/UTM/02/6 and the Universiti Teknologi Malaysia for UTMShine grant, Q.J130000.2451.09G26.
Conflict of Interest
The authors declare that the research was conducted in the absence of any commercial or financial relationships that could be construed as a potential conflict of interest.
References
AASHTO T283 (2014). Standard Method of Test for Resistance of Compacted Bituminous Mixture against to Moisture Induced Damage. Washington, DC: Am. Assoc. State Highw. Transp. Off.
Abdul Hassan, N., Abdulhussein Abdulridha Almusawi, A., Zul Hanif Mahmud, M., Asniza Mohamed Abdullah, N., Athma Mohd Shukry, N., Mashros, N., et al. (2019). Engineering Properties of Crumb Rubber Modified Dense-Graded Asphalt Mixtures Using Dry Process. IOP Conf. Ser. Earth Environ. Sci. 220, 012009. doi:10.1088/1755-1315/220/1/012009
Aguiar-Moya, J. P., Salazar-Delgado, J., Baldi-Sevilla, A., Leiva-Villacorta, F., and Loria-Salazar, L. (2015). Effect of Aging on Adhesion Properties of Asphalt Mixtures with the Use of Bitumen Bond Strength and Surface Energy Measurement Tests. Transportation Res. Rec. 2505, 57–65. doi:10.3141/2505-08
Ahmadinia, E., Zargar, M., Karim, M. R., Abdelaziz, M., and Ahmadinia, E. (2012). Performance Evaluation of Utilization of Waste Polyethylene Terephthalate (PET) in Stone mastic Asphalt. Construction Building Mater. 36, 984–989. doi:10.1016/j.conbuildmat.2012.06.015
Ahmadinia, E., Zargar, M., Karim, M. R., Abdelaziz, M., and Shafigh, P. (2011). Using Waste Plastic Bottles as Additive for Stone mastic Asphalt. Mater. Des. 32, 4844–4849. doi:10.1016/j.matdes.2011.06.016
Al-Adham, K., and Al-Abdul Wahhab, H. (2018). Influence of Temperature on Jnr Values of Polymer Modified Asphalt Binders. Int. J. Pavement Res. Tech. 11, 603–610. doi:10.1016/j.ijprt.2018.01.001
Aliha, M. R. M., Bahmani, A., and Akhondi, S. (2015). Numerical Analysis of a New Mixed Mode I/III Fracture Test Specimen. Eng. Fracture Mech. 134, 95–110. doi:10.1016/j.engfracmech.2014.12.010
Angelone, S., Cauhapé Casaux, M., Borghi, M., and Martinez, F. O. (2016). Green Pavements: Reuse of Plastic Waste in Asphalt Mixtures. Mater. Struct. 49, 1655–1665. doi:10.1617/s11527-015-0602-x
Anochie-Boateng, J., Komba, J., and Tutumluer, E. (2011). 3D Laser Based Measurement of mineral Aggregate Surface Area for South African Hot-Mix Asphalt Mixtures. Washington, DC: Transportation Research Board.
ASTM D1559-89 (1989). Test Method for Resistance of Plastic Flow of Bituminous Mixtures Using Marshall. West Conshohocken, PA: ASTM International.
ASTM D2041/D2041M-11 (2011). Standard Test Method for Theoretical Maximum Specific Gravity and Density of Bituminous Paving Mixtures. West Conshohocken, PA: ASTM International.
ASTM D4123-82 (1995). Standard Test Method for Indirect Tension Test for Resilient Modulus of Bituminous Mixtures. Am. Soc. Test. Mater. doi:10.1520/D4123-82R95
ASTM D4867 (2014). Standard Test Method for Effect of Moisture on Asphalt Concrete Paving Mixtures. West Conshohocken PA: ASTM Int.
Attaelmanan, M., Feng, C. P., and Ai, A.-H. (2011). Laboratory Evaluation of HMA with High Density Polyethylene as a Modifier. Construction Building Mater. 25, 2764–2770. doi:10.1016/j.conbuildmat.2010.12.037
Bera, A., and Babadagli, T. (2015). Status of Electromagnetic Heating for Enhanced Heavy Oil/bitumen Recovery and Future Prospects: A Review. Appl. Energ. 151, 206–226. doi:10.1016/j.apenergy.2015.04.031
Brasileiro, L., Moreno-Navarro, F., Tauste-Martínez, R., Matos, J., and Rubio-Gámezdel, M. C. (2019). Reclaimed Polymers as Asphalt Binder Modifiers for More Sustainable Roads: A Review. Sustainability 11, 1–20. doi:10.3390/su11030646
BS EN 12697-22 (2013). Bituminous Mixtures - Test Methods for Hot Mix Asphalt -. Part 22 Wheel Tracking. London, UK: BSI Stand. Institution.
BS EN 12697-25 (2013). Bituminous Mixtures - Test Methods for Hot Mix Asphalt-Part 25: Cyclic Compression Test. London, UK: BSI Stand. Inst.
Fernandes, S., Costa, L., Silva, H., and Oliveira, J. (2017). Effect of Incorporating Different Waste Materials in Bitumen. Ciência & Tecnologia dos Materiais 29, e204–e209. doi:10.1016/j.ctmat.2016.07.003
González, V., Martínez-Boza, F. J., Gallegos, C., Pérez-Lepe, A., and Páez, A. (2012). A Study into the Processing of Bitumen Modified with Tire Crumb Rubber and Polymeric Additives. Fuel Process. Tech. 95, 137–143. doi:10.1016/j.fuproc.2011.11.018
Hamzah, M. O., Teh, S. Y., Golchin, B., and Voskuilen, J. (2017). Use of Imaging Technique and Direct Tensile Test to Evaluate Moisture Damage Properties of Warm Mix Asphalt Using Response Surface Method. Construction Building Mater. 132, 323–334. doi:10.1016/j.conbuildmat.2016.11.092
Huang, Y., Bird, R. N., and Heidrich, O. (2007). A Review of the Use of Recycled Solid Waste Materials in Asphalt Pavements. Resour. Conservation Recycling 52, 58–73. doi:10.1016/j.resconrec.2007.02.002
Huang, Y., Bird, R. N., Heidrich, O., Kök, B. V., Çolak, H., Zoorob, S. E., et al. (2000). Utilization of Waste Nylon Wire in Stone Matrix Asphalt Mixtures. Waste Manag. 78, 233–242. doi:10.1016/S0958-9465(00)00026-3
Jabatan Kerja Raya Malaysia (JKR) (2008). Standard Specification for Road Works, Sect. 4 Flex. Pavement. No. JKR/SPJ/2008-S4 Kuala Lumpur: Ministry of Public Works, S4-58–S4-69.
Kakar, M. R., Hamzah, M. O., and Valentin, J. (2017). Analyzing the Stripping Potential of Warm Mix Asphalt Using Imaging Technique. IOP Conf. Ser. Mater. Sci. Eng. 236, 012013. doi:10.1088/1757-899X/236/1/012013
Kamaruddin, I., Napiah, M., and Nahi, M. H. (2016). The Influence of Moisture on the Performance of Polymer Fibre-Reinforced Asphalt Mixture. MATEC Web Conf. 78, 01040. doi:10.1051/matecconf/20167801040
Khurshid, M. B., Qureshi, N. A., Hussain, A., and Iqbal, M. J. (2019). Enhancement of Hot Mix Asphalt (HMA) Properties Using Waste Polymers. Arab. J. Sci. Eng. 44, 8239–8248. doi:10.1007/s13369-019-03748-3
Köfteci, S., Ahmedzade, P., and Kultayev, B. (2014). Performance Evaluation of Bitumen Modified by Various Types of Waste Plastics. Construction Building Mater. 73, 592–602. doi:10.1016/j.conbuildmat.2014.09.067
Lastra-González, P., Calzada-Pérez, M. A., Castro-Fresno, D., Vega-Zamanillo, Á., and Indacoechea-Vega, I. (2016). Comparative Analysis of the Performance of Asphalt Concretes Modified by Dry Way with Polymeric Waste. Construction Building Mater. 112, 1133–1140. doi:10.1016/j.conbuildmat.2016.02.156
Lo Presti, D. (2013). Recycled Tyre Rubber Modified Bitumens for Road Asphalt Mixtures: A Literature Review. Construction Building Mater. 49, 863–881. doi:10.1016/j.conbuildmat.2013.09.007
Mishra, B., and Gupta, M. K. (2020). Use of Plastic Waste in Bituminous Mixes by Wet and Dry Methods. Proc. Inst. Civil Eng. - Municipal Engineer 173, 87–97. doi:10.1680/jmuen.18.00014
Modarres, A., and Hamedi, H. (2014). Effect of Waste Plastic Bottles on the Stiffness and Fatigue Properties of Modified Asphalt Mixes. Mater. Des. 61, 8–15. doi:10.1016/j.matdes.2014.04.046
Moghaddam, T. B., Karim, M. R., and Soltani, M. (2013). Utilization of Waste Plastic Bottles in Asphalt Mixture. J. Eng. Sci. Technol. 8, 264–271.
Mohammad, L. N., Cooper, S. B., and Elseifi, M. A. (2011). Characterization of Hma Mixtures Containing High Reclaimed Asphalt Pavement Content with Crumb Rubber Additives. J. Mater. Civ. Eng. 23, 1–34. doi:10.1061/(ASCE)MT.1943-5533.0000359
Montanelli, E. F., and srl, I. (2013). Fiber/Polymeric Compound for High Modulus Polymer Modified Asphalt (PMA). Proced. - Soc. Behav. Sci. 104, 39–48. doi:10.1016/j.sbspro.2013.11.096
Movilla-Quesada, D., Raposeiras, A. C., and Olavarría, J. (2019a). Effects of Recycled Polyethylene Terephthalate (PET) on Stiffness of Hot Asphalt Mixtures. Adv. Civil Eng. 2019, 1–6. doi:10.1155/2019/6969826
Movilla-Quesada, D., Raposeiras, A. C., Silva-Klein, L. T., Lastra-González, P., and Castro-Fresno, D. (2019b). Use of Plastic Scrap in Asphalt Mixtures Added by Dry Method as a Partial Substitute for Bitumen. Waste Manag. 87, 751–760. doi:10.1016/j.wasman.2019.03.018
Oliviero Rossi, C., Spadafora, A., Teltayev, B., Izmailova, G., Amerbayev, Y., and Bortolotti, V. (2015). Polymer Modified Bitumen: Rheological Properties and Structural Characterization. Colloids Surf. A: Physicochemical Eng. Aspects 480, 390–397. doi:10.1016/j.colsurfa.2015.02.048
Polacco, G., Berlincioni, S., Biondi, D., Stastna, J., and Zanzotto, L. (2005). Asphalt Modification with Different Polyethylene-Based Polymers. Eur. Polym. J. 41, 2831–2844. doi:10.1016/j.eurpolymj.2005.05.034
Radeef, H. R., Abdul Hassan, N., Zainal Abidin, A. R., Mahmud, M. Z. H., Mohd Satar, M. K. I., Mohd Warid, M. N., et al. (2021a). Determining Fracture Energy in Asphalt Mixture: A Review. IOP Conf. Ser. Earth Environ. Sci. 682, 012069. doi:10.1088/1755-1315/682/1/012069
Radeef, H. R., Abdul Hassan, N., Zainal Abidin, A. R., Mahmud, M. Z. H., Yaacob, H., Mashros, N., et al. (2021b). Effect of Aging and Moisture Damage on the Cracking Resistance of Rubberized Asphalt Mixture. Mater. Today Proc. 42, 2853–2858. doi:10.1016/j.matpr.2020.12.734
Radhi, H. (2015). Effect of Lab . Compaction Effort on Evaluation of Modified HMA Specimens. Civ. Environ. Res. 7, 112–125.
Sasidharan, M., Torbaghan, M. E., and Burrow, M. (2019). Using Waste Plastics in Road Construction. Brighton, UK: Institute of Development Studies, 1–19.
Vila-Cortavitarte, M., Lastra-González, P., Calzada-Pérez, M. Á., and Indacoechea-Vega, I. (2019). The Use of Recycled Plastic as Partial Replacement of Bitumen in Asphalt concrete. Use Recycl. Plast. Eco-efficient Concr., 327–347. doi:10.1016/b978-0-08-102676-2.00015-3
Xu, W., Wei, X., Wei, J., and Chen, Z. (2020). Experimental Evaluation of the Influence of Aggregate Strength on the Flexural Cracking Behavior of Epoxy Asphalt Mixtures. Materials 13, 1876. doi:10.3390/ma13081876
Yin, J., and Wu, W. (2018). Utilization of Waste Nylon Wire in Stone Matrix Asphalt Mixtures. Waste Manag. 78, 948–954. doi:10.1016/j.wasman.2018.06.055
Yu, R., Fang, C., Liu, P., Liu, X., and Li, Y. (2015). Storage Stability and Rheological Properties of Asphalt Modified with Waste Packaging Polyethylene and Organic Montmorillonite. Appl. Clay Sci. 104, 1–7. doi:10.1016/j.clay.2014.11.033
Zak, J., Stastna, J., Vavricka, J., Milackova, K., Kasek, L., and Zanzotto, L. (2014). Poisson’s Ratio of Hot Asphalt Mixtures Determined by Relaxation and Small Amplitude Oscillation Tests. J. Test. Eval. 43, 49–58. doi:10.1061/9780784478462.007
Keywords: plastic waste, dry method, aggregate gradation, image analysis, performance
Citation: Radeef HR, Abdul Hassan N, Abidin ARZ, Mahmud MZH, Yusoffa NIM, Idham Mohd Satar MK and Warid MNM (2021) Enhanced Dry Process Method for Modified Asphalt Containing Plastic Waste. Front. Mater. 8:700231. doi: 10.3389/fmats.2021.700231
Received: 25 April 2021; Accepted: 21 June 2021;
Published: 09 July 2021.
Edited by:
Hui Yao, Beijing University of Technology, ChinaReviewed by:
Mohamad Yusri Aman, Universiti Tun Hussein Onn Malaysia, MalaysiaAli Behnood, Purdue University, United States
Copyright © 2021 Radeef, Abdul Hassan, Abidin, Mahmud, Yusoffa, Idham Mohd Satar and Warid. This is an open-access article distributed under the terms of the Creative Commons Attribution License (CC BY). The use, distribution or reproduction in other forums is permitted, provided the original author(s) and the copyright owner(s) are credited and that the original publication in this journal is cited, in accordance with accepted academic practice. No use, distribution or reproduction is permitted which does not comply with these terms.
*Correspondence: Norhidayah Abdul Hassan, aG5vcmhpZGF5YWhAdXRtLm15