- China Electronics Technology Group Corporation No. 38 Research Institute, Hefei, China
The development of information transmission technology towards high-frequency microwaves and highly integrated and multi-functional electronic devices has been the mainstream direction of the current communication technology. During signal transmission, resistance-capacitance time delay, crosstalk, energy consumption increase and impedance mismatch restrict the high density and miniaturization of Printed circuit board (PCB). In order to achieve high fidelity and low delay characteristics of high-frequency signal transmission, the development of interlayer dielectric materials with low dielectric constant (Dk) and low dielectric loss factor (Df) has become the focus of researchers. This review introduces the dielectric loss mechanism of polymer composites and the resin matrix commonly used in several high-frequency copper-clad laminates, and mainly describes how to reduce the dielectric constant and dielectric loss of materials from the level of molecular structure design, as well as the effect of fillers on the dielectric properties of polymer substrates. As a kind of potential functional fillers for dielectric polymeric composites, the carbon nanofillers are used to tailor the dielectric properties of their composites via different dimensions and loadings, as well as their proper preparation methods. This review finally summarizes the interface bonding failure mechanism and a feasible idea to optimize the dielectric properties of polymer matrix composites is also proposed.
Introduction
In recent years, the rapid development of information transmission technology has led to the gradual development of electronic devices in the direction of thin and light, high performance, high frequency and high speed. Owing to the development of high density and miniaturization of PCB, the core component of high frequency microwave communication, microwave signal transmission between integrated circuits and microstrip lines in the system is prone to mutual interference, resulting in the delay of resistance and capacitance (RC), crosstalk and increase of energy consumption. The copper clad laminate (CCL) is the carrier of the printed circuit board and plays a major role in its conductivity, insulation and support, largely determining the performance, quality, manufacturing costs and long-term reliability of PCB. Therefore, the fabrication of copper clad laminates for use at high frequencies has become the focus of researchers.
According to the signal transmission rate formula, the relationship between high frequency signal transmission speed V and signal transmission loss and interlayer dielectric resin can be expressed as follows:
Where V (m/s) and c (m/s) are the signal propagation speed and the speed of light in a vacuum. Where k is a Constant and
Where
Compared to the inorganic dielectric material silicon dioxide (SiO2), organic polymer materials as interlayer dielectric (ILD) generally have lower dielectric constants due to their smaller material density and lower single-bond polarizability. In addition, they show obvious advantages in the ease of chemical and geometric structure design. Resin matrix, as an important part of CCL, plays a decisive role in the dielectric properties of CCL. In order to ensure no void electroplating deposition during copper metallization, in addition to composition stability at high temperatures, organic polymers must also have very high glass transition temperatures: minimum temperature above 300°C and ideal temperature above 400°C (Shamiryan et al., 2004). In order to solve the high fidelity, low delay, and impedance matching of signal transmission, these interlayer dielectric resins must have an extremely low dielectric constant and dielectric loss, The dielectric properties of common resins are shown in Table 1.
Dielectric Loss Mechanism
Dielectric loss is caused by the failure of the molecular polarization process to keep pace with the rate of change of the oscillating applied electric field. When the relaxation time (τ) in the polymer is less than or equal to the rate of the oscillating electric field, there is no or minimum loss. However, when the oscillating speed of the electric field is much greater than the relaxation time, the polarization cannot change with the oscillation frequency, resulting in energy absorption and heat dissipation. When the dipole polarization is completely out of sync with the frequency of the applied oscillating electric field, the dielectric loss is also minimal.
The relative permittivity can be expressed by the following complex equation:
It consists of the real part of the dielectric constant (
Among them, dielectric materials have five types of polarization: interface polarization, ion polarization, dipole (orientation) polarization, atomic (vibration) polarization, and electron polarization. Each polarization is related to the dielectric loss at a particular frequency (Figure 1). These five types of polarization can be divided into two states: relaxation and resonance. Relaxation includes interfacial polarization, ion polarization, and dipole (oriented) polarization. For a multicomponent polymer system, Maxwell--Wagner effect generates interfacial and interfacial polarization. For polymers containing ions, whether impurity ions or ions in polymer electrolytes and polyelectrolytes, ion polarization occurs below a few hundred Hertz. If the polymer is polar and contains permanent dipoles, these permanent dipoles may respond to the external field by rotation, resulting in dipole (orientation) polarization in the polymer. There are two types of dipole relaxation: dipole segment relaxation (relaxation associated with micro-brownian motion of the entire chain, called α relaxation) and dipole group relaxation (i.e., relaxation associated with local motion of molecules and small chain units, called β and γ relaxation) (Prateek et al., 2016). The activation energy required for α relaxation is greater than that for β and γ relaxation, and the increase rate of α relaxation is higher when it is close to a glassy state. γ relaxation occurs at lower temperatures and is associated with the movement of small units of the main or side chain. Depending on their nature (amorphous or crystalline) and temperature, these dipoles relax between 10 Hz and a few GHz, covering the power and radio ranges (Figures 2–6).
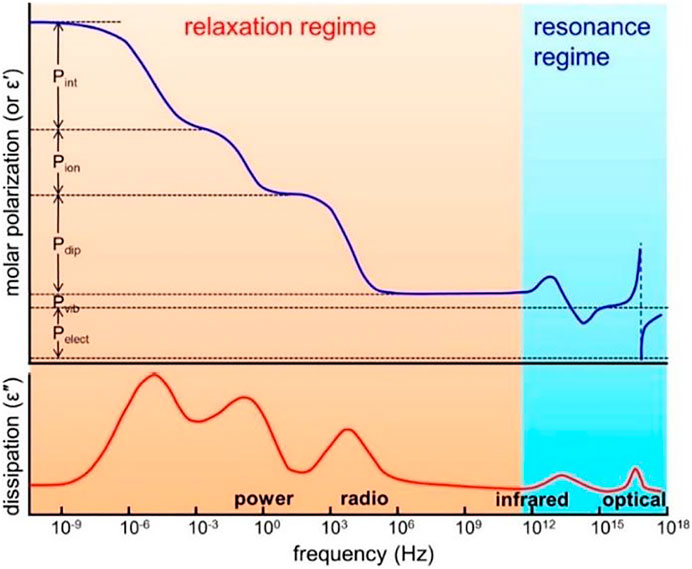
FIGURE 1. Different types of polarization as a function of frequency in polymers. Pelect, electronic polarization; Pat, atomic polarization; Pdip, (dipolar) orientational polarization; Pion, ionic polarization; Pint, interfacial polarization. The top panel shows the molar polarization (or the real part of permittivity), and the bottom panel shows the dissipation factor (the imaginary part of permittivity). Reprinted with permission from Prateek et al. (2016). Copyright© 2016 American Chemical Society.
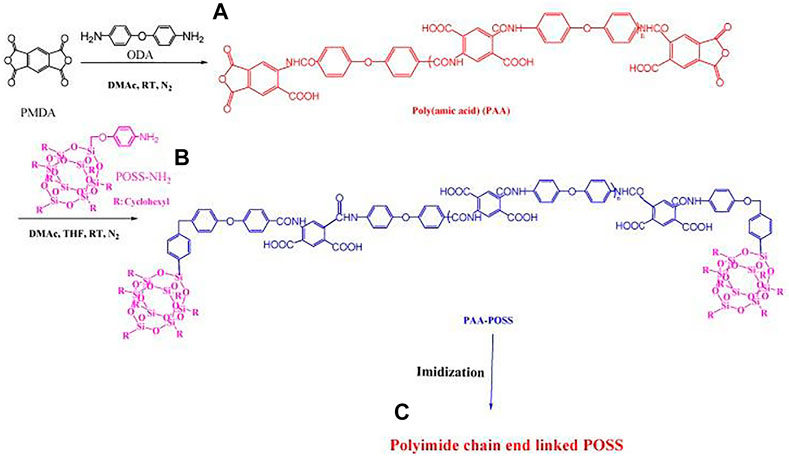
FIGURE 3. Preparation of (A) poly(amic acid) (PAA); (B) PAA/POSS nanocomposites; and (C) PI linked through its chain ends to POSS. Reprinted with permission from (Leu et al., 2003b). Copyright© 2003 American Chemical Society.
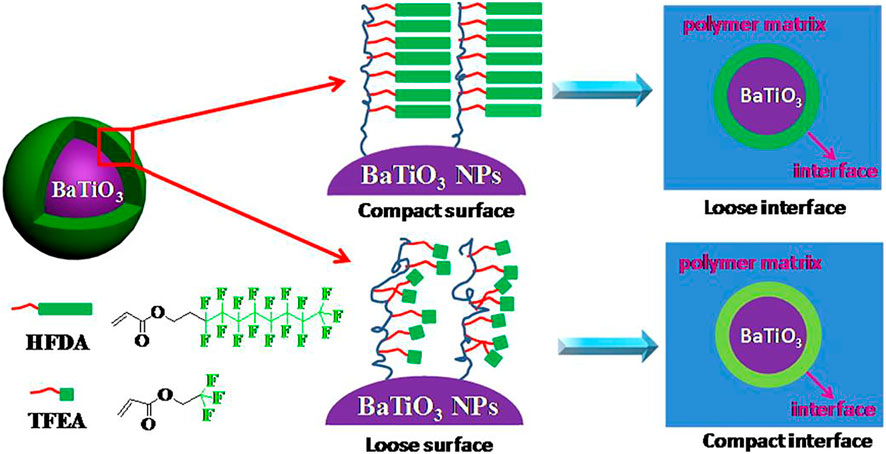
FIGURE 4. Schematic illustration for the fluoro-polymer@BT nanoparticles and the interfacial region within the nanocomposites. Reprinted with permission from Yang el al. (2013). Copyright© 2013 American Chemical Society.
For polymer materials, resonance includes electron polarization and atomic (vibrational) polarization. Both types of polarization exist in polymers, whether polar or nonpolar, amorphous or crystalline. Because their dielectric losses are in the infrared and optical ranges, they are not seen in power and radiofrequency. As can be seen from Figure 1, in the field of 10−6–1012 Hz radio wave communication, in the low-frequency range, the more polarization types, the higher the dielectric constant, and the higher the dielectric loss. As the frequency moves towards the high frequency until within 1012 Hz, various polarization response times gradually lag behind the change of electric field frequency, and the dielectric constant decreases gradually. Therefore, for the composites with dipole polarization and interfacial polarization, the dielectric constant of the composites decreases with the increase of frequency. In the high frequency region (102–106 Hz), the dielectric constants of the composites have little difference, and the difference is mainly reflected in the low frequency region (10–1–102 Hz).
Polymer Composites for High-speed Signal transmission
Commonly Used Resin Matrix for High Frequency Copper Laminates
The molecular structure of the polymer plays a major role in the dielectric and adhesive properties. However, the requirements for low dielectric constant, dielectric loss and strong adhesion of polymer materials are often contradictory. On the one hand, the strong adhesion to the microstrip conductor requires that the polymer chain contains polar functional groups such as amino, cycloxyl, and isocyanate, which can enhance the interfacial forces between polymer chains and microstrip conductors (usually copper foil) by electrostatic interaction, thereby improving the durability and reliability of integrated circuit boards. On the other hand, the orientation polarization of the intrinsic dipole moments combined with the dipole polarization of polar functional groups decisively causes a large increase in the dielectric loss; so, optimizing the dielectric properties (especially low dielectric loss) requires that polymer chains contain nonpolar functional groups. Besides, the position of the polar functional group is also very important: if the polar group is on the side chain of the polymer, especially the flexible polar group, which has strong mobility, it will have a greater impact on the dielectric properties; Polar groups have little effect if they are in the polymer backbone. The branching, cross-linking and orientation stretching of macromolecules also have an impact on the dielectric properties.
Polytetrafluoroethylene [-(CF2−CF2)n-] is a non-polar linear polymer with highly symmetrical structure, composed of two elements: carbon and fluorine. Due to the lack of active polar groups, high crystallinity, high electronegativity of fluorine atom and high dissociation energy of C-F bond, it has lower surface energy and higher surface hydrophobicity, making it hard affected by frequency, temperature and humidity. Except for compound modification and molecular structure design, the surface of PTFE can be directly treated to improve the adhesion performance of PTFE without reducing the bulk performance. PTFE is an available candidate for the high-frequency application in CCL, but its low inferior adhesion to substrates and poor processability, high coefficient of thermal expansion restrict its practical application. At present, sodium naphthalene, ion irradiation and plasma treatment are mostly used for its surface modification. Particularly, plasma modified the surface of PTFE without affecting the overall physical and chemical properties of PTFE.
Polyimide (PI) is a polymer with rigid chain of imide heterocycle, which is composed of binary amine and binary acid/anhydride with large free volume. Due to its excellent overall properties, such as thermal oxidation stability, unique electrical property and high mechanical strength, it is used as a polymer matrix for aerospace and microelectronics. In order to facilitate the processing of polyimide, many researchers have developed soluble polyimide. The main purpose of improving solubility is to reduce the interaction between polymer chains and the stiffness of polymer structure by introducing non coplanar, flexible and kink elements. For thermosetting polyimide, the molecular weight can be turned by the ratio of anhydride to amine group, and the polymer meeting the performance requirements can be obtained by crosslinking. The introduction of asymmetric dianhydride and diamine can not only limit the stacking of main chain and charge transfer electron polarization interaction, but also make polyimide have high Tg and thermal properties. Therefore, polyimides based on molecular or geometric asymmetry exhibit higher solubility, low melt viscosity and other required properties (Yu et al., 2018).
Epoxy resins are used as substrates or adhesives for copper-clad laminates due to its excellent properties, such as good moisture resistance, solvent resistance and chemical resistance, low shrinkage after curing, excellent electrical and mechanical properties, and good adhesion to many substrates. Taking bisphenol A diglycidyl ether epoxy resin as an example, the polar groups in the molecular chain such as ether bond and hydroxyl group are conducive to improve the adhesion between epoxy resin and copper foil. Rigid groups such as benzene ring in the molecular chain are beneficial to improve the rigidity and heat resistance of epoxy resin. The ether bond in the molecular chain endows the epoxy resin with good alkali resistance and flexibility. As a thermosetting resin, epoxy resin has good processability and reactivity, but the application of epoxy resins is limited by its brittleness, high dielectric loss and high dielectric constant. Generally, higher crosslinking degree will make it difficult to orient polar groups, so it will reduce the dielectric constant, but it is unfavorable to the mechanical properties. The crosslinking density and polar group concentration can be reduced by grafting flexible chains or blending with reactive functional groups.
Olefin polymers are carbon chain polymers without any polar groups, which endow dielectric materials with excellent dielectric properties. The common hydrocarbon resins include styrene-butadiene copolymer, styrene-butadiene-divinylbenzene copolymer, butadiene homopolymer and so on, only composed of C and H elements. Due to the small electronic polarizability of C-C and C-H, olefin polymers exhibit low dielectric constant and ultralow dielectric loss over wide frequency and temperature ranges. which are the ideal candidates for high-frequency CCL (Zhang, 2019). Among them, polybutadiene e.g. cis-1,4- polybutadiene, trans-1,4-polybutadiene, 1,2-polybutadiene and polybutadiene with different contents can be synthesized through different polymerization conditions and methods. The side chain of high 1,2-polybutadiene contains many double bonds (with a high degree of unsaturation) and is an amorphous structure, mainly used as an adhesive and sealant. Peroxide decomposed at different temperatures can be selected as crosslinking agent to control the curing temperature of crosslinking reaction. The absence of polar groups limits its application range to a certain extent. For low molecular weight non-vulcanized polybutadiene liquid rubber, the characteristic of easy-creeping makes it difficult to form a stable coating film at room temperature. Up to now, researches on polybutadiene utilized in CCL are relatively less. Other dielectric polymers also include polyphenylene ether (PPO), cyanate ester (CE), Poly (arylene ether nitrile) (Tong et al., 2019), parylene, polynaphthalene, polybenzoxazole resin, polynorbornene, Polyaryl ether, polyarylene, SiLK resin, polyquinoline, polyquinoxaline, polybenzocyclobutene, porous polymer materials (Volksen et al., 2010).
%1.2 Principles and examples of molecular structure design.
According to the Clausius-Mossotti formula:
Where k is dielectric constant, Pm is molar polarizability and Vm is molar free volume fraction. It can be seen from the formula decreasing the molecular molar polarization and increasing the free volume of the polymer are the main ways to reduce the dielectric constant.
The reduction of dielectric constant and dielectric loss by polymer molecular structure design is usually achieved by several key methods. 1) Introducing C-F and C-C chemical bonds composed of chemical elements with low polarizability. 2) Introducing asymmetric, rigid non-planar conjugated structure or flexible structure into the polymer main chain through copolymerization and block polymerization to reduce the molar polarizability. 3) Adding space-occupying groups such as methyl, ethyl and phenyl to the dielectric material to increase the free volume in the polymer structure.
Reduce Molecular Polarizability
First, the chemical elements involved in the preparation of low dielectric constant polymer materials can be appropriately selected to reduce the polarizability of the molecules. Bonding the precursors of atoms with appropriate electronegativity in an appropriate configuration can reduce the molecular polarizability. The incorporation of fluorine atoms is a good way to reduce the dielectric constant of polymers. The C-F bond has the lowest electronic susceptibility and dipole moment, making fluorination a potential candidate for low dielectric constant applications. On the one hand, fluorine atoms possess the strongest electronegativity and can better fix electrons, so the doping of fluorine atoms is particularly effective in reducing the electron and ion polarizability (Dhara and Banerjee, 2010). On the other hand, the bond energy of the C-F bond is very strong, and the fluorine-containing group contains a larger free volume.
Except for C and F, the thermal motion ability and orientation polarization ability of the segment units are different. Compared with aliphatic segment units, silicon is larger than carbon atoms, silicon-oxygen bonds are more flexible than carbon-carbon bonds, and siloxane (Si-O-Si) possesses a low dielectric constant. Therefore, the bulky silicone resin unit has relatively small mobility, which reduces the efficiency of the dipole’s response to changes in polarity at alternating frequencies. Haixia Qi et al. prepared a polyimide siloxane film by copolymerizing the synthesized disiloxane diamine (BATMS) with ODA and ODPA (Qi et al., 2019). Among them, the segments of ODPA-BATMS and ODPA-ODA are randomly distributed along the main chain of the copolymer, but the segments of ODPA-BATMS are alternately composed of disiloxane alkyl and imide rings (Figure 2). The flexible, high-temperature resistant disiloxane segment increases the molecular chain spacing and inhibits the charge transfer complex (CTC). The interaction between polymer chains is reduced, which helps to reduce chain accumulation and charge transfer electron polarization interactions. When the molar ratio of BATMS reaches 75%, the free volume and hydrophobic properties increase, and the molar polarization is significantly reduced. The dielectric constant of the copolymer film PI-75 is as low as 2.48 (1 MHz, 25°C). In addition, there exists the inherent non-polarization of the disiloxane segment and the change of the aggregation state of the copolymer film. The molar volume of the siloxane segment unit increases and the unit molar polarizability of the segment decreases. On the one hand, the introduction of asymmetric monomers or large side groups increases the free volume of PI molecules and disrupts the formation of effective CTCs. On the other hand, this reduces the interaction between polymer chains and the rigid structure of the polymer, which can improve the melt processability of the polymer or the solubility in organic solvents.
Polyhedral oligomeric silsesquioxane (POSS) with empirical formula (RSiO1.5) is the smallest silica nanoparticle (NP), where R can be an organic functional group (such as amine, alkyl, alkylene, epoxide unit, acrylate, hydroxyl) or hydrogen atom. According to the number of R and the preparation method of nanocomposites, Mohamed et al. classified it as a blend of non-functional POSS nanocomposites and polyimide, and a chain of monofunctional POSS nanocomposites and polyimide (Mohamed and Kuo, 2019), and the covalent connection of the end or side chain, the covalent bonding of the bifunctional POSS nanocomposite material and the polyimide main chain, and the formation of the polyimide thermal crosslinking structure of the multifunctional POSS nanocomposite material. Among them, POSS molecules are usually stable at temperatures as high as 400°C, which is higher than the thermal degradation temperature of most polymers. Incorporating them into some polymers leads to improved thermal stability and mechanical properties (Leu et al., 2003a). Wei et al. synthesized POSS-diamine monomer, and synthesized nano-polyimide composites with an adjustable dielectric constant by controlling the molar ratio of POSS-diamine monomer, PMDA, and ODA (Leu et al., 2003b). At the same time, POSS can be used as a large steric hindrance substituent group, the lowest dielectric constant of this composite material is 2.3, and the mechanical properties are controllable.
Leu et al. used pyromellitic dianhydride (PMDA) react with 4,4'-oxydiphenylamine (ODA) in DMAc under N2 atmosphere at room temperature to form PAA (Leu et al., 2003a). Then, POSS-NH2 reacts with PAA in DMAc to obtain PAA/POSS nanocomposite (Figure 3). After the PAA/POSS nanocomposite is thermally imidized at 300°C, its chain end is connected with POSS nanoparticles to obtain PI. Upon addition of 2.5 mol% of POSS units, the dielectric constant of the resulting polyimide POSS nanocomposite decreased from 3.40 to 3.09 for the pure PI without any decrease in mechanical strength. POSS cage-like siloxane structure is currently a better silicon-containing group for modified PI, which can overcome the disadvantages of reduced mechanical properties and high cost due to dependence on fluorine-modified materials.
Xiangxiu Chen et al. synthesized trapezoidal multifunctional polysiloxane (PN-PSQ) with a large number of amino groups and phenanthrene phosphide structures so as to improve the heat and fire resistance, dimensional stability and dielectric properties of BMI resin (Chen et al., 2014). On the one hand, PN-PSQ resin with phosphide-phenanthryl group has larger benzene side groups and non-coplanar structure; on the other hand, the–NH2 group of PN-PSQ resin shortens the distance between curing crosslinking points of BMI resin which to a certain extent increased the crosslinking density of BMI resin, so the orientation and relaxation of dipoles which accompanying the movement of polymer segments is limited, and finally the dielectric properties (the dielectric constant and loss at 1 MHz are 3.01 and 0.008, which are only 88 and 80% of that of BMI resin, respectively) are optimized. While there are polar reactive groups in PN-PSQ, their good symmetry has little effect on the dielectric constant and loss of the resin.
Devaraju et al. blended glycidyl terminated hyperbranched polysiloxane (HPSiE) with different percentages into diglycidyl ether bisphenol A (DGEBA) resin, and added curing agent diaminodiphenylmethane (DDM) (Devaraju et al., 2013). With the increase of HPSiE composition, the flexible polysiloxane bonding arising more porous voids, compared with pure DGEBA resin (the dielectric constant is 4.5), the dielectric constant can reach 3.5 at 15 wt%. Owing to the chemical interaction between HPSiE and DGEBA and the existence of Si-O-Si hydrophobic bond in the composites, the contact angle and water resistance are enhanced. Jie Dong et al. make amino-functionalized hyperbranched polysiloxane (NH2-HBPSi) graft onto PI chain by in-situ polymerization (Dong et al., 2017). The large bulk of HBPSi enhances the free volume and dielectric confinement effect, the dielectric constant of 16 wt% NH2-HBPSi/PI can be as low as 2.2, and the dielectric loss is about 0.01 at 108 Hz.
Increase the Free Volume of Polymer
Chen, Jiangbin et al. designed and synthesized a benzoxazine resin with high frequency, low dielectric constant (<3) and very low dielectric loss (tan<0.005) (Chen et al., 2019). The large -C(CH3)3 group was introduced into the end of benzoxazine copolymer, which effectively increased the free volume of the polymer and reduced the molecular polarity of it. Both alicyclic and aliphatic units have lower molar polarizability and molecular chain packing density, which can destroy the planarity of molecular chains and inhibit the charge transfer interaction within chains and/or between chains at the same time, and it is beneficial to improve the solubility of materials, reduce the dielectric constant and improve the optical transparency (Zhuang et al., 2019). In addition, the alicyclic structure can improve the hydrophobicity of the material and keep the dielectric properties of the film stable. Takashi Tasaki et al. developed a soluble polyimide with benign heat resistance and low dielectric properties (Dk is 2.85, Df is 0.0056 at 10 GHz) by optimizing the composition ratio of aliphatic, alicyclic and aromatic groups in the main chain of polyimide (Tasaki, 2018). In addition, polyimide adhesive shows good adhesion to common polyimide film and copper foil, and can be used in three-layer flexible copper-clad laminate (FCCL). The FCCL shows a transmission loss similar to that of liquid crystal polymer (LCP) FCCL at a frequency less than 20 GHz.
Ping Yu et al. used 3,4'-diaminodiphenyl ether (3,4'-ODA), 2,3,3',4'-biphenyl tetracarboxylic dianhydride (a-BPDA) and 2,3,3',4'-Oxydiphthalicanhydride (a-ODPA) as raw materials, synthesizing a new asymmetric bismaleimide oligomers with different molecular weights and dianhydride (Yu et al., 2019). Oligomers have high solubility and solubility stability in common organic solvents. Low molecular weight OD-BMI-1 and BP-BMI-1 oligomers have lower melt viscosity and wider processing window.
Others
Introducing air holes or nano foam materials, blending inorganic low dielectric materials, forming hyperbranched structures (Lei et al., 2016; Dong et al., 2017) and the use of cross-link interpenetrating network structure (Song et al., 2019) can be beneficial to reduce the orientation of dipole and improve the comprehensive properties of polymer. For nano-porous composites, the structure, size and size distribution of pores will have a vital impact on the uniformity of material properties, which is not conducive to the preparation process of large-scale production. In addition, the introduction of the hole structure will significantly reduce the mechanical properties and air tightness of the material, therefore, the material has low mechanical strength and poor barrier performance, which cannot meet the requirements of practical application.
The Influence of Fillers on the Dielectric Properties of Polymer Matrix
The morphology of inorganic particles includes zero-dimensional nanofillers (spherical nanoparticles, nanocubes and nanoparticles with irregular morphology), one-dimensional nanofillers (nanowires, nanofibers, nanotubes, such as carbon nanotubes CNT) and two-dimensional nanofillers (including nanosheets and nanoplates, such as graphene and nanoclay). Among them, it is often used Al2O3 (Yao et al., 2015) SiO2、TiO2、h-BN (Yang et al., 2017; Ge et al., 2019)、AlN (Wang et al., 2019) and ZrTi2O6 to improve the dielectric and mechanical properties. Because of the hydrophilicity of ceramic filler surface and the hydrophobicity of polymer, there is interface incompatibility, which leads to the aggregation of nanoparticles (Mittal et al., 2018). The difference of dielectric constant and conductivity between ceramic filler and polymer does not match at the interface, which leads to the accumulation of charge and local redistribution of electric field, and produces Maxwell-Wagner interface polarization (Murugaraj et al., 2005). Compared with zero-dimensional nano-filler, one-dimensional ceramic filler is easier to permeate, which leads to higher relative dielectric constant and heat conductivity coefficient. At the same time, large dipole moment is beneficial to the dielectric enhancement of polymer composites under low load. The zero-dimensional nano filler is easy to agglomerate because of its high surface area and volume effect, and is difficult to form monodisperse nanoparticles in polymer matrix, so it is necessary to modify the surface of inorganic particles (Kango et al., 2013). In order to obtain the strong interaction between polymer and nano-filler, the dispersibility of nano-filler and its compatibility with polymer are of vital importance. The filler dispersion of polymer/nanofiller composites (PNC) which is prepared by solution intercalation, in-situ intercalation, melt intercalation and other methods can be improved to a varying extent. The surface treatment of nano-filler, among which the surface modification can obtain good dispersibility and/or compatibility with polymer of nano-filler, and improve Tg of composite material.
Carbon nanotubes (CNTs) and graphene nanoplatelets (GnPs) are respectively typical one-dimensional and two-dimensional carbon nanofillers, which are commonly used in polymeric composites with dielectric properties (He et al., 2017; Zhao et al., 2019). It is well known that a sudden increase in the dielectric permittivity with one or several orders of magnitude is presented when the loading of conductive fillers reaches a critical value (Xu et al., 2015), i.e., the percolation threshold. However, when the content of the conductive filler is very close to or above the percolation threshold, conductive networks are generated and, thus, significant dielectric loss caused by leakage conduction loss from the capacitors would occur (Huang and Terentjev, 2010). On this basis, the dielectric behavior of polymeric composites can be tailored by tuning the conductive network structures, such as by compounding appropriate contents of carbon fillers in polymer matrix, as well as controlling the agglomeration situation of conductive nanofillers.
Li et al. studied the dielectric properties of polyamide 11(PA11)/CNT and PA11/Polypropylene (PP)/CNT composites, and the dielectric constant of all systems enhances significantly when the loading of CNTs approaches the conductively percolative threshold (Li et al., 2019). As for the PA11/CNT system, when the content of CNTs reaches up to 0.1 wt%, it shows an obvious increase of the dielectric constant from 6 to 50 at 100 Hz. The AC conductivity of the exceeded loading of 2 wt% CNTs shows a typical frequency-independence within the low frequency range, presenting a typical transition from insulation to conduction. In terms of PA11/PP/CNT composites, a lower percolative loading on 0.05 wt% of CNTs was presented (A9P1C0.05) when the dielectric constant underwent its sharp increase, arising from the segregated conductive structure of CNTs in the PA11/PP blend. For the possible reason, the author proposed the effect of “barrier medium” PP in the segregated CNT network on the dielectric property. They thought the PP long chain can diffuse into the CNT bundles with the incorporation of polar PP-g-MAH, enhancing interfacial interaction between CNTs and PP. As a result, the charge accumulation under electric field would be enhanced via interfacial polarization. The mini-capacitor network with CNTs as electrodes and a very thin PP layer as dielectric can be formed in the composites near the percolative threshold, which is correlated with the increment of dielectric constant. In addition, the PP in between CNTs can hinder the connection of CNTs and the current leakage, finally resulting in the suppressed increase of dielectric loss. (Re. Constructing a segregated carbon nanotube network in polyamide-based composites towards high dielectric constant and low loss).
As is mentioned above, nanofiller is easy to agglomerate because of its high surface area and volume effect. According to the micro-capacitor model (Prateek et al., 2016), a better dispersion of conductive particles in an insulating polymer matrix is capable of generating more micro-capacitors, which is benefit for achieving higher polarization effect. Zhu et al. reported CNT/PVDF dielectric composites dielectric composites, in which CNT was modified by coating with the polydopamine (PDA) (Zhu et al., 2017). In this study, the coating layer was controlled by changing the concentration of dopamine monomers, and this PDA coating, playing roles of constructing the barrier layer and hindering CNTs aggregation, benefiting the dispersion of CNT in the PVDF matrix. It is exhibited that both of the dielectric permittivity and loss factor of the composites gradually decrease accompanied with the thickening of PDA coatings, which can be ascribed to the weakened interfacial polarization between conducting particles and polymeric matrix.
The influence of interface on the dielectric properties of nanocomposites has been widely studied and can be described from two aspects (Zhang et al., 2018). On one hand, the interface structure characteristics (such as inorganic/organic compatibility, polymer/filler interaction, polymer chain configuration and particle surface state) can determine the final dispersion or aggregation of ceramic nanoparticles in polymer matrix. On the other hand, abnormal local interface electrical/dielectric properties (e.g. interface electronic state and effects on traps or scattering points, charge density, charge redistribution and electric field) can adjust the dielectric and breakdown behavior of nanocomposites and the overall performance of polymer and ceramic fillers. Polymer-filler particle interface model, such as dielectric bilayer model (LEWIS, 1994), multi-core model (Tanaka et al., 2005) and interphase power law model (Todd and Shi, 2005), phase field model (Shen et al., 2018) etc., the development of these model can explain the influence of interface effect on electrical and dielectric properties of nanocomposites. The model makes idealized assumptions on the size and shape of micro/nano-particles, and a single theory or model cannot explain the interaction between interfaces. According to the volume fraction, shape and direction of particles in the filler, the ratio of dielectric constant between filler and matrix and the interaction between filler and matrix, the geometric shape, size, concentration and size-to-dielectric constant of composite materials can be predicted through one or more equations in the model.
The change of polymer molecular structure (free volume fraction, mobility, crystallinity and polymer chain configuration) from interface to matrix caused by interface will affect the way of charge transport and the dielectric properties of polymer matrix. Generally, it is proved that the dielectric constant of the interface region surrounded by filler is lower than that of the bulk polymer matrix (Anandraj and Joshi, 2018). Theoretically, nanoparticles with larger surface area and higher interface strength can effectively limit the orientation and movement of dipoles in the interface region. Although interfacial polarization increases the dielectric constant to a certain extent, interfacial inhibition plays a major role in affecting the dielectric constant of composites. The bonding between filler and matrix also helps to reduce dipole polarity, the decreasing of mobility of polymer chain and the charge transfers lead the dielectric loss decreases simultaneously. However, in fact, nano-materials have a larger surface area than micron-materials (higher dielectric constant and loss due to higher interfacial polarization), which leads to easy aggregation of materials in the matrix (especially at higher load, aggregation of nanofillers and its poor compatibility with polymers will lead to induced voids and matrix density decreases). Aggregation of nanofillers and poor compatibility with polymers will lead to weak interaction between nanofillers and polymers, it means that there is more free volume at the interface between filler and polymer, which makes it cannot effectively limit the mobility of polymer chain. At the same time, the ceramic filler is hydrophilic in nature, if the particle surface is not coupled with silane coupling agent, its hygroscopicity will increase, resulting in a decrease in dielectric properties. In addition, if silane coupling agent is used for surface modification, the introduction of polar groups may increase the dielectric constant. It should be understood that silane coupling agent improves the interface adhesion.
Wu et al. used silica (SiO2) to coat multi-walled carbon nanotubes (MWCNTs) to form a core-shell structure (SiO2@MWCNTs) and organically modified montmorillonite (O-MMT) synergistically modified Reinforce epoxy resin (Wu et al., 2017). Under the lower filling of nanometer materials, the firm interface between the filler and the polymer and the entanglement of EP molecular chains are conducive to restricting the movement of charge carriers, and contributing to the reduction of dielectric constant and dielectric loss. On the one hand, its surface has abundant polar groups (-OH), which produces higher interface strength with the polymer. On the other hand, the one-dimensional filler carbon nanotubes (CNT) with high aspect ratio has a relatively low surface area, which helps to reduce the surface energy, thereby preventing filler agglomeration (Huang et al., 2019a). In addition, the conductive percolation threshold of most CNT/polymer conductive nanocomposites is less than 1 wt%. Therefore, higher interface strength and larger surface area can effectively limit the orientation of the dipole and the movement at the interface. At the same time, the SiO2 layer can effectively inhibit the electron transfer and accumulation of the composite. However, under the filling of higher nanomaterials, the aggregation of CNTs will reduce the area of the interface region. Compared with epoxy resin, carbon nanotubes have a higher dielectric constant. At this time, the interface polarization is dominant, so the dielectric constant increases instead.
Graphene oxide acts as an insulator, and its oxygen atoms are randomly attached to the graphene. The sp2−hybridized carbon atoms are converted into the sp3−hybridized carbon in GO, which reduces the conjugation and limits the π electrons, and loses the graphene’s conductivity. In view of the homogeneity of GO is not as high as that of graphene, and the electron mobility is much lower. The dielectric constant of the film is determined by the dielectric constant of the PI matrix and the impedance of GO. Jen-Yu Wang prepared two low dielectric constant and ultra-high strength graphene oxide (GO)/polyimide composite films (PI-GO and PI-ODA-GO films) through solution blending and in-situ polymerization (Wang et al., 2011). As the GO content increases, the dielectric constant (Dk) decreases to 2.0. Moreover, the uniform dispersion of ODA-GO in the PI-ODA-GO composite film prepared by in-situ polymerization leads to an increase in the dielectric constant. In addition to the introduction of ODA-GO can increase the free volume in the PI matrix, at the same time the partially reduced GO sheet evenly dispersed in the PI can also form many heterojunctions, limiting the movement of electrons in the PI, that is, limiting The electrons in PI are polarized, thereby reducing its dielectric constant. On the one hand, if the GO surface has many polar functional groups, the molecular polarization of PI is increased. On the other hand, many tiny incompatible interfaces are formed between PI and GO, and the interaction force is weak, which is equivalent to introducing a large amount of interface polarization to produce interface polarization.
Yang et al. synthesized core-shell fluoropolymer@BaTiO3 hybrid nanoparticles with different shell thicknesses or different shell structures through reversible addition-fragmentation chain transfer (RAFT) polymerization (Yang et al., 2013). The encapsulated shell polymer is chosen to be more insulating to maintain high resistivity and low loss. Using this hybrid nanoparticle as a filler, a solution blending method was used to prepare an energy storage nanocomposite based on polyvinylidene fluoride-hexafluoropropylene copolymer [P(VDF-HFP)] (Figure 4). Among them, the BT-PTFEA hybrid nanoparticles with shorter side groups have a higher graft density, and the interface formed by the close packing limits the mobility of the macromolecular chains of the matrix polymer, resulting in the lowest dielectric loss [from Pure (VDF-HFP) 0.032 is reduced to the lowest 0.016].
Among them, the dielectric loss of dielectric materials usually comes from three different factors: direct current (DC) conduction, space charge transfer (interface polarization) and the movement of molecular dipoles (dipole loss). Generally speaking, a decrease in polymer content will result in a decrease in molecular dipoles, which means a decrease in dipole loss; BaTiO3 nanoparticles are covered by a stable and dense fluoropolymer shell, the formation of an insulating layer outside the dielectric nanofiller limits the transfer and accumulation of space charges in the nanocomposite material. Surface modification by fluoropolymer improves the dispersibility of BT nanoparticles and enhances the interfacial adhesion of nanocomposites, which may further limit the movement of molecular dipoles.
Interface Design of Polymer Composite Material and Metal Microstrip Wire
The interface not only exists between polymer-filler particles, polymer-polymer, and filler particles-filler particles. For copper-clad laminates, the interface between polymer composites and metal microstrip wires has an impact on the performance of the final product. important influence. In order to manufacture very fine copper circuits with line widths and pitches less than 1 micron, it is necessary to maintain the dielectric properties of the printed circuit board while improving the adhesion between the copper and the dielectric substrate.
In high-speed signal transmission, there are two factors that cause transmission loss due to signal propagation loss on printed circuit boards: conductor loss and dielectric (insulating material) loss (Kondo et al., 2016):
Conductor loss can be divided into scattering loss caused by surface roughness and skin effect loss (Okubo et al., 2013):
Among them, αH is the loss caused by the skin effect, and αS is the scattering loss. The skin effect is a phenomenon called the skin effect that AC signals mainly flow near the surface of the conductor, rather than the entire cross-section of the conductor. The general way to reduce conductor loss is to make the surface of the circuit smooth. However, smoothing the surface of copper circuits will weaken the adhesion between them and insulating materials. Therefore, it is difficult to manufacture a copper circuit with a smooth surface and good adhesion to insulating materials. Silica nanofillers include spherical, amorphous, fused, crystalline, and synthetic silica. Hisao Kondo et al. used uniform spherical SiO2 as a filler to make the insulating material and the substrate have a similar coefficient of thermal expansion (Kondo et al., 2016). Compared with the conductor surface that is usually micro-etched or roughened in the past, the film has a smooth surface to reduce the conductor loss.
In addition, the surface of dielectric materials can be treated by plasma treatment, ultraviolet light, γ-ray, ion radiation, and chemical modification (Baklanov et al., 2013). Polar functional groups are introduced on the surface to increase the polarity and polarity of the material surface. Surface tension can avoid the introduction of a large number of polar functional groups in the body, thereby avoiding poor dielectric properties when improving adhesion performance. Oxygen-containing polar functional groups at the interface have strong electronic polarization and atomic polarization, resulting in high dielectric constant and dielectric loss. In particular, while the interface adhesion is improved, when the peel strength of the polymer-metal interface exceeds a certain value, the failure mode of the polymer and copper foil after surface modification is essentially cohesive failure. There is no point in increasing the interface bonding at this point.
In dielectric bonded films, the adhesive force is related to the dielectric properties of the substances involved, so that the dielectric constant, conductivity, dipole moment and refractive index can also be used to determine the general adhesive tendency. IWAMOTO, NE et al. state that the adhesive force is the sum of the forces acting between metal-polymer, polymer-polymer and any intermediate layers (e.g. metal-oxide and oxide-polymer) (Iwamoto, 1995). For the general interfacial interaction energy, this can be qualitatively summarised by the following expression:
The metal-polymer interface is analogous to a capacitive circuit, where A is the surface area of the capacitor; d is the distance between the capacitor poles. ε is the dielectric constant, ρ is the resistivity, ω is the frequency, R is the electric field induced by the dipole in the interaction and μ is the dipole moment. However, it cannot be ignored that the structure and geometry of the dielectric bonding film interface, plastic deformation has an influence on the measured structure of the experiment, but this complicates the model.
Product Structure Design
Copper-clad laminates (CCL) are the dominant base material for the manufacture of PCBs. Copper-clad laminates include double-layer flexible copper-clad laminates (FCCL) made of insulating film and copper or triple-layer flexible copper-clad laminates (3-FCCL) with additional adhesive. Most of the existing commonly used high temperature resistant adhesives are epoxy resin adhesives, which are widely used as adhesives due to their strong bonding properties, chemical resistance, good insulating properties, low curing shrinkage and other excellent properties. The different molecular weights of epoxy resins affect their bonding properties because the number of epoxy groups in the molecule decreases as the average molecular weight increases; the distance between the crosslinking points then increases after curing, which leads to a reduction in crosslink density. Due to the severe stress concentration, the mechanical properties of the cured product will decrease as the crosslinking density decreases. This coupled with the fact that internal stresses are difficult to relieve as the crosslink density increases, will result in a non-uniform network structure and reduced mechanical properties. To solve this problem, some researchers have tried to blend two or more epoxy resins with different molecular weights to obtain products with medium crosslinking density.
Many add rubber elastomers to epoxy resins to overcome their brittleness, and phenolic resins and modified polyimides are receiving increasing attention as an ideal class of high temperature resistant adhesives. Polyimides can be divided into two categories, thermosetting and thermoplastic. In recent years, thermosetting PI resins have been valued and developed significantly for their excellent heat resistance. The curing process requires appropriate pressurisation to remove air bubbles due to moisture and solvent evaporation.
Lee et al. designed a three-layer composite structure of polyimide (PI) film as a core layer with low dielectric bonding layers on both sides for a high frequency bonding layer (HFBP) film with a Dk of about 2.80 and a Df of about 0.006 at 10 GHz and a peel strength of more than 1.2 kgf/cm (Lee and Du, 2016). By adjusting the thickness of the core layer, it can be used as a bonding sheet in a copper-clad laminate (CCL) It can also be used together with copper foil for the preparation of high frequency single/dual sided substrates. HFBP films offer better performance than LCP films in terms of workability and thickness tolerance.
Takashi Tasaki et al. developed solvent soluble polyimide with good heat resistance and low dielectric constant (Dk)/dissipation factor (Df) characteristics by optimising the composition ratio of aliphatic, alicyclic and aromatic groups present in the polyimide backbone (Tasaki, 2018). Using this PI binder, a low-profile copper foil and a plain PI film, a three-layer FCCL was developed which showed similar transmission losses to the LCP FCCL at frequencies less than 20 GHz.
Interfacial Bonding Damage Mechanisms
In addition to properties such as low dielectric constant, low hygroscopicity and high Tg, materials used as insulating layers in microelectronic applications are expected to exhibit high adhesive adhesion to metals used as interconnecting wires. Bond damage can occur either within the bonding layer or at the interface between the bonding layer and the surface to be bonded. The former refers to cohesive failure, where the adhesive ends up on both surfaces. The latter refers to interfacial bond failure, where the damage occurs at the interface between the bonding layer and the surface to be bonded (Barcia et al., 2004). The peel strength of the adhesive is determined by the interactions between the internal adhesive layer and the bonding interface (van der Waals interactions, electrostatic forces and hydrogen bonding) as well as the deformability and fluidity of the adhesive (mechanical coupling forces). In the case of microstrip copper conductors, the interactions during bonding originate directly from the oxide of copper and the polar groups of the polymer, and possible coordination interactions at the interface also have an impact on the bond strength.
Before introducing the mechanism of bond breakage, we first introduce four mechanisms that explain adhesion. 1) Mechanical interlocking theory: Mechanical interlocking refers to the existence of unevenness or hollows and cavities on the surface of the substrate, where the adhesive flows, diffuses, penetrates and solidifies to form something like a “hook bond,” “nail bond,” “anchor bond,” like glue being locked into a rough, unruly wood surface. 2) Diffusion theory: The molecular chains of the adhesive in close contact with the surface molecules of the substrate penetrate each other and fuse at the interface. Compared to other bonding theories, this theory does not require molecular interactions to achieve excellent bond strength, but diffusion theory is only applicable to the adhesion of compatible polymers and the bonding of thermoplastics. 3) Electronic theory: There is an electrostatic attraction between the interface of the adhesive and the substrate, i.e. positive and negative charges are attracted to each other. In this theory, the adhesion force is due to electrostatic interaction between the partially charged adhesive and the bonded object. However, the contribution of electrostatics to the adhesion force is usually small. 4) Adsorption theory: This theory of adhesion is probably the most generally accepted, where adhesives and adhered substances can adhere by forces acting between atoms in the interfacial region when sufficiently tight intermolecular contact is achieved at the interface. The interactions between the adhesive and the adhered can be divided into two categories, namely primary and secondary valence bonds. Primary valence bonds lead to chemisorption and include covalent, ionic and metallic bonds; secondary valence bonds lead to physical adsorption and include van der Waals forces, hydrogen bonds and acid-base interactions. There is debate as to which type of bond is more important for adhesion, with primary bonds having a fairly high interaction energy (15–250 kcal/mol) compared to secondary bonds, which are typically less than 10 kcal/mol. Of the primary valence bonds, ionic and metallic bonds are relatively undirected because the ions in ionic and metallic bonds are approximately spherical, whereas covalent bonds are strongly oriented because the orbitals involved are usually non-spherical. In practice, mixtures of these bond types occur frequently, and many adsorption systems should include bonding properties similar to more than one type. The theory of adsorption adhesion based on the formation of secondary valence bonds is of broad utility because secondary valence bonds have few directional constraints, are applicable to many systems, and are less dependent on surface morphology than primary valence bonds.
Spherical particles have a higher packing density and uniform stress distribution than laminated particles, which when filled into the resin increases fluidity, resulting in better mechanical and adhesive properties of CCL.
Specific Examples of Dielectric Loss Mechanisms and Signal Transmission Simulations
According to the Debye relaxation model, the real and imaginary parts of the dielectric constant can be expressed by the following equation:
The model relates the dielectric properties to the relaxation time. The relationship between
This is a form of the hemispherical diagram, often referred to as the Cole-Cole diagram (Figure 5). Where
Todd et al. used the dielectric constants of the filler component, matrix component and interphase region and their respective volume fractions to determine the effective dielectric constants of the composite system (Todd and Shi, 2005). In contrast to hybrid models such as Power-law, Maxwell-Garnett and Bruggeman, the interphase power law (IPL) model takes into account the interaction forces at the composite interface and determines the real and imaginary parts of the complex dielectric constant of the composite system.
The IPL model is applicable to any random, homogeneous dispersion of filler particles in the matrix. The model takes into account interphase permittivity, interphase volume fraction, interphase overlap and filler shape/orientation effects.
Where,
On the one hand, experiments provide an intuitive understanding of physical and chemical processes from a qualitative or quantitative perspective, and the simulation method can even further quantitatively evaluate the microstructure and properties of polymeric materials systematically (Huang et al., 2019b). Based on molecular dynamics (MD), Subramanian et al. studied the relationship between regular sequence, structure and properties of model polymers, such as polypropylene (PP) and polymethyl- methacrylate (PMMA) (Subramanian et al., 2001). By using the density functional theory (DFT), Bai et al. quantitatively analyzed the charge density and the dipole moment of PIs with two different molecular structures (Bai et al., 2000). In particular, reactive molecular dynamics (ReaxFF MD), combined with advantages of the DFT and the MD, could iteratively calculate the distribution of electrons in each atom (Van Duin et al., 2001). It could also effectively simulate interactions of both intramolecular chemical bonds and intermolecular non-bonded, and on this basis, it could study the influence of intramolecular characteristic groups on the model dipole moments. The dipole moment could directly measure the polarization of dielectrics. Xu wei Huang et al. 2014applied the ReaxFF MD simulation to the polyimide model, and calculated the effect of different phenyl sulfide contents on molecular stacking and polarization characteristics of modified polyimide by clarifying the change of the intermolecular interaction with the intermolecular distance and the electron distribution (Huang et al., 2019a).
On the basis of MD, Lina Si et al. analyzed the nanoporous amorphous silica (n-a-SiO2) with different porosities and mechanical properties of the films, as well as the interfacial adhesion strength of silica (Si et al., 2014). The relationship between the increasing in porosity of n-a-SiO2 and its decreasing on dielectric constant were predicted by using Lorentz-Lorenz, Rayleigh, Parallel model and Clausius-Mossotti equation. To study the interfacial adhesion strengths of n-a-SiO2, SiOCH films and silica, the interfacial adhesion interaction (Eint) between low-k films and silica was calculated by using the following equation:
Where Etot is the total internal energy of the low-k films/silica system, Elow-k is the energy of the individual low-k film, and Esilica is the energy of silica. In the Accelrys MD software, the unit of interaction energy is kcal/mol, where “mol” means a mole number of system molecules in the periodic condition. Therefore, the area of the interface is a mole number of interface in a single system with a thickness of 10.697 Å and the unit of the interaction energy becomes Joules per square meter (J/m2). Therefore, the following equation is used to calculate the interaction energy in simulations:
If the interaction energy is negative, there is an attractive force between the objects, such as adhesion; if it is positive, there is a repulsive force. The interfacial strength of n-a-SiO2/SiO2 decreases with the increase of the porosity, and the introduction of -CH2 groups in SiOCH improves its bonding strength.
Tao Pang et al. used furfurylamine (FU), aniline (AN) and bisphenol A to prepare furfural-based thermosetting benzoxazine resins by using toluene as a non-polar solvent (Pang et al., 2019). Based on the crosslink density (ρ) equation and Debye formula, they analyzed the influence of different ratios of FU and AN on the Tg and dielectric properties of benzoxazine resins. Compared with most polybenzoxazines whose k value normally was about 3.50, furfural-based polybenzoxazines had a lower dielectric constant (2.60, 5 GHz; 2.71, 10 GHz) and ultra-low dielectric loss (0.007, 5 GHz; 0.006 10 GHz). By increasing FU content, the cross-linking density and reactivity of polybenzoxazines were gradually improved. As the FU/AN molar ratio increased from 1:2 to 1:1, the Tg value changed from 278 to 294°C due to the increasing in cross-linking density and hydrogen bonding. However, as the FU/AN molar ratio was further increased from 1:1 to 2:1, under the condition of that the increasing in free volume induced by benzene was ignored, such a partially replacement of AN by FU not only reduced the molecular aromaticity of obtained polybenzoxazines, but also reduced the rigidity of polymer segments. Therefore, the Tg value did not increase significantly.
The elastic modulus of the crosslink density (ρ) of the thermoset material in the rubber region can be estimated by the following equation (Ran et al., 2010):
Where ρ is the number of moles of network chains per unit volume of the cured polymer,
In addition, the concentration of cross-linking per unit volume (Xdensity) can be used to define the cross-linking density, using the following semi-empirical equation (Shen et al., 2018):
In the formula, G' is the storage modulus of cured resins in the rubber platform area where the temperature is higher than glass transition temperature (Tg). In this work, G' is the modulus at absolute temperature T, which is 40°C higher than Tg. The possible cause of this phenomenon can be analyzed through the following Debye formula (Volksen et al., 2010).
Where kb is Boltzmann’s constant, N is the number density of dipoles,
In the signal transmission, the chemical structure of furan without benzene ring is conducive to the reduction of dielectric constant and dielectric loss factor. Firstly, after AN with the phenyl in high polarizability being replaced by FU, a reduction in polar functional groups occurred in polybenzoxazines, leading to the reduction of N and
Yanhui (Huang et al., 2014) conducted a two-dimensional finite element analysis on the image of on the surface of silica (Yan et al., 2014). The resulting TEM images retained the volume fraction, dispersion and geometric shape (simplifying SiO2 to an ellipse) of the filler in the matrix through the conversion algorithm.
Due to the inherent structural heterogeneity of polymers, it can be described by the superposition of Debye functions with different relaxation time:
α and β represent the relaxations of different structures in the interface region. α relaxation is the micro-Brownian motion of entire chain segment, and β relaxation is the rotation of polar groups. τi is higher than τ0 when α is relaxed, and τi is lower τ0 when β is relaxed. M, S and c respectively are tuning parameters under the corresponding relaxation obtained by matching the simulation output and the experimental data, the relaxation time and effects of dipole, ion and electronic polarization. The increase in dielectric loss at low frequencies is attributed to the α relaxation caused by the movement of polymer main chains, while the peak around 104 Hz is attributed to β relaxation caused by the movement of polar groups on side chains. It shows that the horizontal moving of Sα and Sβ in the curve reflect the change of the relaxation time of α and β in interface area, and the vertical displacement of Mα and Mβ in the curve reflect the change of the degree of polarization. The tuning parameter c reflects the change of dielectric constant over 106 Hz under effects of dipolarization, ion polarization and electronic polarization. For the tested system, S is usually less than 1 and M is greater than 1, which indicates that the chain segment at the interface has greater mobility and polarizability than that in the matrix. At the same time, the interface area of the composite may have stronger chain mobility and polarization in the frequency range measured. The change in polarization at higher frequencies may be attributed to short functional groups such as short-chain molecules (i.e. p-thiophene and ferrocene). It should be noted that only unimodal PGMA nano-dielectric material (Mα = 4) cannot reflect the change of polarization degree very well, which is caused by relatively poor fitting of its loss curve.
Furthermore, Yanhui Huang et al. used three-dimensional finite element analysis again to analyze a series of core@single-shell/double-shell nanocomposites (NP) (Figure 6) (Huang et al., 2016). In order to effectively control the thickness of each polymer shell, atom transfer radical polymerization (ATRP) was initiated on the surface of barium titanate (BaTiO3) to graft one or more acrylic polymers to form spherical nanoparticles. Then, the polymer (shell) will deform to a certain extent to fill the gaps between the spherical NPs to form a close-packed structure by direct compression. Assuming that the free polymer PMMA and PHEMA have the same imaginary part permittivity (the methacrylic acid skeleton and the relaxation mode strength of the ester group are same), the direct current conductivity of PHEMA can be collected from the fictitious permittivity spectrum of PHEMA to obtain Fitted PHEMA DC conductivity. First, the experimental dielectric spectra of free polymers without barium titanate (PMMA, PHEMA, and PANa) were used as the input dielectric constant of each polymer shell, and the geometric information was clearly captured for two-dimensional finite element simulation and simulation.
For BT@PMMA, at low BT content (16 vol% BT), the simulated dielectric spectrum of the longer graft chain matches the experimental data very well. At high BT content (40 vol% BT), the short graft chain would induce the elimination of the volume effect, and the polymer chains near the particle surface are more easily stretched and tightly packed. At this time, the filler/matrix interface interaction cannot be ignored. Compared to free polymer, this produces an interfacial region with a slower relaxation time and reduced strength. Since the simulation is assuming that the grafted polymer has the same relaxation behavior as the free polymer, the difference between the simulated dielectric spectrum and the experimental dielectric spectrum may be related to the grafted polymer having an interface area with the free polymer. As the geometry and interface are considered in the simulation, the interface relaxation behavior is clearly included in the simulated dielectric spectrum, which is different from the two-dimensional finite element analysis.
Since the grafted polymer and the free polymer have different relaxation behaviors, the dielectric spectrum of the free polymer initiated without barium titanate is decomposed into a superposition of 20 Debye relaxation functions, and the tuning parameters M, S is used as the offset factor of relaxation strength and relaxation time to inversely calculate the interface relaxation:
Similarly,
Conclusion and Perspective
The strong adhesion and excellent dielectric properties of polymer materials are often contradictory. It has been proved difficult to accurately predict the dielectric properties. Understanding the mechanism of interface adhesion failure and the mechanism of high-frequency dielectric response is an important step in the design to meet the requirements of high-speed and high-frequency signal transmission. Due to the inherent multi-variable problem of adhesion, the morphological factors of different chemical structures and the bonding strength of elements to copper will affect the results of the peeling test between copper and polymer. The method of surface modification improves the bonding strength (addition of functional groups that promote adhesion into the polymer/metal interface), which can avoid the introduction of a large number of polar groups in the body of the adhesive film, but this lacks the specificity of the groups to improve the adhesion. At the same time, the surface treatment conditions, such as the pre-curing of the polymer and the surface treatment, affect the interface roughness and mechanical strength, and ultimately determine the adhesion and dielectric properties.
As a non-polar liquid rubber, polybutadiene has a low dielectric constant and loss due to its extremely weak polarity, making it very suitable for high insulation materials. There have been studies on the modification of polybutadiene with functional groups and epoxy resins, for example, HTPB (hydroxy-terminated polybutadiene), EHTPB (epoxidized hydroxy-terminated polybutadiene), CTPB (carboxyl-terminated polybutadiene) Polybutadiene), NCOTPB (isocyanate terminated polybutadiene) to modify and toughen epoxy resin. However, the interface bonding strength between non-polar polybutadiene and copper foil is poor, and it is very challenging to improve the interface bonding strength as much as possible without damaging the dielectric properties. In terms of low dielectric constant, since the dielectric constant of the filler particles is much higher than the dielectric constant of the polymer, the filler particles are the main factor that affects the dielectric constant of the dielectric bonding film material. How to control the dielectric constant of the filler particles to achieve the low thermal expansion coefficient and low dielectric constant of the dielectric bonding film material has important theoretical significance and engineering application value.
Ceramic particles are often used to reduce the thermal expansion coefficient of the polymer matrix material and adjust the dielectric constant of the adhesive film material. Compared with layered particles, spherical particles have higher bulk density and uniform stress distribution, and can increase fluidity when filled into resin, so that CCL has better mechanical properties and adhesion properties. SiO2 is the most common surface modifier and filler used in CCL. Due to its excellent performance, many literature reports that the spherical SiO2 used in electronic equipment can improve dielectric properties, increase heat resistance, improve drilling processability and reduce costs. In addition to the molecular structure design of the polymer, attention should be paid to the influence of nano-filler particles on the dielectric properties of the composite material. The higher interface area of the nano-composite material effectively improves the polarization effect. The improvement of the chemical or physical bonding between the polymer matrix and the filler helps to reduce the conduction loss and the dielectric loss caused by the interface polarization. Therefore, the surface modification of nano-filler particles such as SiO2 can be carried out. For example, functional groups (such as γ-APS, γ-aminopropyltriethoxysilane) are introduced after treatment with the coupling agent and take advantage of the synergistic effect between different types of filler particles (such as multi-component polydisperse fillers and core-shell fillers) to give composite materials more excellent comprehensive properties. At the same time, a theoretical model of the relationship between the structure of the functional filler and the dielectric properties is established to provide new ideas for the development of high-performance dielectric materials. In addition to directly introducing nano-filler particles SiO2 (or POSS-NH2 with functional groups), tetraethoxysilane can be used to prepare multiple composite films through a sol-gel process. Through the modification and adjustment of the composite system, the performance of the hybrid multi-element composite material and its relationship with the composition could be studied, and the thermal expansion coefficient of the composite material would be optimized. Thus, its complementary effect would improve the weakness of the single or binary system.
Author Contributions
LW: article framework design and writing; JY: literature review and summary of relevant conclusions; WC: picture editing and related copyright acquisition; JZ: form editing and correction; DZ: Reference editing and correction.
Conflict of Interest
Authors LW, JY, WC, JZ, and DZ were employed by the company China Electronics Technology Group Corporation No. 38 Research Institute.
Publisher’s Note
All claims expressed in this article are solely those of the authors and do not necessarily represent those of their affiliated organizations, or those of the publisher, the editors and the reviewers. Any product that may be evaluated in this article, or claim that may be made by its manufacturer, is not guaranteed or endorsed by the publisher.
References
Anandraj, J., and Joshi, G. M. (2018). Fabrication, Performance and Applications of Integrated Nanodielectric Properties of Materials - A Review. Compos. Inter. 25 (5-7), 455–489. doi:10.1080/09276440.2017.1361717
Bai, X., Zhang, Z., Feng, J., Xie, G., and Chen, J. (2000). Studies on Charge Transfer of Polyimide Rings. Chem. J. Chin. Univ-chin 21 (9), 1455–1458.
Baklanov, M. R., De Marneffe, J.-F., Shamiryan, D., Urbanowicz, A. M., Shi, H., Rakhimova, T. V., et al. (2013). Plasma Processing of Low-K Dielectrics. J. Appl. Phys. 113 (4), 041101. doi:10.1063/1.4765297
Barcia, F. L., Soares, B. G., and Sampaio, E. (2004). Adhesive Properties of Epoxy Resin Modified by End-Functionalized Liquid Polybutadiene. J. Appl. Polym. Sci. 93 (5), 2370–2378. doi:10.1002/app.20739
Chen, J., Zeng, M., Feng, Z., Pang, T., Huang, Y., and Xu, Q. (2019). Design and Preparation of Benzoxazine Resin with High-Frequency Low Dielectric Constants and Ultralow Dielectric Losses. ACS Appl. Polym. Mater. 1 (4), 625–630. doi:10.1021/acsapm.8b00083
Chen, X., Ye, J., Yuan, L., Liang, G., and Gu, A. (2014). Multi-functional Ladderlike Polysiloxane: Synthesis, Characterization and its High Performance Flame Retarding Bismaleimide Resins with Simultaneously Improved thermal Resistance, Dimensional Stability and Dielectric Properties. J. Mater. Chem. A. 2 (20), 7491–7501. doi:10.1039/c4ta01292e
Devaraju, S., Vengatesan, M. R., Selvi, M., Song, J. K., and Alagar, M. (2013). Hyperbranched Polysiloxane-Based Diglycidyl Ether of Bisphenol a Epoxy Composite for Low K Dielectric Application. Polym. Compos. 34 (6), 904–911. doi:10.1002/pc.22496
Dhara, M. G., and Banerjee, S. (2010). Fluorinated High-Performance Polymers: Poly(arylene Ether)s and Aromatic Polyimides Containing Trifluoromethyl Groups. Prog. Polym. Sci. 35 (8), 1022–1077. doi:10.1016/j.progpolymsci.2010.04.003
Dong, J., Yang, C., Cheng, Y., Wu, T., Zhao, X., and Zhang, Q. (2017). Facile Method for Fabricating Low Dielectric Constant Polyimide Fibers with Hyperbranched Polysiloxane. J. Mater. Chem. C 5 (11), 2818–2825. doi:10.1039/c7tc00196g
Ge, M., Zhang, J., Zhao, C., Lu, C., and Du, G. (2019). Effect of Hexagonal boron Nitride on the thermal and Dielectric Properties of Polyphenylene Ether Resin for High-Frequency Copper Clad Laminates. Mater. Des. 182, 108028. doi:10.1016/j.matdes.2019.108028
He, D., Wang, Y., Song, S., Liu, S., and Deng, Y. (2017). Significantly Enhanced Dielectric Performances and High Thermal Conductivity in Poly(vinylidene Fluoride)-Based Composites Enabled by SiC@SiO2 Core-Shell Whiskers Alignment. ACS Appl. Mater. Inter. 9 (51), 44839–44846. doi:10.1021/acsami.7b14751
Huang, X., Sun, B., Zhu, Y., Li, S., and Jiang, P. (2019a). High-k Polymer Nanocomposites with 1D Filler for Dielectric and Energy Storage Applications. Prog. Mater. Sci. 100, 187–225. doi:10.1016/j.pmatsci.2018.10.003
Huang, X., Wang, J., Li, Q., Lin, J., and Wang, Z. (2019b). Impact of the Phenyl Thioether Contents on the High Frequency Dielectric Loss Characteristics of the Modified Polyimide Films. Surf. Coat. Technology 360, 205–212. doi:10.1016/j.surfcoat.2018.12.111
Huang, Y., Huang, X., Schadler, L. S., He, J., and Jiang, P. (2016). Core@Double-Shell Structured Nanocomposites: A Route to High Dielectric Constant and Low Loss Material. ACS Appl. Mater. Inter. 8 (38), 25496–25507. doi:10.1021/acsami.6b06650
Huang, Y., Krentz, T. M., Nelson, J. K., Schadler, L. S., Li, Y., Zhao, H., Brinson, L. C., Bell, M., Benicewicz, B., Wu, K., and Breneman, C. M. (2014). “Prediction of Interface Dielectric Relaxations in Bimodal brush Functionalized Epoxy Nanodielectrics by Finite Element Analysis Method,” in IEEE Conference on Electrical Insulation and Dielectric Phenomena, (Des Moines, IA: CEIDP), 748–751. doi:10.1109/CEIDP.2014.6995897
Huang, Y. Y., and Terentjev, E. M. (2010). Tailoring the Electrical Properties of Carbon Nanotube-Polymer Composites. Adv. Funct. Mater. 20 (23), 4062–4068. doi:10.1002/adfm.201000861
Iwamoto, N. E. (1995). Comparisons of Experimental Data to Indices of Adhesion Derived from Dielectric Models. Polym. Eng. Sci. 35 (22), 1811–1817. doi:10.1002/pen.760352211
Kango, S., Kalia, S., Celli, A., Njuguna, J., Habibi, Y., and Kumar, R. (2013). Surface Modification of Inorganic Nanoparticles for Development of Organic-Inorganic Nanocomposites-A Review. Prog. Polym. Sci. 38 (8), 1232–1261. doi:10.1016/j.progpolymsci.2013.02.003
Kondo, H., Yoshida, M., Kusama, M., and Teraki, S. (2016). High Performance Insulating Adhesive Film for High-Frequency Applications. Int. Conf. Electronics Packaging (Icep), 35–38. doi:10.1109/ICEP.2016.7486777
Lee, W.-C., and Du, B.-S. (2016). “A Composite Structure of High Frequency Low Dielectric Film and the Application in Flexible Printed Circuit (FPC),” in International Microsystems, Packaging, Assembly, and Circuits Technology Conference, IMPACT, Taipei, Taiwan, October 26–28, 2016 (IEEE), 389–392. doi:10.1109/IMPACT.2016.7800019
Lei, X., Chen, Y., Qiao, M., Tian, L., and Zhang, Q. (2016). Hyperbranched Polysiloxane (HBPSi)-Based Polyimide Films with Ultralow Dielectric Permittivity, Desirable Mechanical and thermal Properties. J. Mater. Chem. C 4 (11), 2134–2146. doi:10.1039/c5tc03391h
Leu, C.-M., Chang, Y.-T., and Wei, K.-H. (2003a). Synthesis and Dielectric Properties of Polyimide-Tethered Polyhedral Oligomeric Silsesquioxane (POSS) Nanocomposites via POSS-Diamine. Macromolecules 36 (24), 9122–9127. doi:10.1021/ma034743r
Leu, C.-M., Reddy, G. M., Wei, K.-H., and Shu, C.-F. (2003b). Synthesis and Dielectric Properties of Polyimide-Chain-End Tethered Polyhedral Oligomeric Silsesquioxane Nanocomposites. Chem. Mater. 15 (11), 2261–2265. doi:10.1021/cm0208408
Lewis, T. J. (1994). Nanometric Dielectrics. IEEE Trans. Dielect. Electr. Insul. 1 (5), 812–825. doi:10.1109/TDEI.2013.6451377
Li, W., Wang, M., Huang, T., Zhang, N., Yang, J., and Wang, Y. (2019). Constructing a Segregated Carbon Nanotube Network in Polyamide-Based Composites towards High Dielectric Constant and Low Loss. Mater. Lett. 245, 204–207. doi:10.1016/j.matlet.2019.03.004
Mittal, G., Rhee, K. Y., Mišković-Stanković, V., and Hui, D. (2018). Reinforcements in Multi-Scale Polymer Composites: Processing, Properties, and Applications. Composites B: Eng. 138, 122–139. doi:10.1016/j.compositesb.2017.11.028
Mohamed, M., and Kuo, S. (2019). Functional Polyimide/polyhedral Oligomeric Silsesquioxane Nanocomposites. Polymers 11 (1), 26. doi:10.3390/polym11010026
Murugaraj, P., Mainwaring, D., and Mora-Huertas, N. (2005). Dielectric Enhancement in Polymer-Nanoparticle Composites through Interphase Polarizability. J. Appl. Phys. 98 (5), 054304. doi:10.1063/1.2034654
Okubo, T., Sudo, T., Hosoi, T., Tsuyoshi, H., and Kuwako, F. (2013). “Signal Transmission Loss on Printed Circuit Board in GHz Frequency Region,” in 2013 IEEE Electrical Design of Advanced Packaging Systems Symposium, Nara, Japan, December 12–15, 2013, 112–115. doi:10.1109/EDAPS.2013.6724402\
Pang, T., Zeng, M., Feng, Z., Chen, J., Huang, Y., and Xu, Q. (2019). A Facile Method for the Preparation of Furfurylamine Based Benzoxazine Resin with High-Frequency Low Dielectric Constants and Ultra-low Dielectric Losses. J. Mater. Sci. Mater. Electron. 30 (9), 8358–8370. doi:10.1007/s10854-019-01153-y
Prateek, Thakur, V. K., and Gupta, R. K. (2016). Recent Progress on Ferroelectric Polymer-Based Nanocomposites for High Energy Density Capacitors: Synthesis, Dielectric Properties, and Future Aspects. Chem. Rev. 116 (7), 4260–4317. doi:10.1021/acs.chemrev.5b00495
Qi, H., Wang, X., Zhu, T., Li, J., Xiong, L., and Liu, F. (2019). Low Dielectric Poly(imide Siloxane) Films Enabled by a Well-Defined Disiloxane-Linked Alkyl Diamine. ACS Omega 4 (26), 22143–22151. doi:10.1021/acsomega.9b03302
Ran, Q.-c., Tian, Q., Li, C., and Gu, Y. (2010). Investigation of Processing, Thermal, and Mechanical Properties of a New Composite Matrix-Benzoxazine Containing Aldehyde Group. Polym. Adv. Technol. 21 (3), 170–176. doi:10.1002/pat.1412
Shamiryan, D., Abell, T., Iacopi, F., and Maex, K. (2004). Low-k Dielectric Materials. Mater. Today 7 (1), 34–39. doi:10.1016/S1369-7021(04)00053-7
Shen, Z. H., Wang, J. J., Jiang, J. Y., Lin, Y. H., Nan, C. W., Chen, L. Q., et al. (2018). Phase‐Field Model of Electrothermal Breakdown in Flexible High‐Temperature Nanocomposites Under Extreme Conditions. Adv. Energ. Mater. 8 (20), 1800509. doi:10.1002/aenm.201800509
Si, L., Guo, D., Xie, G., and Luo, J. (2014). Mechanical Properties and Interface Characteristics of Nanoporous Low-K Materials. ACS Appl. Mater. Inter. 6 (16), 13850–13858. doi:10.1021/am503236m
Song, N., Yao, H., Ma, T., Wang, T., Shi, K., Tian, Y., et al. (2019). Decreasing the Dielectric Constant and Water Uptake by Introducing Hydrophobic Cross-Linked Networks into Co-polyimide Films. Appl. Surf. Sci. 480, 990–997. doi:10.1016/j.apsusc.2019.02.141
Subramanian, V., Samuel Asirvatham, P., Balakrishnan, R., and Ramasami, T. (2001). Molecular Mechanics Studies on Polypropylene and Polymethylmethacrylate Polymers. Chem. Phys. Lett. 342 (5), 603–609. doi:10.1016/S0009-2614(01)00620-0
Tanaka, T., Kozako, M., Fuse, N., and Ohki, Y. (2005). Proposal of a Multi-Core Model for Polymer Nanocomposite Dielectrics. IEEE Trans. Dielect. Electr. Insul. 12 (4), 669–681. doi:10.1109/TDEI.2005.1511092
Tasaki, T. (2018). “Low Transmission Loss Polyimides Substrates: A Novel Alternative to Liquid Crystal Polymers,” in 2018 IEEE 20th Electronics Packaging Technology Conference, Singapore, December 04–07, 2018 (Irvine, CA: EPTC), 237–240. doi:10.1109/EPTC.2018.8654331
Todd, M. G., and Shi, F. G. (2005). Complex Permittivity of Composite Systems: A Comprehensive Interphase Approach. IEEE Trans. Dielect. Electr. Insul. 12 (3), 601–611. doi:10.1109/TDEI.2005.1453466
Tong, L., Lei, X., Yang, G., and Liu, X. (2019). Self-toughening and Self-Enhancement Poly(arylene Ether Nitrile) with Low Dielectric Constant by Solid Crosslinking Reaction. Polymers 11 (9), 1403. doi:10.3390/polym11091403
Van Duin, A. C. T., Dasgupta, S., Lorant, F., and Goddard, W. A. (2001). ReaxFF: A Reactive Force Field for Hydrocarbons. J. Phys. Chem. A. 105 (41), 9396–9409. doi:10.1021/jp004368u
Volksen, W., Miller, R. D., and Dubois, G. (2010). Low Dielectric Constant Materials. Chem. Rev. 110 (1), 56–110. doi:10.1021/cr9002819
Wang, H., Yuan, Y., Chi, Z., Yang, Z., Li, E., and Tang, B. (2019). Researches on Silane Coupling Agent Treated AlN Ceramic Powder and Fabrication of AlN/PTFE Composites for Microwave Substrate Applications. J. Mater. Sci. Mater. Electronmaterials Electronics 30 (22), 20189–20197. doi:10.1007/s10854-019-02402-w
Wang, J.-Y., Yang, S.-Y., Huang, Y.-L., Tien, H.-W., Chin, W.-K., and Ma, C.-C. M. (2011). Preparation and Properties of Graphene Oxide/polyimide Composite Films with Low Dielectric Constant and Ultrahigh Strength via In Situ Polymerization. J. Mater. Chem. 21 (35), 13569–13575. doi:10.1039/c1jm11766a
Wu, Z., Gao, S., Chen, L., Jiang, D., Shao, Q., Zhang, B., et al. (2017). Electrically Insulated Epoxy Nanocomposites Reinforced with Synergistic Core-Shell SiO2 @MWCNTs and Montmorillonite Bifillers. Macromol. Chem. Phys. 218 (23), 1700357. doi:10.1002/macp.201700357
Xu, N., Hu, L., Zhang, Q., Xiao, X., Yang, H., and Yu, E. (2015). Significantly Enhanced Dielectric Performance of Poly(vinylidene Fluoride-Co-Hexafluoropylene)-Based Composites Filled with Hierarchical Flower-like TiO2 Particles. ACS Appl. Mater. Inter. 7 (49), 27373–27381. doi:10.1021/acsami.5b08987
Yang, K., Huang, X., Huang, Y., Xie, L., and Jiang, P. (2013). Fluoro-Polymer@BaTiO3 Hybrid Nanoparticles Prepared via RAFT Polymerization: Toward Ferroelectric Polymer Nanocomposites with High Dielectric Constant and Low Dielectric Loss for Energy Storage Application. Chem. Mater. 25 (11), 2327–2338. doi:10.1021/cm4010486
Yang, X., Tang, L., Guo, Y., Liang, C., Zhang, Q., Kou, K., et al. (2017). Improvement of Thermal Conductivities for PPS Dielectric Nanocomposites via Incorporating NH2-POSS Functionalized nBN Fillers. Composites A: Appl. Sci. Manufacturing 101, 237–242. doi:10.1016/j.compositesa.2017.06.005
Yao, Y., Zeng, X., Guo, K., Sun, R., and Xu, J.-b. (2015). The Effect of Interfacial State on the thermal Conductivity of Functionalized Al2O3 Filled Glass Fibers Reinforced Polymer Composites. Composites Part A: Appl. Sci. Manufacturing 69, 49–55. doi:10.1016/j.compositesa.2014.10.027
Yu, P., Wang, Y., Yu, J., Zhu, J., and Hu, Z. (2018). Influence of Different Ratios of A-ODPA/a-BPDA on the Properties of Phenylethynyl Terminated Polyimide. J. Polym. Res. 25 (5), 1–11. doi:10.1007/s10965-018-1508-4
Yu, P., Zhang, Y. l., Yang, X., Pan, L. j., Dai, Z. y., Xue, M. z., et al. (2019). Synthesis and Characterization of Asymmetric Bismaleimide Oligomers with Improved Processability and Thermal/mechanical Properties. Polym. Eng. Sci. 59 (11), 2265–2272. doi:10.1002/pen.25229
Zhang, F. (2019). Study of the Effect of SiO2 Morphology and Particle Size on the Dielectric Properties of Polyolefin Composite Resins. Master's thesis. Chengdu, China: University of Electronic Science and Technology.
Zhang, X., Li, B.-W., Dong, L., Liu, H., Chen, W., Shen, Y., et al. (2018). Superior Energy Storage Performances of Polymer Nanocomposites via Modification of Filler/Polymer Interfaces. Adv. Mater. Inter. 5 (11), 1800096. doi:10.1002/admi.201800096
Zhao, B., Hamidinejad, M., Zhao, C., Li, R., Wang, S., Kazemi, Y., et al. (2019). A Versatile Foaming Platform to Fabricate Polymer/carbon Composites with High Dielectric Permittivity and Ultra-low Dielectric Loss. J. Mater. Chem. A. 7 (1), 133–140. doi:10.1039/c8ta05556d
Zhu, J., Ji, X., Yin, M., Guo, S., and Shen, J. (2017). Poly (Vinylidene Fluoride) Based Percolative Dielectrics with Tunable Coating of Polydopamine on Carbon Nanotubes: Toward High Permittivity and Low Dielectric Loss. Composites Sci. Technology 144, 79–88. doi:10.1016/j.compscitech.2017.03.017
Keywords: dielectric constant, dielectric loss, high frequency, polymer composites, carbon nanofillers
Citation: Wang L, Yang J, Cheng W, Zou J and Zhao D (2021) Progress on Polymer Composites With Low Dielectric Constant and Low Dielectric Loss for High-Frequency Signal Transmission. Front. Mater. 8:774843. doi: 10.3389/fmats.2021.774843
Received: 13 September 2021; Accepted: 28 September 2021;
Published: 19 October 2021.
Edited by:
Dong Xiang, Southwest Petroleum University, ChinaReviewed by:
Wei Zhou, Beijing Institute of Radiation Medicine, Beijing, ChinaXianlong Zhang, Sichuan University, China
Copyright © 2021 Wang, Yang, Cheng, Zou and Zhao. This is an open-access article distributed under the terms of the Creative Commons Attribution License (CC BY). The use, distribution or reproduction in other forums is permitted, provided the original author(s) and the copyright owner(s) are credited and that the original publication in this journal is cited, in accordance with accepted academic practice. No use, distribution or reproduction is permitted which does not comply with these terms.
*Correspondence: Lu Wang, NDIyNzgwMTBAcXEuY29t