Research Progress of Doped Manganite Materials in Magnetic Refrigeration
- 1College of Materials Science and Engineering, Guilin University of Technology, Guilin, China
- 2Collaborative Innovation Center for Exploration of Hidden Nonferrous Metal Deposits and Development of New Materials, Guilin University of Technology, Guilin, China
Magnetic refrigeration technology is a new, green, high-efficiency approach. It has attracted increasing attention from researchers and has a strong competitiveness over traditional refrigeration methods. With the continuous development of social lives, magnetic refrigeration technology must have important application prospects. This article briefly describes the basic principles. The focus is on the introduction and summary of research on perovskite manganite doping in magnetic refrigeration. Finally, the outlook and summary of magnetic refrigeration technology are presented.
Introduction
Magnetic refrigeration is a green and safe technology (Shen et al., 2021). Compared with gas compression refrigeration technologies, the gas compression refrigeration cycle can generally only reach 5–10% of the Carnot cycle, while magnetic refrigeration can reach 30–60% (Liu, 2009). Magnetic refrigeration is a technology that uses the magnetocaloric effect (MCE) of magnetic materials. MCE is unique as it changes the magnetic entropy of the material through variations in the external magnetic field. This is accompanied by the process of heat absorption and release in the material. This principle is illustrated in Figure 1 (Bao and Zhang, 2004).
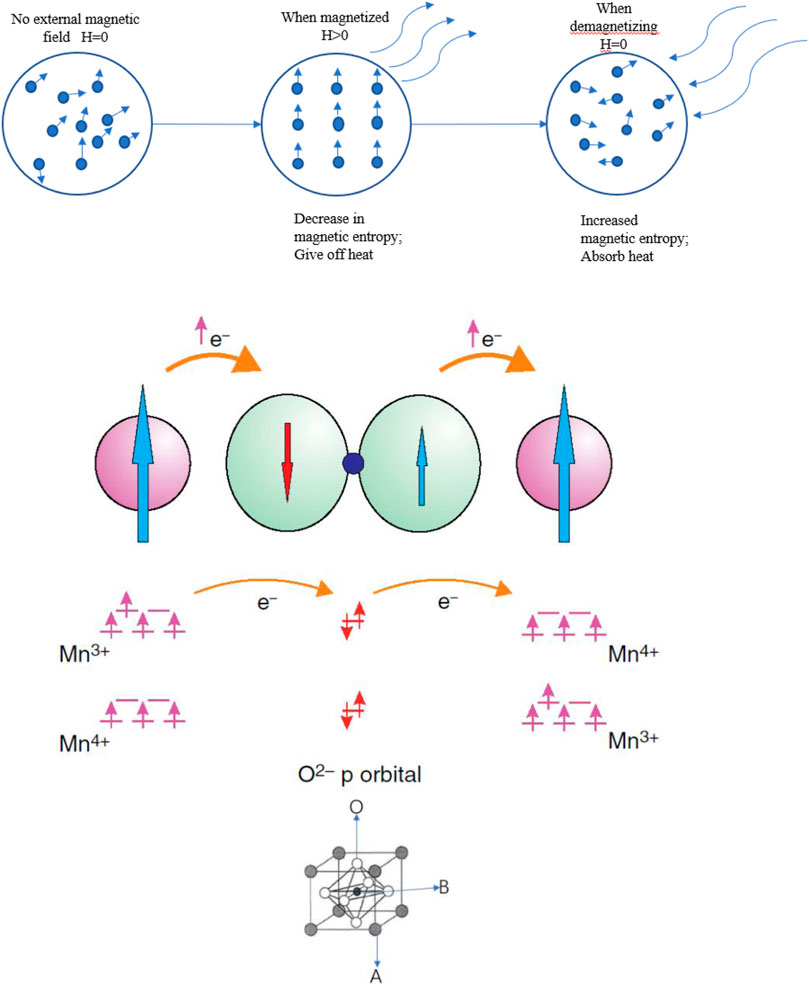
FIGURE 1. Schematic diagram of magnetic refrigeration, schematic diagram of double exchange mechanism and crystal structure of perovskites (ABO3).
There are many kinds of refrigeration materials. In 1976, Brown (1976) was the first to use Gd for magnetic refrigeration. Gd metals have a giant MCE, but their purity requirements are high and the price is too high for refrigeration. At the beginning of the 21st century, Wada et al. (2003) discovered a large MCE in the compound MnAs. Although MnAs as an Mn-based compound, has a good MCE, As is toxic. The perovskite manganese oxides have a wide range of Tc adjustments, easy preparation and synthesis, low cost, strong chemical stability, and high MCE. Therefore, many researchers have a strong interest in perovskite manganite in refrigeration.
The molecular formula of Perovskite manganite is RE1-xAExMnO3, where RE is a rare earth element and AE is an alkaline earth element. RE and AE constitute the A position of the Perovskite manganite structure, and Mn constitutes the B position, so the general formula is generally written as ABO3. The ideal ABO3 has a cubic structure, in which the A-site ion is located at the apex of the cubic unit cell, the B-site ion is located at the body center of the cubic unit cell, and O2- is located at the face center of the cubic unit cell. The tolerance factor (t) proposed by Goldsschmidt is usually used to describe the stability of perovskite manganite structure (Goldschmidt, 1926; Siwach et al., 2008). In fact, the ABO3 crystal will undergo lattice distortion, forming an orthogonal (t < 0.96) or rhombic (0.96 < t < 1) structure.
Different Ways of Doping
Ion doping can be divided into three categories: A-site, B-site, and vacancy doping. Ion doping produces three changes in perovskite manganite: 1) Change in ion valence, 2) Change in ion size, and 3) change in its crystal structure. The essential source of the magnetic properties for perovskite manganite is the double exchange effect (DE) of Mn3+-O2--Mn4+. It is believed part of the trivalent RE in perovskite manganite are replaced by low-valent AE and part of Mn3+ will be changed to Mn4+. The eg orbit becomes an empty state and uses O2- as a bridge between the two manganese ions of the two valence states to form DE (Zener, 1951; Anderson and Hasegawa, 1955). The principle of DE is shown in Figure 1. To better adjust the MCE and temperature of the magnetic phase transition, scientists have focused primarily on doping rare earth elements or AE to replace the A and B sites of perovskite manganite (CosKun et al., 2016; Ghosh and Ghatak, 2016; Sfifir et al., 2017).
A-Site Doping
At the A site, the wide variety of doped elements give differing magnetic moments, valence states, and ionic radii. This generates a mixed valence state of Mn3+ and Mn4+, which makes it possible to pass the DE at low temperatures and produce ferromagnetism. At the same time, the average ionic radius of the A-site ions, crystal structure, and magnetic moment of the molecules change. This affects the DE and MCE of the material while producing a wealth of magnetic order phenomena, such as the charge order, orbit order, and mutual coupling. After A-site doping, the internal DE and molecular magnetic moment of the material strongly influence the Curie temperature (Tc) and maximum magnetic entropy (
Based on the selected substrate RaMnO3 (Ra=La, Nd, Pr, Sm, such as LaMnO3), has been found to have better magnetocaloric properties in the room temperature range (Phan and Yu, 2007; Sun et al., 2017), especially in low and medium magnetic fields, which has a larger magnetic entropy change than that of Gd and GdSiGe alloy phases.
The types of ion doping can be divided into single-, multi-ion doping. Single-ion doping generally uses Na, Sr, Ag, Ca, Cd, Ba, and K plasmas at the A site, as shown in Table 1. The study found that under the same valence state, different doping ion radii have different effects on the structure and magneto-caloric properties of LaMnO3. Zhong et al. (1998), Zhong et al. (1999), Das and Dey (2007) studied La1-xNaxMnO3 (0 < x < 0.15) and La1-xKxMnO3 (0 < x < 0.2) materials, they found that under the conditions of the same magnetic field changes,
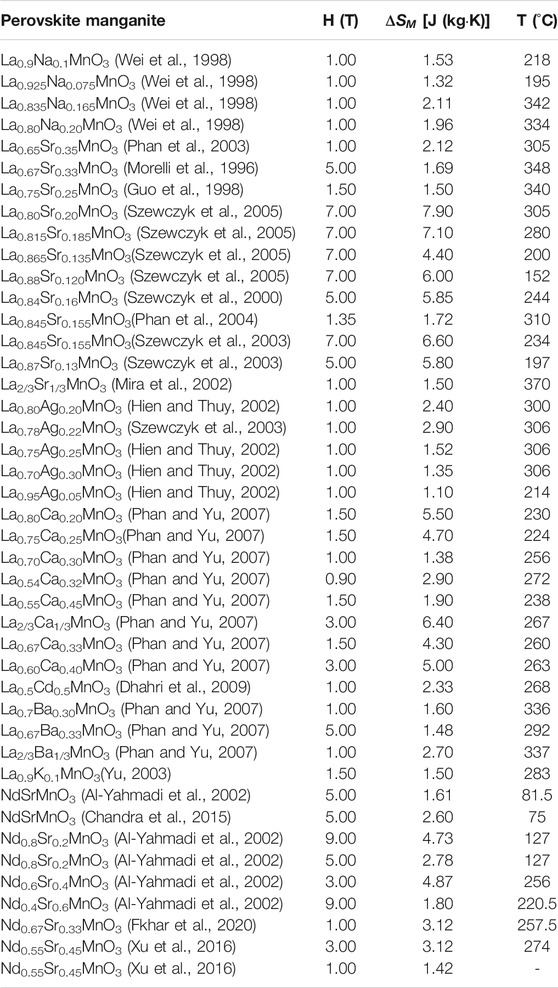
TABLE 1. Curie temperature and magnetic entropy change of perovskite materials substituted with different elements.
At the same time, the study found that the addition of alkaline earth metal or alkali metal ions that are lower in value than La in the A site has a significant change in the performance of LaMnO3. Mcbride et al. (2016), Demin and Koroleva (2004) and others have carried out research on the A-site Sr2+ doping of LaMnO3. They believe that the A-site doping of Sr2+ with a slightly larger radius than La3+ will cause the lattice structure to deviate from the ideal cubic structure, thereby producing Mn-O The bond length and Mn3+-O2--Mn4+ bond angle changes, the overlap of electron orbits increases the DE and the MCE. With the increase of Sr2+content, the magnetic entropy change of La1-xSrxMnO3 (0.1 < x < 0.3) increases, and Tc also increases,
When multi-ion doping, the effect of doping behavior on the magnetocaloric properties and Tc of perovskite manganite is more complicated. One study found that La0.65Sr0.35MnO3 (Phan et al., 2003) with an applied magnetic field of 1 T has Tc = 305 K, which is close to room temperature. The
In the study of magnetic refrigeration, Dagotto divided perovskite manganite into wide-, medium- and small-bandwidth types based on the bandwidth of the DE (Dagotto et al., 2001). La0.65Sr0.35MnO3 material belongs to the wide-bandwidth type, and another important perovskite manganite of Pr1-xSrxMnO3 belongs to the small-bandwidth type. The ferromagnetic-antiferromagnetic and paramagnetic-ferromagnetic transitions appear in the magnetic phase diagram simultaneously for x between 0.5 and 0.55 (Pollert et al., 2002). In this T range, as the Sr content at the A site increases, Tc gradually decreases, and the Neel temperature (critical temperature of antiferromagnetic-paramagnetic transition) increases with the two transitions when reaching a certain value (Martin et al., 1999). This shows that Sr2+ doping at the A site causes changes in the ratio of Mn ions, which affects the DE and phase change of the material. Since the 21st century, researchers have performed increasing studies on Pr1-xSrxMnO3. Bingham (Caballeroflores et al., 2014) found that Pr0.5Sr0.5MnO3 has a significant anomalous MCE. When the external magnetic field is 5 T,
B-Site Doping
To maintain the balance of the valence state after doping, A-site doping indirectly changes the ratio and content of Mn3+/Mn4+, changes the structure of the perovskite manganite, and affects its DE and rich physical properties (magnetic, electrical, etc.). As Mn3+-O2--Mn4+ is the basis of DE, the magnetic properties of ABO3 perovskite structures are usually produced based on B-site ions; thus, B-site doping directly affects the ratio of Mn3+/Mn4+. At the same time, other magnetic ions introduced by doping increase the abundance and complexity of the DE between B-site ions. Therefore, a small amount of doping is performed at the B-site to change the Tc and increase the magnetic moment of the site to increase the
B-site can be doped with transition metals (Ni, V, Fe, Co, Cr, Cu). Kong (2018) doped Ni and V at the B site for La0.65Sr0.35MnO3. When doped with Ni, the Tc decreased significantly with the doping content, which moved below room temperature. When the B site was doped with V, Tc showed an increasing trend, but the
Studies have found that after perovskite manganese oxide is doped at the B site and is placed under an external magnetic field, the Tc and
The main reasons why the doping effect of the B site is lower than that of the A site are as follows. 1) Changes in Tc depend directly on the DE, and mixed interactions occur after the B site is doped with ions. At greater doping contents and, after the doping ions replace the Mn sites, the DE between Mn3+-O2--Mn4+ decreases due to the increased super-exchange between doping and the surrounding ions, which causes the Tc to decrease. 2) After the B -sites are doped, the doping ions replace one of the Mn3+-O2--Mn4+ ions in the double exchange, such as Co ions. The diverse spin electronic states and complex valence states of Co ions produce complex DE. Thus, Co doping is considered to as a partial replacement of Mn ions for DE. When Co ions replace Mn, antiferromagnetic super exchange channels (Co3+-O2--Mn4+, Co2+-O2--Co4+) and ferromagnetic double exchange channels (Mn3+-O2--Mn4+, Co3+-O2-Co4+) form in the system (Ghosh et al., 1999). Thus, antiferromagnetic double exchange interactions and ferromagnetic double exchange interactions coexist. As antiferromagnetic and ferromagnetic exchanges coexist, as the doped Co ions content increases and the long-range ferromagnetic order of the sample is replaced with ferromagnetic cluster behaviors (Chainani et al., 1992). This result in spin magnetic moment direction. The deviation and chaotic magnetic moment of the spin result in an overall decreased magnetic moment and
During ion doping modification, more consideration should be given to doping at the A site of the perovskite manganese oxide to indirectly change the proportion of manganese ions to affect the crystal structure and physical properties of the perovskite manganese oxide.
Vacancy Doping
The crystal structure of perovskite manganese oxides can be changed through doping. Thus, research has been conducted to determine the impact of vacancy doping. Holes are divided into two categories: oxygen and elements. However, the generation of oxygen holes reduces DE of the material, while the Tc and
The Tc of the La0.925-xMnO3 prepared by Xu (Xu) decreased with x. La0.925-xMnO3 was also affected by the decreased Tc due to the ionic radius of the A site and the increased Mn4+ content, which led to an increased Tc. the large La0.925-xMnO3 ion vacancy concentration causes significant lattice distortion, and a smaller A-site ion radius is the main factor controlling the decrease in Tc.
La1-xCaxMnO3 has a high magnetic entropy change and a relatively low Tc, which can be increased by doping. La1-xCaxMnO3 replaces La3+ with Ca2+. To balance the valence, Mn4+ needs to be produced, which further modifies the magnetization and Tc of the material. Changes in the valence state of Mn ions can alter the magnetic properties of the material from ferromagnetic to paramagnetic. There are two energy levels for Mn ions. The principle of the lowest energy, high energies into two orbitals, which causes crystal lattice distortion. This system has therefore become a refrigeration material that is widely studied by scientific researchers. He (He, 2019) used the sol-gel method to prepare La0.65-xCa0.35MnO3 (0 ≤ x ≤ 0.15). The experimental results show that as the La3+ vacancy concentration increases, the ion radius and the mismatch factor change. The Tc of La0.65-xCa0.35MnO3 also gradually increases. When the external magnetic field is 0–5 T,
Brion (Brion et al., 1999) used the solid phase method to prepare La1-xMnO3. The study found that the Tc of La0.93MnO3 and La0.97MnO3 were 170 and 118 K, respectively, and the Mn4+ content was 21 and 9%. Brion believed that when x mol of La3+ is replaced with a vacancy, 3x mol of Mn3+ is converted into Mn4+. Walha et al. (2009) found that Mn4+ increased with the vacancy concentration. However, Sankar (Joy et al., 2002) prepared La1-xMnO3 using the solid-phase method, which indicated that Tc increased with the vacancy concentration but Mn4+ decreased. There are different experimental results for Mn4+ with changes in the vacancy concentration, which manifest in the A site of perovskite manganese oxide. A small amount of AE or RE with a large ion radius can be added when synthesizing samples. To generate cation vacancies, it is first ignored that there are more B-site ions than A-site ions, which can enter A-sites. Tang et al. (2007) researched and proposed the A1-xBO3-δ model based on the minimum energy principle and the crystal defects in thermal equilibrium theory. In the model, the A-site gap is larger than the B-site gap. Ions on the lattice points are generated in high-temperature heat-treated samples. The violent thermal movement results in the migration of vacancy defects, and large gaps are first occupied by ions. The lack of A-site ions is compensated by B-site ions (such as Mn). Therefore, vacancies in the sample appear in the A site instead of the B site.
Summary and Outlook
This paper introduces the basic principles of magnetic refrigeration. In particular, the relationship between the doping content and properties of perovskite manganese oxide materials is introduced in detail, provide reference value for the future study. In the 21st century, important breakthroughs have been made in the research and development of magnetic refrigeration materials. However, there are still many problems that need to be studied and solved. From the current research results, when Mn3+/Mn4+ is close to 2:1, the double exchange effect of the materials reaches the strongest, but the internal mechanism is still unclear. In addition, the performance of the materials is also related to the size of the doped ions on the symmetry of the crystal structure, and then the Jahn-Teller effect affects the performance of the material.
Magnetic refrigeration has a good application market at room temperature, such as air conditioners. In some research fields, refrigeration materials need to be light weight and have a wide range of temperatures for refrigeration, but current magnetic refrigeration materials cannot meet the requirements. Hence, research of magnetic refrigeration has good development and application prospects.
Author Contributions
ZX: Conceptualization, Data curation, Writing original draft. BH: Syntax modification. ZZ, ZM, and LL: Supervision.
Conflict of Interest
The authors declare that the research was conducted in the absence of any commercial or financial relationships that could be construed as a potential conflict of interest.
Publisher’s Note
All claims expressed in this article are solely those of the authors and do not necessarily represent those of their affiliated organizations, or those of the publisher, the editors and the reviewers. Any product that may be evaluated in this article, or claim that may be made by its manufacturer, is not guaranteed or endorsed by the publisher.
References
Abdouli, Kh., Cherif, W., and Omrani, H. (2019). Structural, Magnetic and Magnetocaloric Properties of La0.5Sm0.2Sr0.3Mn1-xFexO3 Compounds with (0≤x≤0.15). J. Magnetism Magn. Mater. 475, 635–642. doi:10.1016/j.jmmm.2018.12.007
Al-Yahmadi, I. Z., Abdel-Latif, A. L., and MaMari, F. A. (2002). Giant Magnetocaloric Effect and Magnetic Properties of Nanocomposites of Manganite Nd1-xSrxMnO3 (0.0 ≤ X ≤0.8) Synthesized Using Modified Sol-Gel Method. J. Alloys Compd. 857, 157566. doi:10.1016/j.jallcom.2020.157566
Anderson, P. W., and Hasegawa, H. (1955). Considerations on Double Exchange. Phys. Rev. 100 (2), 675. doi:10.1103/physrev.100.675
Bao, Yumei., and Zhang, Kangda. (2004). Magnetic Refrigeration Technology. Beijing: Chemical Industry Press.
Biswas, A., Bingham, N. S., and Phan, T. L. (2014). Impacts of First-Order Phase Transition and Phase Coexistence on the Universal Behavior of Inverse Magnetocaloric Effect. J. Appl. Phys. 115, 1479. doi:10.1063/1.4860940
Bohigas, X., Tejada, J., and Marnez-Sarrión, M. L. (2000). Magnetic and Calorimetric Measurements on the Magnetocaloric Effect in La0.6Ca0.4MnO3. J. Magnetism Magn. Mater. 208 (1-2), 85–92. doi:10.1016/s0304-8853(99)00581-8
Brion, S. D., Ciorcas, F., and Chouteau, G. (1999). Magnetic and Electric Properties of La1-δMnO3. Phys. Rev. B 59 (2), 1304–1310. doi:10.1103/physrevb.59.1304
Brown, G. V. (1976). Magnetic Heat Pumping Near Room Temperature. J. Appl. Phys. 47, 73673–73680. doi:10.1063/1.323176
Caballeroflores, R., Bingham, N. S., and Phan, M. H. (2014). Magnetocaloric Effect and Critical Behavior in Pr0.5Sr0.5MnO3. J. Phys. Condensed Matter.
Chainani, A., Mathew, M., and Sarma, D. D. (1992). Electron-spectroscopy Study of the Semiconductor-Metal Transition in La1-xSrxCoO3. Phys. Rev. B Condensed Matter 46. doi:10.1103/physrevb.46.9976
Chandra, S., Biswas, A., Phan, M. H., and Srikanth, H. (2015). Impacts of Nano-Structuring and Magnetic Ordering of Nd3+ on the Magnetic and Magnetocaloric Response in NdMnO3. J. Magnetism Magn. Mater. 384, 138–143. doi:10.1016/j.jmmm.2015.02.032
CosKun, A., TasArkuyu, E., and Irmak, A. E. (2016). The Structural, Magnetic, and Magnetocaloric Properties of La1-xAgxMnO3(0.05≤x≤0.25). J. Superconductivity Novel Magnetism 29, 2075–2084. doi:10.1007/s10948-016-3516-0
Dagotto, E., Hotta, T., and Moreo, A. (2001). Colossal Magnetoresistant Materials: the Key Role of Phase Separation. Phys. Rep. 344, 1–153. doi:10.1016/s0370-1573(00)00121-6
Das, S., and Dey, T. K. (2007). Magnetic Entropy Change in Polycrystalline La1-xKxMnO3 Perovskites. J. Alloys Compd. 440 (1-2), 0–35. doi:10.1016/j.jallcom.2006.09.051
Demin, R., and Koroleva, L. (2004). Influence of a Magnetic Two-phase State on the Magnetocaloric Effect in the La1−xSrxMnO3, Manganites. Phys. Solid State. 46 (6), 1081–1083. doi:10.1134/1.1767248
Dhahri, J., Dhahri, A., Oumezzine, M., Dhahri, E., Said, M., and Vincent, H. (2009). Magnetocaloric Properties of Cd-Substituted Perovskite-type Manganese Oxides. J. Alloys Compd. 467, 44–47. doi:10.1016/j.jallcom.2007.12.001
Linh, D. C., Thanh, T. D, Anh, L. H, Duong Dao, V, Piao, H-G, and Yu, S-C., (2017). Critical Properties Around the Ferromagnetic-Paramagnetic Phase Transition in La0.7Ca0.3-xAxMnO3 Compounds (A = Sr, Ba and X = 0, 0.15, 0.3). J. Alloys Compd. 725, 484–495. doi:10.1016/j.jallcom.2017.07.168
Duc, N. T. M., Hung, C. M., and Huong, N. T. (2019). Magnetic Interactions and Magnetocaloric Effect in (La0.5Pr0.5)0.6Ba0.4MnO3. Effect of A-site co-doping 49 (4), 2596–2607. doi:10.1007/s11664-020-07974-6
Fkhar, L., Lamouri, R., and Mahmoud, A. (2020). Enhanced Magnetic and Magnetocaloric Properties of La0.45Nd0.25Sr0.3MnO3/CuO Composite. J. Superconductivity Novel Magnetism 33, 2543–2549. doi:10.1007/s10948-020-05509-y
Ghosh, B., and Ghatak, A. (2016). Manganite (LaAMnO; A = Sr, Ca) Nanowires with Adaptable Stoichiometry Grown by Hydrothermal Method: Understanding of Growth Mechanism Using Spatially Resolved Techniques. J. Mater. Sci. 51 (21), 9679–9695. doi:10.1007/s10853-016-0201-4
Ghosh, K., Ogale, S. B., and Ramesh, R. L. (1999). Transition-element Doping Effects in LaCaMnO. Phys. Rev. B 59 (1), 533–537. doi:10.1103/physrevb.59.533
Goldschmidt, V. M. (1926). Die gesetze der krystallochemie. Naturwissenschaften 14 (21), 477–485. doi:10.1007/bf01507527
Guo, Z. B., Yang, W., and Shen, Y. T. (1998). Magnetic Entropy Change in La0.75 Ca0.25-xSrxMnO3 Perovskites. Solid State. Commun. 105, 89–92. doi:10.1016/s0038-1098(97)10064-3
He, Qingqing. (2019). Preparation of Ion-Doped Perovskite Manganese Oxide and its Magnetocaloric Effect. Guilin, Guilin University of Technology.
Hien, N. T., and Thuy, N. P. (2002). Preparation and Magneto-Caloric Effect of La1-xAgxMnO3 (x=0.10∼0.30) Perovskite Compounds. Physica B Phys. Condensed Matter 319, 168–173. doi:10.1016/s0921-4526(02)01118-3
Hussain, I., Anwar, M. S., and Kim, E. (2016). Impact of Ba Substitution on the Magnetocaloric Effect in La1-xBaxMnO3 Manganites. Korean J. Mater. Res. 26 (11), 623–627. doi:10.3740/mrsk.2016.26.11.623
Joy, P. A., Sankar, C. R., and Date, S. K. (2002). The Limiting Value of X in the Ferromagnetic Compositions La1-xMnO3. J. Phys. Condensed Matter 14, 663–669. doi:10.1088/0953-8984/14/39/104
Kong, Xiangyu. (2018). Preparation and Magnetocaloric Effect of Perovskite Manganese Oxide Magnetic Refrigeration Material. Guilin, Guilin University of Technology.
Kong, Xiangyu., and Zou, Zhengguang. (2018). Preparation of La0.65Sr0.2Gd0.15MnO3 Nanoparticles and its Magnetocaloric Effect. J. Chin. Ceram. Soc.
Liu, Tao. (2009). The Application prospect of Magnetic Refrigeration Technology. Refrigeration and Air Conditioning 23, 83–86.
Lu, W. J., Sun, Y. P., and Zhao, B. C. (2006). Induced Ferromagnetism in Mo-Substituted LaMnO3. Phys. Rev. B 73, 174425. doi:10.1103/physrevb.73.174425
Martin, C., Maignan, A., and Hervieu, M. (1999). Magnetic Phase Diagrams of L1-xAxMnO3 Manganites (L=Pr,Sm; A=Ca,Sr). Phys. Rev. B 60, 12191. doi:10.1103/physrevb.60.12191
Mcbride, K., Cook, J., and Gray, S. (2016). Evaluation of La1−xSrxMnO3 (0≤x<0.4) Synthesised via a Modified Sol–Gel Method as Mediators for Magnetic Fluid Hyperthermia. CrystEngComm 18 (3), 407–416. doi:10.1039/c5ce01890k
Mira, J., Rivas, J., and Hueso, L. E. (2002). Drop of Magnetocaloric Effect Related to the Change from First- to Second-Order Magnetic Phase Transition in La2/3(Ca1-xSrx)1/3MnO3. J. Appl. Phys. 91, 8903–8905. doi:10.1063/1.1451892
Morelli, D. T., Man, Ce. A. M., and Mantese, J. V. (1996). Magnetocaloric Properties of Doped Lanthanum Manganite Films. J. Appl. Phys. 79, 373–375. doi:10.1063/1.360840
Patra, M., De, K., and Majumdar, S. (2009). Multifunctionality Attributed to the Self-Doping in Polycrystalline La0.9MnO3: Coexistence of Large Magnetoresistance and Magnetocaloric Effect. Appl. Phys. Lett. 94, 2472. doi:10.1063/1.3095519
Phan, M. H., Phan, T. L., and Yu, S. C. (2004). Large Magnetocaloric Effect in La0.845Sr0.155Mn1−xMxO3 (M = Mn, Cu, Co) Perovskites. Physica Status Solidi 241, 1744–1747. doi:10.1002/pssb.200304587
Phan, M. H., Tian, S. B., and Hoang, D. Q. (2003). Large Magnetic-Entropy Change above 300 K in CMR Materials. J. Magnetism Magn. Mater. 258, 309–311. doi:10.1016/s0304-8853(02)01151-4
Phan, M. H., and Yu, S. C. (2007). Review of the Magnetocaloric Effect in Manganite Materials. J. Magnetism Magn. Mater. 308, 325–340. doi:10.1016/j.jmmm.2006.07.025
Pollert, E., Jirák, Z., and Hejtmánek, J. (2002). Detailed Study of the Structural and Magnetic Transitions in Pr1−xSrxMnO3 Single Crystals (0.48≤x≤0.57). J. Magnetism Magn. Mater. 246, 290–296. doi:10.1016/s0304-8853(02)00076-8
Sfifir, I., Ezaami, A., Cheikhrouhou-Koubaa, W., and Cheikhrouhou, A., (2017). Structural, Magnetic and Magnetocaloric Properties in La0.7-xDyxSr0.3MnO3 Manganites (x=0.00, 0.01 and 0.03). J. Alloys Compd. 696, 760–767. doi:10.1016/j.jallcom.2016.11.286
Shen, Jun., Mo, Zhaojun., Li, Zhenxing., Gao, Xinqiang., Sun, Hao., Xie, Huicai., et al. (2021). Research Progress in Magnetic Refrigeration Materials and Technology. J]Science China: Phys. Mech. Astron. 51, 7–21. doi:10.1360/sspma-2020-0339
Siwach, P. K., Singh, H. K., and Srivastava, O. N. (2008). Low Field Magnetotransport in Manganites. J. Phys. Condens Matter 20 (27), 273201. doi:10.1088/0953-8984/20/27/273201
Sun, Xiaodong., Duan, Yumei., and Yun, Huiqin. (2017). Magnetic Card Effect in ABO3(A=R1-xMx;B=Mn) Manganese Oxide Materials. J. Inner Mongolia Normal Univ. (Natural Chin. Edition) 46, 341–345.
Sun, Y., Xu, X., and Zhang, Y. (2000). Large Magnetic Entropy Change in the Colossal Magnetoresistance Material La2/3Ca1/3MnO3. J. Magnetism Magn. Mater. 219 (2), 183–185. doi:10.1016/s0304-8853(00)00433-9
Szewczyk, A., Gutowska, M., and Dabrowski, B. (2005). Specific Heat Anomalies in La1−xSrxMnO3 (0.12⩽x⩽0.2). Phys. Rev. B 71. doi:10.1103/physrevb.71.224432
Szewczyk, A., Gutowska, M., and Piotrowski, K. (2003). Direct and Specific Heat Study of Magnetocaloric Effect in La0.845Sr0.155MnO3. J. Appl. Phys. 94 (3), 1873–1876. doi:10.1063/1.1591411
Szewczyk, A., Szymczak, H., and Wisniewski, A. (2000). Magnetocaloric Effect in La1-xSrxMnO3 for x=0.13 and 0.16. Appl. Phys. Lett. 77, 1026–1028. doi:10.1063/1.1288671
Tang, G. D., Hou, D. L., and Chen, W. (2007). Estimation of Mn4+ Ion Content Ratio in Self-Doped Compound La1-xMnO3-δ. Appl. Phys. Lett. 91, 12503. doi:10.1063/1.2794777
Wada, H., Morikawa, T. K., Shibata, T., Yamada, Y., and Akishige, Y. (2003). Giant Magnetocaloric Effect of MnAs1-xSbx in the Vicinity of First-Order Magnetic Transition. Physica B: Condensed Matter, 328, 114–116. doi:10.1016/s0921-4526(02)01822-7
Walha, I., Ehrenberg, H., and Fuess, H. (2009). Structural and Magnetic Properties of La0.6-x□xCa0.4MnO3 (0 ≤ X ≤ 0.2) Perovskite Manganite. J. Alloys Compd. 485, 64–68. doi:10.1016/j.jallcom.2009.06.121
Wei, Z., Wei, C., and Ding, W. (1998). Magnetocaloric Properties of Na-Substituted Perovskite-type Manganese Oxides. Solid State. Commun. 106, 55–58.
Xu, L., Fan, J., and Zhu, Y. (2016). Magnetocaloric Effect and Spontaneous Magnetization in Perovskite Manganite Nd0.55Sr0.45MnO3. Mater. Res. Bull. 73, 187–191. doi:10.1016/j.materresbull.2015.08.024
Xu, Tiantian. Preparation of Perovskite Manganese Oxide Magnetic Refrigeration Material. Zhejiang University of Technology.
Yu, F. B. (2003). Review on Research of Room Temperature Magnetic Refrigeration. Int. J. Refrigeration 26, 622–636. doi:10.1016/s0140-7007(03)00048-3
Zener, C.. Interaction between the D-Shells in the Transition Metals. II. Ferromagnetic Compounds of Manganese with Perovskite Structure. (1951). Phys. Rev. 82 (3), 403–405.
Zhong, W., Chen, W., and Ding, W. (1998). Magnetocaloric Properties of Na-Substituted Perovskite-type Manganese Oxides. Solid State. Commun. 106 (1), 55–58. doi:10.1016/s0038-1098(97)10239-3
Keywords: magnetic refrigeration, green, high efficiency, perovskite manganite, doping
Citation: Xie Z, Zou Z, He B, Liu L and Mao Z (2021) Research Progress of Doped Manganite Materials in Magnetic Refrigeration. Front. Mater. 8:771941. doi: 10.3389/fmats.2021.771941
Received: 07 September 2021; Accepted: 15 October 2021;
Published: 01 November 2021.
Edited by:
Mangalaraja Ramalinga Viswanathan, University of Concepcion, ChileReviewed by:
Kadiyala Chandra Babu Naidu, Gandhi Institute of Technology and Management (GITAM), IndiaShabnam Andalibi Miandoab, Islamic Azad University of Tabriz, Iran
Dmitriy Karpenkov, National University of Science and Technology MISiS, Russia
Copyright © 2021 Xie, Zou, He, Liu and Mao. This is an open-access article distributed under the terms of the Creative Commons Attribution License (CC BY). The use, distribution or reproduction in other forums is permitted, provided the original author(s) and the copyright owner(s) are credited and that the original publication in this journal is cited, in accordance with accepted academic practice. No use, distribution or reproduction is permitted which does not comply with these terms.
*Correspondence: Zhengguang Zou, zouzgglut@163.com