- 1College of Chemical and Material Engineering, Quzhou University, Quzhou, China
- 2Laboratory of Polymer Materials and Engineering, NingboTech University, Ningbo, China
- 3MOE Key Laboratory of Macromolecular Synthesis and Functionalization, Department of Polymer Science and Engineering, Zhejiang University, Hangzhou, China
- 4Ningbo Miruo Electronic Technology Co., Ltd, Ningbo, China
It has been significant yet challenging to recycle and reuse different kinds of wastes because of their mass production within society. Many efforts have been conducted to reuse wastes in different fields. Interestingly, some wastes have been employed to replace traditional petroleum-based flame retardants for polymeric materials. This review focuses on the recent development of waste flame retardants and their impacts on thermal stability, flame retardancy, and smoke suppression of polymers, followed by representative flame-retardant mechanisms. Finally, the key challenges associated with waste flame retardants are presented, and some future perspectives are proposed.
Introduction
With the development of our society, abundant wastes have been continuously generated in daily life and industrial production, which have led to serious environmental deterioration problems, for example, marine microplastics and global warming. Such issues have driven scientists and engineers to explore how to effectively change waste into wealth. Hence, recent years have witnessed the recycle and reuse of different kinds of wastes in various industrial areas (Kabirifar et al., 2020; Zhang et al., 2020).
The superior chemical resistance, and thermal and mechanical properties of polymeric materials have meant they have ubiquitous applications in modern society (Chen et al., 2017; Liu and Wang, 2018; Wang et al., 2020b). However, most of them are highly flammable and produce abundant smoke during combustion, which severely threatens humans and the environment; thus, it is necessary to enhance the fire safety of polymers via adding flame retardants (Huo et al., 2020; Xue et al., 2020; Yang et al., 2021a). In the past, flame retardants are mainly derived from petroleum resources, which worsen the depletion of fossil resources (Wang et al., 2019). Based on the sustainable development strategy, many wastes have been reused to replace traditional flame retardants recently, and many important outcomes have been reported, demonstrating that the application of some wastes is an effective way to prepare flame-retardant polymeric materials (Li et al., 2019). For instance, Qian et al. (2015) used Bayer red mud to synthesize Mg/Al/Fe-layered double hydroxides (Mg/Al/Fe-LDHs), which were applied in the preparation of flame-retardant ethylene-vinyl acetate (EVA). Their results showed that the limiting oxygen index of the as-prepared EVA can be up to 26.8%. Das et al. (2016) reported a flame-retardant polypropylene (PP) with ammonium polyphosphate (APP), waste-based biochar, and wool. The results showed that both biochar and wool not only improved the flame retardancy of PP but also retained the mechanical properties. Hence, many waste-based flame retardants exhibit obvious flame retardant function towards polymeric materials.
Herein, this review focuses on the recent development of three kinds of common wastes (industrial, domestic, and plant; see Scheme 1) and highlights their influences on the thermal stability, flame retardancy, and smoke suppression of polymeric materials. Additionally, the flame-retardant mechanism of different wastes will be analyzed. Finally, their key challenges and future perspectives will also be indicated.
Industrial Waste
With the development of modern production, a constant stream of industrial waste is discharged into the environment, which has been harming the ecosystem and even human health severely. Thus, the reuse of industrial waste has become a hot topic in both academia and industry (Huo et al., 2021). Fortunately, many types of industrial wastes, for example, fly ash, oil sludge, and red mud, contain different valuable flame-retardant ingredients, such as thermally stable SiO2, Al2O3, and Fe2O3, thus exhibiting huge potential in the fabrication of flame-retardant polymers (Cao et al., 2017; Zhang et al., 2019; Liu et al., 2020). For instance, Kusakli et al. (2020) fabricated a flame-retardant epoxy resin (ER), which contained ammonium tetrafluoroborate (ATFB), aluminum hydroxide (Al(OH)3), and red mud (RM). Obviously, the introduction of ATFB, Al(OH)3, and RM improves the charring capacity of ER (see Figure 1). During the burning process, RM effectively retards the combustion of epoxy matrix due to its abundant Fe, Al, and Si elements. Additionally, the combination of ATFB, Al(OH)3, and RM endows epoxy resin with improved flame retardancy, mechanical properties, and thermal stability (see Figure 2), for example, a limited oxygen index (LOI) of 29.0%, a tensile strength of 112 MPa, and a glass transition temperature (Tg) of 210°C. Jia et al. (2014) synthesized an Mg-Al-Fe ternary-layered double hydroxide (LDH) using red mud as a raw material, and the corresponding flame-retardant and thermally stable ethylene-vinyl acetate/layered double hydroxide/graphite powder (EVA/LDH/GP) composites were fabricated. The results show that the as-prepared EVA/LDH/GP exhibits 81.1 and 54.2% reductions in the peak of the heat release rate (PHRR) and the average of the heat release rate (AHRR), respectively, relative to the neat EVA, indicating significantly improved flame retardancy. The combination of LDH and GP contributes to the formation of compact char layers due to their condensed-phase effect, thus leading to the significantly reduced heat release and improved fire safety of EVA composites. Such results further verify that waste-based RM can be used to prepare flame-retardant LDH because of its abundant metallic elements, for example, Al, Ca, and Fe. Fly ash rich in Si/Al oxides is also recognized as one class of effective waste flame retardants. Nguyen (2020) reported the synthesis of stearic acid–modified fly ash and the preparation of the corresponding flame-retardant epoxy resins with various fly ash contents (see Figure 3). Twenty wt% of fly ash decreases the burning rate of epoxy thermoset to 16.78 mm/min and increases the LOI to 23.2% (see Figure 4), demonstrating the flame-retardant characteristic. In addition, Wang et al. (2020a) employed magnesium alloy scrap to enhance the fire resistance of intumescent fire-retardant polyacrylate-based coatings applicable to structural steel. Magnesium alloy scrap is capable of significantly increasing the char expansion degree and suppressing the heat transfer of intumescent coatings when exposed to high-temperature flame (see Figure 5). In detail, introducing 0.48 wt% scrap reduces the backside temperature of the coating from 310 to 171°C by 44.8%, with a 100.8% increase in char expansion degree. The highly intumescent char effectively suppresses the heat transfer and protects the underlying steel during combustion. Hence, the waste-based magnesium alloy scrap can be used as a synergist for the intumescent fire-retardant systems that comprise ammonium polyphosphate (APP), pentaerythritol (PER), and melamine (MEL). Similar reuse of waste for the fabrication of fire-resistant polymers had also been reported by Saba et al. (2019). Obviously, the flame-retardant element (e.g., Al, Mg, and Si)-rich industrial wastes can be applied in the preparation of flame-retardant polymeric materials. However, the compatibility between industrial wastes and polymeric materials is an unavoidable issue, which impacts the comprehensive properties of polymers, and thus significantly restricts the practical applications of industrial wastes. In addition, the efficiency of the industrial waste–based flame retardants is not high enough, and needs to be further increased.
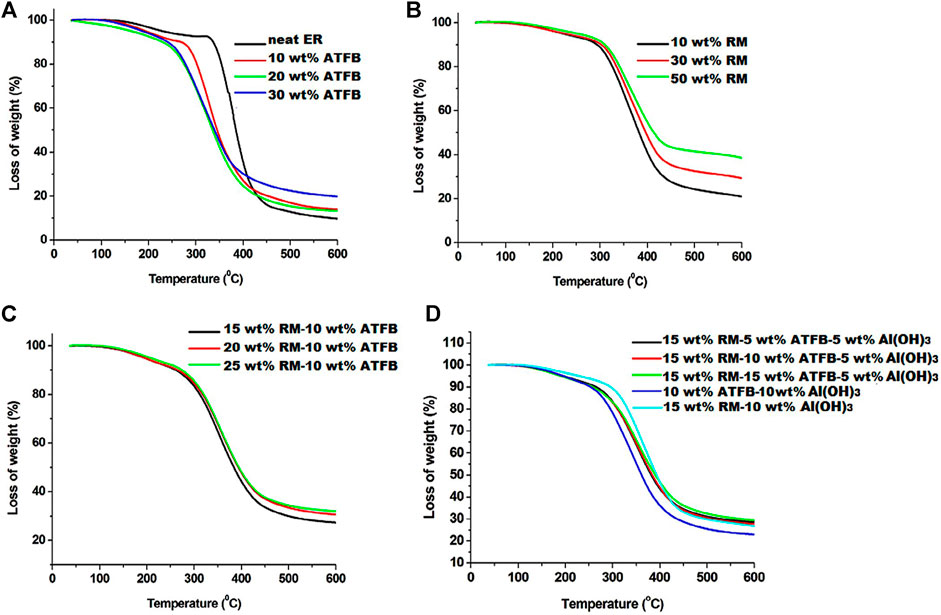
FIGURE 1. Thermogravimetric analysis (TGA) curves of (A) the neat ER and ER composites with 10–30 wt% ATFB, (B) ER composites with 10–50 wt% RM, (C) ER composites with 15–25 wt% RM and 10 wt% ATFB, and (D) ER composites with different contents of RM, ATFB, and Al(OH)3 (Kusakli et al., 2020).
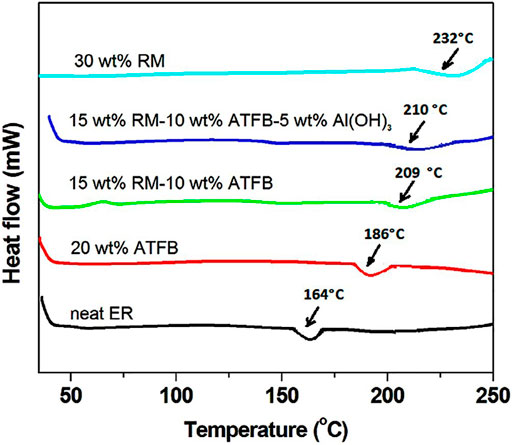
FIGURE 2. Differential scanning calorimeter (DSC) curves of the neat ER and ER composites with different contents of ATFB, RM, and Al(OH)3 (Kusakli et al., 2020).
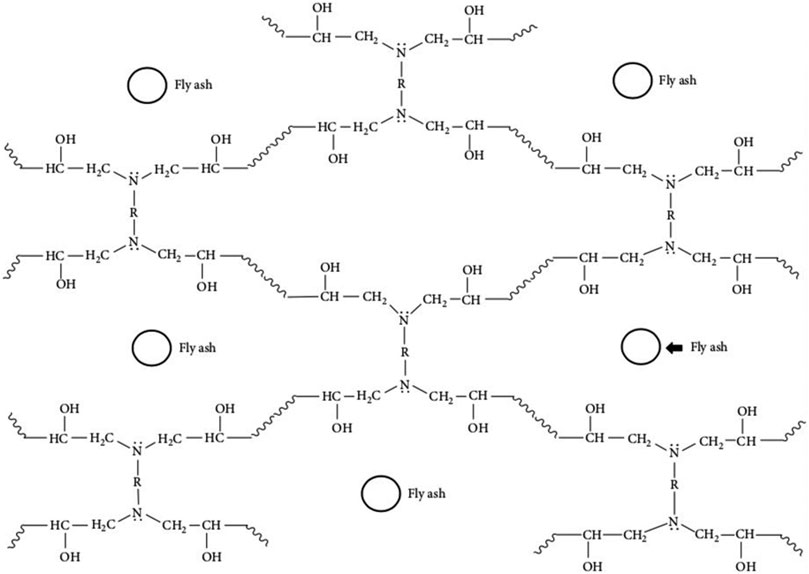
FIGURE 3. Schematic diagram of fly ash–containing epoxy networks after curing (Nguyen, 2020).
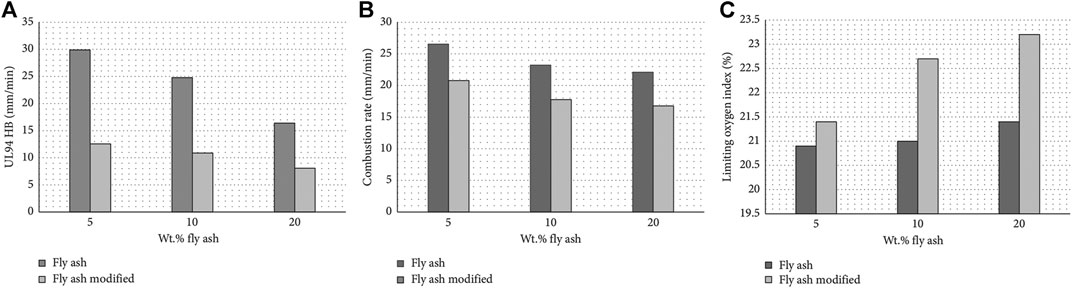
FIGURE 4. Fire resistance of epoxy resins containing fly ash or modified fly ash: (A) UL94 HB result, (B) combustion rate, and (C) LOI (Nguyen, 2020).
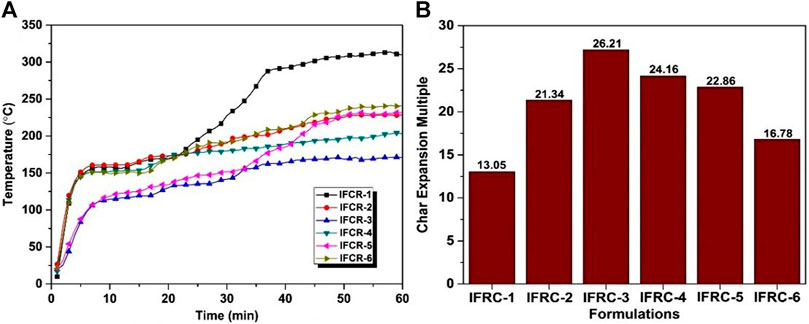
FIGURE 5. (A) Backside temperatures of coatings and (B) char expansion degrees of chars (Wang et al., 2020a).
Domestic Waste
Domestic waste comprises disposable materials generated by households in daily activities. Nowadays, only some domestic waste can be recycled, and the rest will go to landfills. However, the landfills will be completely filled if the level of waste continues to grow rapidly. To help ease this issue, the reuse of domestic waste has been a high priority in recent years. Interestingly, many kinds of domestic wastes have been explored to create flame-retardant polymers. For example, Xu et al. (2018) employed chicken eggshell (CES) to enhance the flame-retardant and smoke-suppressive performances of APP/PER/MEL-containing epoxy coatings (see Figure 6). The main component of CES is CaCO3, which is able to react with APP to generate calcium metaphosphate and calcium phosphate, and release inert gases, thus increasing the compactness and intumescent degree of residual char and diluting the combustible fragments and toxic smoke (see Figure 7). In detail, 3 wt% of CES decreases the peak heat release rate (PHRR) and total smoke release (TSR) by 42.2 and 15.0% in comparison to those of the APP/PER/MEL-containing epoxy system. Similarly, chicken eggshell has also been used as a flame-retardant synergist in poly (lactic acid) (PLA), ethylene-vinyl acetate copolymer (EVA), and acrylic resin (Yew et al., 2015; Oualha et al., 2019; Urtekin et al., 2020). Besides, Kong et al. (2020) intentionally collected banana peel powder that can serve as a char-forming agent, and then modified it with phytic acid to improve the flame-retardant performances (PA, see Figure 8). The resultant PA-modified powder (PA-B) imparts superior flame retardancy to PLA composite, of which the LOI value and UL-94 rating can reach up to 37.5% and V-0, respectively. Additionally, PA-B obviously reduces the heat release rate of PLA composite during combustion due to its condensed-phase flame-retardant effect. Thus, this work provides a scalable method to reuse the fruit peel in the fabrication of flame-retardant polymers. Recently, Chen et al. (2020) deposited six chitosan/APP bilayers on the surface of bagasse (BF) to prepare an environmentally benign flame retardant (6BL@BF) for epoxy resin. 6BL@BF reduces the PHRR and total heat release (THR) of epoxy thermoset by 64.6 and 13.2%, respectively, relative to those of the neat epoxy thermoset. Additionally, the incorporation of expandable graphite (EG) can further improve the fire safety of 6BL@BF-containing epoxy thermoset due to the cooperation of 6BL@BF and EG in the condensed phase. Shen et al. (2020) prepared a triglycidylisocyanurate (TGIC)/9,10-dihydro-9-oxa-10-phosphaphenanthrene-10-oxide (DOPO)–containing bagasse flame retardant (bagasse@TGIC@DOPO) for epoxy resins. It was found that the char yield of epoxy composite is increased from 14.1 wt% of the neat epoxy thermoset to 23.4 wt% with the incorporation of bagasse@TGIC@DOPO, indicating the positive effect of bagasse@TGIC@DOPO on the carbonization of epoxy matrix. Such an effect is conducive to the formation of compact char layers during the combustion of epoxy thermoset. Zabihi et al. (2020) used waste fish DNA as a surfactant to prepare a graphene-based flame retardant (GnPD). Notably, introducing only 10 wt% GnPD brings about ∼86, ∼80, and ∼61% increases in the LOI values of epoxy thermoset, poly (vinyl alcohol) (PVA), and polystyrene (PS), respectively. They also used waste fish DNA to modify the clay structure and fabricated high-performance flame-retardant epoxy resins based on this fish DNA–modified clay (Zabihi et al., 2016). Additionally, the waste-based flame-retardant epoxy coatings were prepared based on vegetable compounds (ginger powder and coffee husk), which were also used as carbon source of intumescent flame retardant system (Stéphanie et al., 2017). Both vegetable compounds can effectively enhance the flame-retardant performances of the intumescent flame retardant system. Obviously, most domestic wastes can be applied as carbon source for the intumescent flame-retardant system because of their OH-rich structure. All these works mentioned above pave a new route to reuse domestic wastes, which promotes the sustainable development of society and enhances the fire safety of polymers. In the future, how to prepare a high-efficiency intumescent flame-retardant system based on different domestic wastes is critical for the development of domestic waste–based flame retardants.
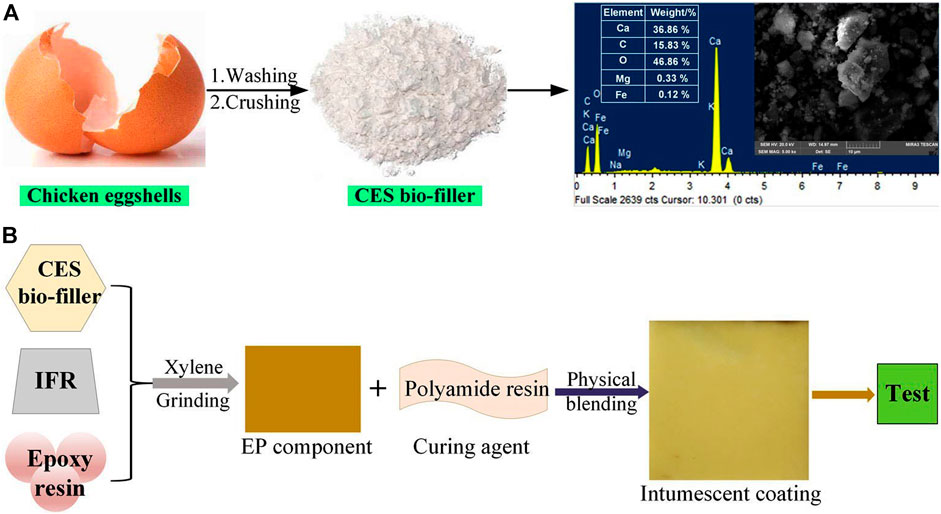
FIGURE 6. Illustration for (A) the preparation of CES bio-filler and (B) fabrication of CES-containing intumescent fire-retardant epoxy coatings (Xu et al., 2018).
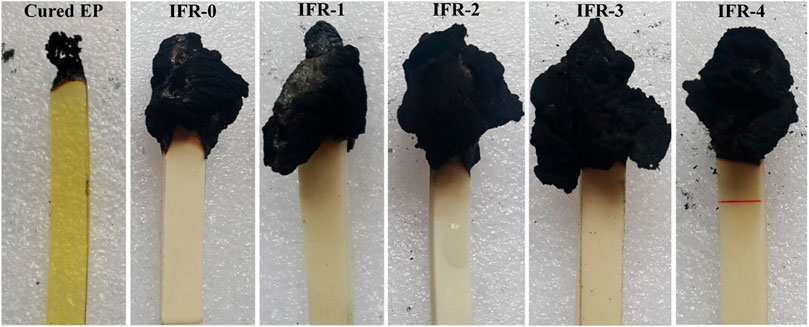
FIGURE 7. Digital photographs of the epoxy coatings after LOI tests (Xu et al., 2018).
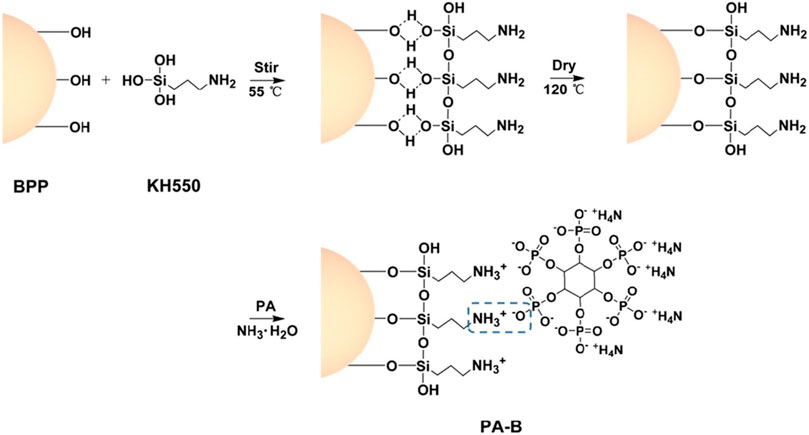
FIGURE 8. The fabrication of PA-B (Kong et al., 2020).
Plant Waste
There are two types of plant waste, cellulose nanofibers and lignin, that are often applied in the flame-retardant polymeric materials because of their renewable, biodegradable, and nontoxic characteristics (Zhang et al., 2015; Liu et al., 2016). Both cellulose nanofibers and lignin feature OH/C-rich structure, which can serve as charring agent during combustion, and their flame-retardant performances can be further enhanced via chemically introducing different flame-retardant groups, for example, P-, N-, and Si-containing ones (Feng et al., 2017; Yang et al., 2020; Yang et al., 2021b). Dai et al. (2020) synthesized P/N-containing lignin-based flame retardants (Lig-M and Lig-F, see Figure 9) by introducing piperazine and 9,10-dihydro-9-oxa-10-phosphaphenanthrene-10-oxide (DOPO) into lignin. The Lig-containing epoxy resin exhibits improved flame retardancy and smoke suppression, of which the UL-94 rating increases to V-0, and THR and the total smoke production (TSP) are reduced by 46.6 and 53%, respectively. The flame-retardant mechanism analysis indicates that the synergistic effect of lignin, nitrogen, and phosphorus is responsible for the improved flame retardancy and smoke suppression of epoxy thermoset. Yin et al. (2018) reported a flame-retardant additive (APP@CNF) obtained from the reaction between cellulose nanofibers (CNFs) and ammonium polyphosphate, and applied it in the fabrication of mechanically robust and flame-retardant PLA biocomposites (see Figure 10). With 5 wt% of APP@CNF, the PLA biocomposite features reduced the heat release rate (see Figure 11) and improved flame-retardant and mechanical properties, which passes a UL-94 V-0 rating, achieves a high LOI of 27.5%, and shows 54% increase in impact strength. The enhancements in flame retardancy and mechanical properties of PLA biocomposite are attributed to the improved dispersion of APP@CNF and the synergistic flame-retardant effect between APP and CNF. Recently, Chen et al. (2018) also synthesized a 2,6,7-trioxa-1-phosphabicyclo-[2,2,2]octane-4-methanol-1-oxide/triazine–modified lignin (CP-lignin) for polybutylene succinate (PBS), with the synthesis route shown in Figure 12. After incorporating 30 wt% of CP-lignin, the PHRR and THR of PBS composite are reduced by 27 and 31%, respectively, due to the promotion effect of CP-lignin on the carbonization of polymer matrix (see Figure 13). Ding et al. (2014) obviously enhanced the chemical activity of lignin by dripping the mixed solutions of diethanolamine and formaldehyde into the solution of lignin, and applied the modified lignin to prepare flame-retardant epoxy thermosets. Their results show that the introduction of the modified lignin brings about obvious enhancements in flame retardancy and thermal stability of epoxy thermoset. In detail, the decomposition temperature of lignin-based epoxy sample is 31°C higher than that of the neat epoxy sample. Xu et al. (2021) synthesized magnesium phytate (Mg-Phyt) and used it and rice husk ash as waste-based flame-retardants for epoxy resins. In comparison to a pure epoxy sample, the peak heat release rate, total heat release, peak smoke production rate, and total smoke release of the epoxy sample containing 5 wt% Mg-Phyt and 5 wt% rice husk ash are reduced remarkably by 28.6, 10.6, 33.0, and 7.8%, respectively. Therefore, both chemically introducing and physically combining flame-retardant groups are effective methods to improve the flame-retardant properties of lignin and cellulose nanofibers. However, lots of organic solvents are employed in the modification of lignin and cellulose nanofibers, which is not conducive to the sustainable development. In the future, more efforts should be made to create high-efficiency flame-retardant lignin and CNF via the green solvent or solvent-free method.
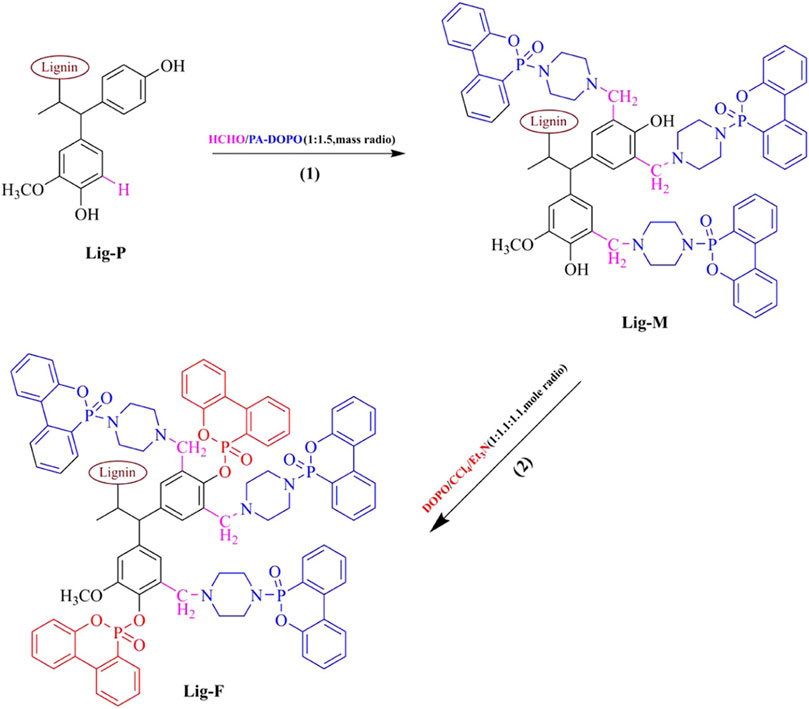
FIGURE 9. Synthesis of P/N-containing lignin (Lig-M and Lig-F) (Dai et al., 2020).
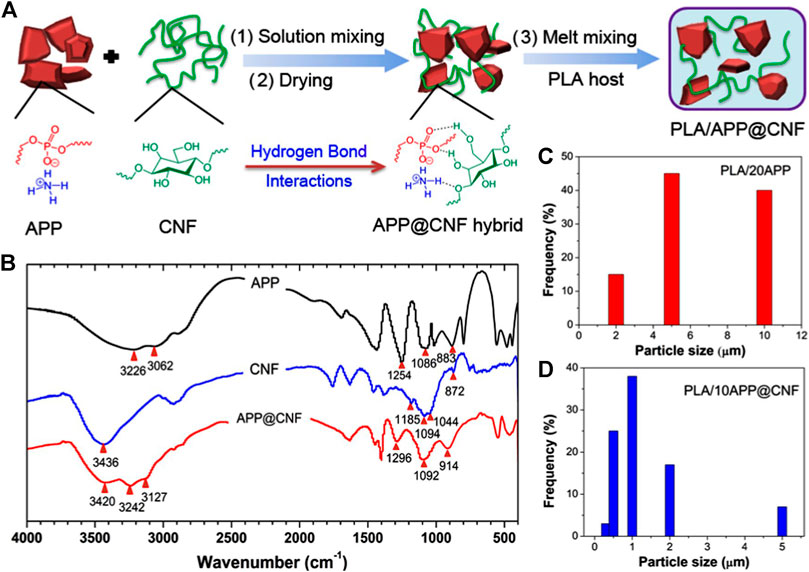
FIGURE 10. (A) The design and preparation of both APP@CNF and its PLA composite, (B) Fourier transform infrared spectroscopy (FTIR) spectra of APP, CNF, and APP@CNF, and the weight–average particle size distribution of (C) 20% APP and (D) 10% APP@CNF in the PLA matrix (Yin et al., 2018).
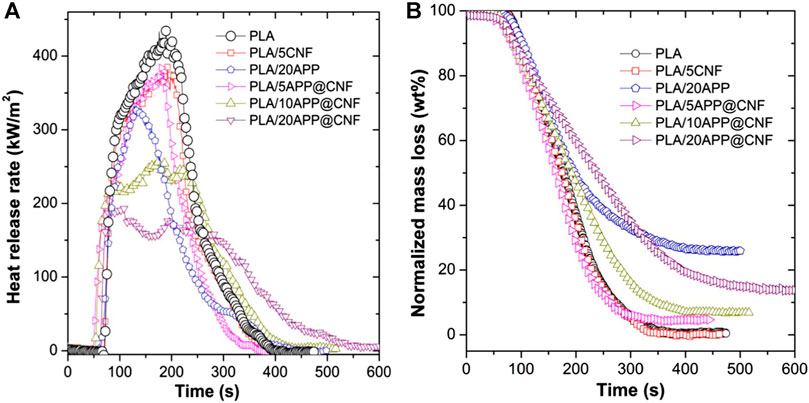
FIGURE 11. (A) HRR and (B) mass loss curves of PLA biocomposites obtained from cone calorimeter tests with an external heat flux of 35 kW/m2 (Yin et al., 2018).
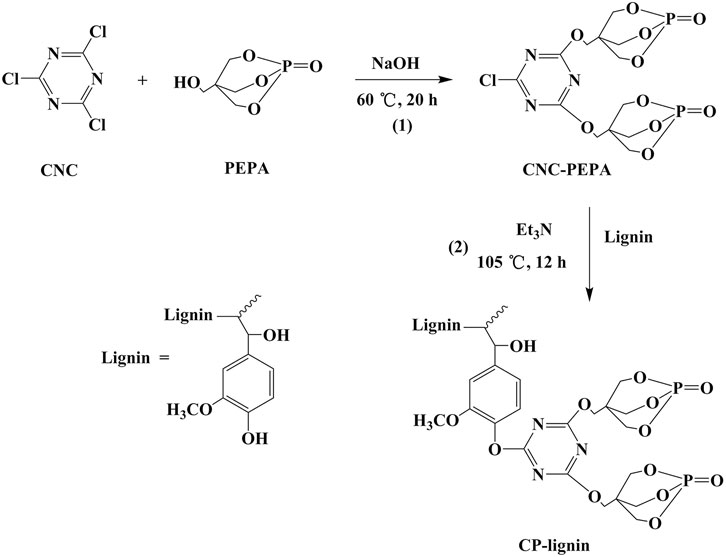
FIGURE 12. Synthesis of CP-lignin (Chen et al., 2018).
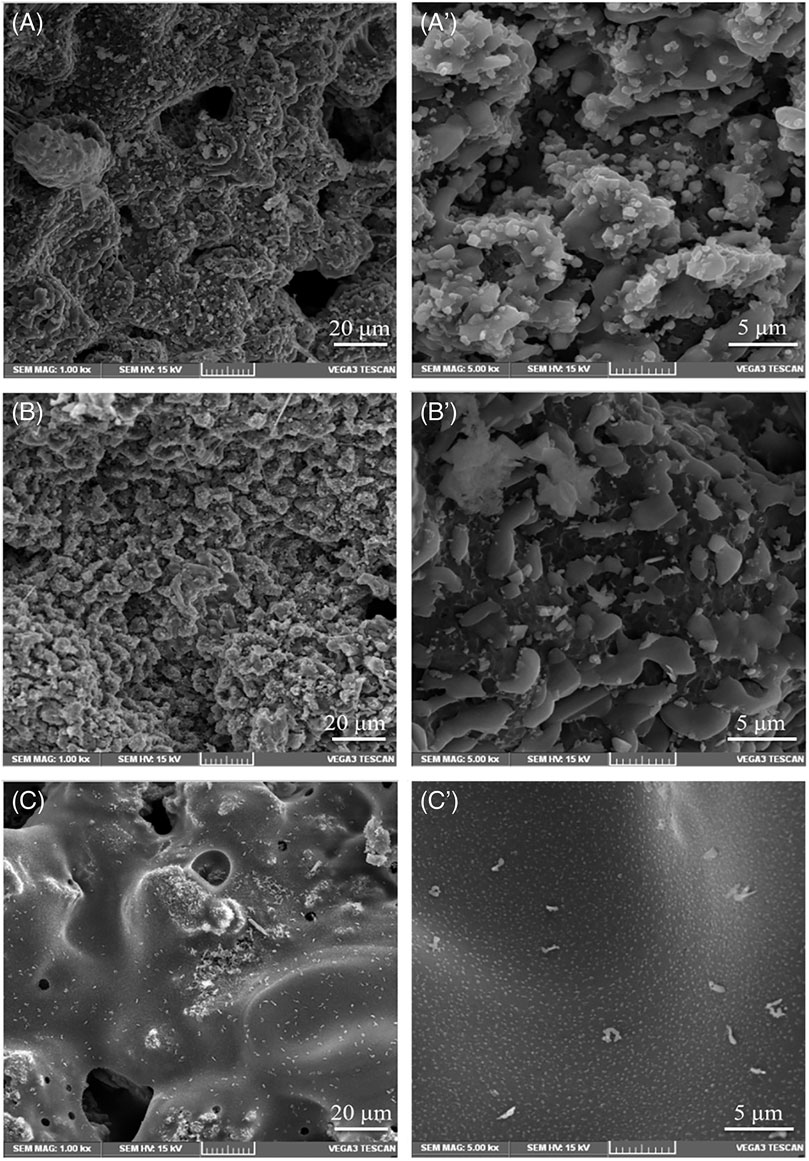
FIGURE 13. Scanning electron microscopy (SEM) images of the chars for PBS composites with (A, A′) 10 wt% CP-lignin, (B, B′) 20 wt% CP-lignin, and (C, C′) 30 wt% CP-lignin after cone calorimeter tests (Chen et al., 2018).
Conclusion and Perspectives
Recent years have witnessed the rapid development of waste-based flame retardants. Some industrial, domestic, and plant wastes have been applied in the fabrication of flame-retardant polymers because of their specific flame-retardant ingredients (see Table 1). However, the flame-retardant efficiency and compatibility issues of waste-based flame retardants have yet to be solved, thus restricting the practical applications of waste-based flame retardants. In the future, we should further increase the flame-retardant efficiency of waste-based flame retardants via the green method and prepare waste-based flame retardants with good compatibility for different polymers.
Author Contributions
CG contributed to writing—original draft, review, and editing. SH contributed to conceptualization, writing—original draft, review, and editing. ZC contributed to investigation.
Funding
The authors gratefully acknowledge the financial support for this work by the Natural Scientific Foundation of Zhejiang Province (No. LY20E030001) and the Startup Foundation of Quzhou University (No. BSYJ202001).
Conflict of Interest
Author ZC was employed by the company Ningbo Miruo Electronic Technology Co., Ltd.
The remaining authors declare that the research was conducted in the absence of any commercial or financial relationships that could be construed as a potential conflict of interest.
Publisher’s Note
All claims expressed in this article are solely those of the authors and do not necessarily represent those of their affiliated organizations, or those of the publisher, the editors and the reviewers. Any product that may be evaluated in this article, or claim that may be made by its manufacturer, is not guaranteed or endorsed by the publisher.
References
Cao, H., Zhang, Y., Li, X., Wang, F., and Zhang, X. (2017). Effects of Treated Waste Silicon Rubber on Properties of Poly(lactic Acid)/ammonium Polyphosphate Composites. J. Appl. Polym. Sci. 134 (34), 45231. doi:10.1002/app.45231
Chen, S., Lin, S., Hu, Y., Ma, M., Shi, Y., Liu, J., et al. (2018). A Lignin-Based Flame Retardant for Improving Fire Behavior and Biodegradation Performance of Polybutylene Succinate. Polym. Adv. Technol. 29 (12), 3142–3150. doi:10.1002/pat.4436
Chen, Y., Wang, W., Qiu, Y., Li, L., Qian, L., and Xin, F. (2017). Terminal Group Effects of Phosphazene-Triazine Bi-group Flame Retardant Additives in Flame Retardant Polylactic Acid Composites. Polym. Degrad. Stab. 140, 166–175. doi:10.1016/j.polymdegradstab.2017.04.024
Chen, Z., Jiang, J., Yu, Y., Chen, G., Chen, T., and Zhang, Q. (2020). Layer‐by‐layer Assembled Bagasse to Enhance the Fire Safety of Epoxy Resin: A Renewable Environmental Friendly Flame Retardant. J. Appl. Polym. Sci. 138 (11), 50032. doi:10.1002/app.50032
Dai, P., Liang, M., Ma, X., Luo, Y., He, M., Gu, X., et al. (2020). Highly Efficient, Environmentally Friendly Lignin-Based Flame Retardant Used in Epoxy Resin. ACS Omega 5 (49), 32084–32093. doi:10.1021/acsomega.0c05146
Das, O., Kim, N. K., Sarmah, A. K., and Bhattacharyya, D. (2017). Development of Waste Based Biochar/wool Hybrid Biocomposites: Flammability Characteristics and Mechanical Properties. J. Clean. Prod. 144, 79–89. doi:10.1016/j.jclepro.2016.12.155
Ding, N., Wang, X., Tian, Y., Yang, L., Chen, H., and Wang, Z. (2014). Chen H.-Z., Wang, Z.-CA Renewable Agricultural Waste Material for the Synthesis of the Novel Thermal Stability Epoxy Resins. Polym. Eng. Sci. 54 (12), 2777–2784. doi:10.1002/pen.23838
Feng, J., Sun, Y., Song, P., Lei, W., Wu, Q., Liu, L., et al. (2017). Fire-Resistant, Strong, and Green Polymer Nanocomposites Based on Poly(lactic Acid) and Core-Shell Nanofibrous Flame Retardants. ACS Sust. Chem. Eng. 5 (9), 7894–7904. doi:10.1021/acssuschemeng.7b01430
Huo, S., Song, P., Yu, B., Ran, S., Chevali, V. S., Liu, L., et al. (2021). Phosphorus-containing Flame Retardant Epoxy Thermosets: Recent Advances and Future Perspectives. Prog. Polym. Sci. 114, 101366. doi:10.1016/j.progpolymsci.2021.101366
Huo, S., Yang, S., Wang, J., Cheng, J., Zhang, Q., Hu, Y., et al. (2020). A Liquid Phosphorus-Containing Imidazole Derivative as Flame-Retardant Curing Agent for Epoxy Resin with Enhanced thermal Latency, Mechanical, and Flame-Retardant Performances. J. Hazard. Mater. 386, 121984. doi:10.1016/j.jhazmat.2019.121984
Jia, C. X., Chen, X. L., and Qian, Y. (2014). Synergistic Flame Retardant Effect of Graphite Powder in EVA/LDH Composites. Plastics, Rubber and Composites 43 (2), 46–53. doi:10.1179/1743289813Y.0000000072
Kabirifar, K., Mojtahedi, M., Wang, C., and Tam, V. W. Y. (2020). Construction and Demolition Waste Management Contributing Factors Coupled with Reduce, Reuse, and Recycle Strategies for Effective Waste Management: A Review. J. Clean. Prod. 263, 121265. doi:10.1016/j.jclepro.2020.121265
Kong, F.-b., He, Q.-l., Peng, W., Nie, S.-b., Dong, X., and Yang, J.-n. (2020). Eco-friendly Flame Retardant Poly(lactic Acid) Composites Based on Banana Peel Powders and Phytic Acid: Flame Retardancy and thermal Property. J. Polym. Res. 27, 204–215. doi:10.1007/s10965-020-02176-4
Kusakli, S., Kocaman, S., Ceyhan, A. A., and Ahmetli, G. (2020). Improving the Flame Retardancy and Mechanical Properties of Epoxy Composites Using Flame Retardants with Red Mud Waste. J. Appl. Polym. Sci. 138 (13), 50106. doi:10.1002/app.50106
Li, P., Wang, B., Xu, Y.-J., Jiang, Z., Dong, C., Liu, Y., et al. (2019). Ecofriendly Flame-Retardant Cotton Fabrics: Preparation, Flame Retardancy, Thermal Degradation Properties, and Mechanism. ACS Sust. Chem. Eng. 7 (23), 19246–19256. doi:10.1021/acssuschemeng.9b05523
Liu, L., Qian, M., Song, P. a., Huang, G., Yu, Y., and Fu, S. (2016). Fabrication of Green Lignin-Based Flame Retardants for Enhancing the Thermal and Fire Retardancy Properties of Polypropylene/Wood Composites. ACS Sust. Chem. Eng. 4 (4), 2422–2431. doi:10.1021/acssuschemeng.6b00112
Liu, L., and Wang, Z. (2018). Facile Synthesis of a Novel Magnesium Amino-Tris-(methylenephosphonate)-Reduced Graphene Oxide Hybrid and its High Performance in Mechanical Strength, thermal Stability, Smoke Suppression and Flame Retardancy in Phenolic Foam. J. Hazard. Mater. 357, 89–99. doi:10.1016/j.jhazmat.2018.05.052
Liu, S., Wang, C., Hu, Q., Huo, S., Zhang, Q., and Liu, Z. (2020). Intumescent Fire Retardant Coating with Recycled Powder from Industrial Effluent Optimized Using Response Surface Methodology. Prog. Org. Coat. 140, 105494. doi:10.1016/j.porgcoat.2019.105494
Nguyen, T. A. (2020). Effects of the Amount of Fly Ash Modified by Stearic Acid Compound on Mechanical Properties, Flame Retardant Ability, and Structure of the Composites. Int. J. Chem. Eng. 2020, 1–6. doi:10.1155/2020/2079189
Oualha, M. A., Omri, N., Oualha, R., Nouioui, M. A., Abderrabba, M., Amdouni, N., et al. (2019). Development of Metal Hydroxide Nanoparticles from Eggshell Waste and Seawater and Their Application as Flame Retardants for Ethylene-Vinyl Acetate Copolymer (EVA). Int. J. Biol. Macromolecules 128, 994–1001. doi:10.1016/j.ijbiomac.2019.02.065
Qian, Y., Li, S., and Chen, X. (2015). Synthesis and Characterization of LDHs Using Bayer Red Mud and its Flame-Retardant Properties in EVA/LDHs Composites. J. Mater. Cycles Waste Manag. 17, 646–654. doi:10.1007/s10163-015-0409-4
Saba, N., Jawaid, M., Alrashed, M. M., and Alothman, O. Y. (2019). Oil palm Waste Based Hybrid Nanocomposites: Fire Performance and Structural Analysis. J. Building Eng. 25, 100829. doi:10.1016/j.jobe.2019.100829
Shen, M.-Y., Kuan, C.-F., Kuan, H.-C., Ke, C.-Y., and Chiang, C.-L. (2020). Study on Preparation and Properties of Agricultural Waste Bagasse Eco-type Bio-Flame-Retardant/epoxy Composites. J. Therm. Anal. Calorim. 144 (2), 525–538. doi:10.1007/s10973-020-10368-9
Stéphanie, C.-S., Milena, M.-S., and Rafael, S.-P. (2017). Environmentally Friendly Intumescent Coatings Formulated with Vegetable Compounds. Prog. Org. Coat. 113, 47–59. doi:10.1016/j.porgcoat.2017.08.007
Urtekin, G., Hazer, S., and Aytac, A. (2020). Effect of Eggshell and Intumescent Flame Retardant on the thermal and Mechanical Properties of Plasticised PLA. Plastics, Rubber and Composites 50 (3), 127–136. doi:10.1080/14658011.2020.1844522
Wang, B., Xu, Y.-J., Li, P., Zhang, F.-Q., Liu, Y., and Zhu, P. (2020b). Flame-retardant Polyester/cotton Blend with Phosphorus/nitrogen/silicon-Containing Nano-Coating by Layer-By-Layer Assembly. Appl. Surf. Sci. 509, 145323. doi:10.1016/j.apsusc.2020.145323
Wang, C., Huo, S., Liu, S., Hu, Q., Zhang, Q., and Liu, Z. (2020a). Recycle of Magnesium alloy Scrap for Improving Fire Resistance, thermal Stability, and Water Tolerance of Intumescent Fire-Retardant Coatings. J. Coat. Technol. Res. 18 (2), 447–458. doi:10.1007/s11998-020-00413-5
Wang, X., Guo, W., Song, L., and Hu, Y. (2019). Intrinsically Flame Retardant Bio-Based Epoxy Thermosets: A Review. Composites B: Eng. 179, 107487. doi:10.1016/j.compositesb.2019.107487
Xu, Y., Li, J., Shen, R., Wang, Z., Hu, P., and Wang, Q. (2021). Experimental Study on the Synergistic Flame Retardant Effect of Bio-Based Magnesium Phytate and rice Husk Ash on Epoxy Resins. J. Therm. Anal. Calorim. 27, 1–12. doi:10.1007/s10973-020-10420-8
Xu, Z., Chu, Z., Yan, L., Chen, H., Jia, H., and Tang, W. (2018). Effect of Chicken Eggshell on the Flame‐retardant and Smoke Suppression Properties of an Epoxy‐based Traditional APP‐PER‐MEL System. Polym. Compos. 40 (7), 2712–2723. doi:10.1002/pc.25077
Xue, Y., Feng, J., Huo, S., Song, P., Yu, B., Liu, L., et al. (2020). Polyphosphoramide-intercalated MXene for Simultaneously Enhancing thermal Stability, Flame Retardancy and Mechanical Properties of Polylactide. Chem. Eng. J. 397, 125336. doi:10.1016/j.cej.2020.125336
Yang, H., Shi, B., Xue, Y., Ma, Z., Liu, L., Liu, L., et al. (2021a). Molecularly Engineered Lignin-Derived Additives Enable Fire-Retardant, UV-Shielding, and Mechanically Strong Polylactide Biocomposites. Biomacromolecules 22 (4), 1432–1444. doi:10.1021/acs.biomac.0c01656
Yang, H., Yu, B., Xu, X., Bourbigot, S., Wang, H., and Song, P. (2020). Lignin-derived Bio-Based Flame Retardants toward High-Performance Sustainable Polymeric Materials. Green. Chem. 22 (7), 2129–2161. doi:10.1039/d0gc00449a
Yang, S., Huo, S., Wang, J., Zhang, B., Wang, J., Ran, S., et al. (2021b). A Highly Fire-Safe and Smoke-Suppressive Single-Component Epoxy Resin with Switchable Curing Temperature and Rapid Curing Rate. Composites Part B: Eng. 207, 108601. doi:10.1016/j.compositesb.2020.108601
Yew, M. C., Ramli Sulong, N. H., Amalina, M. A., Johan, M. R., and Johan, M. R. (2015). Eggshells: A Novel Bio-Filler for Intumescent Flame-Retardant Coatings. Prog. Org. Coat. 81, 116–124. doi:10.1016/j.porgcoat.2015.01.003
Yin, W., Chen, L., Lu, F., Song, P., Dai, J., and Meng, L. (2018). Mechanically Robust, Flame-Retardant Poly(lactic Acid) Biocomposites via Combining Cellulose Nanofibers and Ammonium Polyphosphate. ACS Omega 3 (5), 5615–5626. doi:10.1021/acsomega.8b00540
Zabihi, O., Ahmadi, M., Khayyam, H., and Naebe, M. (2016). Fish DNA-Modified Clays: Towards Highly Flame Retardant Polymer Nanocomposite with Improved Interfacial and Mechanical Performance. Sci. Rep. 6, 38194. doi:10.1038/srep38194
Zabihi, O., Ahmadi, M., Li, Q., Ferdowsi, M. R. G., Mahmoodi, R., Kalali, E. N., et al. (2020). A Sustainable Approach to Scalable Production of a Graphene Based Flame Retardant Using Waste Fish Deoxyribonucleic Acid. J. Clean. Prod. 247, 119150. doi:10.1016/j.jclepro.2019.119150
Zhang, Y., He, S., Wu, J., Ma, J., Shao, S., He, L., et al. (2019). Application of Waste Silicon Rubber Composite Treated by N 2 Plasma in the Flame‐retardant Polypropylene. J. Appl. Polym. Sci. 136 (45), 48187. doi:10.1002/app.48187
Zhang, Y., Song, P. a., Fu, S., and Chen, F. (2015). Morphological Structure and Mechanical Properties of Epoxy/polysulfone/cellulose Nanofiber Ternary Nanocomposites. Composites Sci. Tech. 115, 66–71. doi:10.1016/j.compscitech.2015.05.003
Keywords: waste, sustainable development, polymeric materials, thermal stability, flame retardancy
Citation: Gao C, Huo S and Cao Z (2021) Solid Wastes Toward Flame Retardants for Polymeric Materials: A Review. Front. Mater. 8:712188. doi: 10.3389/fmats.2021.712188
Received: 20 May 2021; Accepted: 23 June 2021;
Published: 23 July 2021.
Edited by:
Pingan Song, University of Southern Queensland, AustraliaReviewed by:
Rongkun Jian, Fujian Normal University, ChinaXiaming Feng, Louisiana State University, United States
Copyright © 2021 Gao, Huo and Cao. This is an open-access article distributed under the terms of the Creative Commons Attribution License (CC BY). The use, distribution or reproduction in other forums is permitted, provided the original author(s) and the copyright owner(s) are credited and that the original publication in this journal is cited, in accordance with accepted academic practice. No use, distribution or reproduction is permitted which does not comply with these terms.
*Correspondence: Siqi Huo, c3FodW9AbmJ0LmVkdS5jbg==