- 1Nanjing Stomatological Hospital, Medical School of Nanjing University, Nanjing, China
- 2State Key Laboratory of Pharmaceutical Biotechnology, Division of Sports Medicine and Adult Reconstructive Surgery, Department of Orthopedic Surgery, Nanjing Drum Tower Hospital, The Affiliated Hospital of Nanjing University Medical School, Nanjing, China
Fusobacterium nucleatum is a typical periodontal opportunistic pathogen that contributes to inflammatory diseases at oral or extra-oral sites. The emergence of antibiotics resistance calls for a substitute therapeutic strategy to control bacterial infections effectively. In addition, macrophage is an essential part of host defense against microorganism invasion in vivo. Herein, we synthesized gold nanoclusters (AuNCs) as potent antibacterial material to kill F. nucleatum through bactericidal behavior and pro-phagocytic activity. Firstly, we evaluated the bactericidal effect of AuNCs on F. nucleatum by the means of plate counting, growth curve measurement, bacterial viability analysis, and live/dead fluorescent imaging. Morphological changes and the intracellular reactive oxygen species levels of bacteria were further analyzed to explore the possible mechanisms. Additionally, the impact of AuNCs on the ability of macrophages to uptake bacteria under normal and inflammatory circumstance was also evaluated by fluorescent imaging. We found that AuNCs exhibited superior antibacterial performance on F. nucleatum in vitro by a combination of membrane disruption and oxidative stress. Furthermore, in the presence of AuNCs, macrophages displayed enhanced phagocytosis against F. nucleatum without inducing any significant cytotoxicity. Therefore, AuNCs are providing a new platform for the prevention and treatment of F. nucleatum-associated diseases.
Introduction
Fusobacterium nucleatum, a Gram-negative anaerobic microorganism, is frequently detected in the oral microbiota of healthy or diseased humans (Brennan and Garrett, 2019). An abnormal quantity of F. nucleatum in oral cavities has long been known to cause dysbiotic microbial communities and immune response disorder, subsequently inducing infectious diseases (Hajishengallis and Lamont, 2012; Hajishengallis, 2015). In the formation of subgingival plaque, F. nucleatum functions as an important bridging microorganism that connects primary colonizers with secondary colonizers to mediate periodontal health and disease (Kolenbrander et al., 2010). Moreover, the potential relationship of F. nucleatum with systemic conditions, such as adverse pregnancy outcomes, acute appendicitis, brain abscesses, osteomyelitis, pericarditis, and gastrointestinal disease has been widely reported (Brennan and Garrett, 2019). Specifically, F. nucleatum has recently garnered attention due to its robust association with colorectal cancer and poor prognosis, although the exact mechanisms remain unclear (Brennan and Garrett, 2019; Kitamoto et al., 2020). Therefore, F. nucleatum has come to the forefront of scientific research, which may provide a target for the treatment of related diseases.
Most drugs used to treat bacterial infections are chemical antibiotics. However, the emergence of multi-drug-resistant (MDR), even extremely drug resistant (XDR), bacteria because of the overuse and misuse of traditional antibacterial drugs poses a great threat to our health (Rather et al., 2017). A novel and efficacious strategy to achieve greater antibacterial effects is urgently required. Advances in engineered nanomaterials and nanotechnologies have provided new platforms for antibacterial infections (Pelgrift and Friedman, 2013). Previous studies reported that when the size of nanoparticles (NPs) decreases to less than 2 nm, called “ultrasmall metal nanoclusters (NCs)”, they act as broad-spectrum potent killers of Gram-positive and Gram-negative bacteria (Wang et al., 2015; Chang et al., 2019; Yougbare et al., 2019; Tang et al., 2020). In particular, gold nanoclusters (AuNCs) such as Au25(SR)18 (SR denotes thiolate ligands) have sparked increasing interest due to their excellent biocompatibility, facile preparation and functionalization (Song et al., 2016). Practical applications of AuNCs and related nanomaterials have been researched extensively in many areas such as drug delivery, biological imaging, biosensing, and antibacterial agents (Zhang and Wang, 2014; Zhang et al., 2015; Zheng and Xie, 2020). In 2017, Zheng et al. demonstrated that AuNCs exerted higher antimicrobial activity on Staphylococcus aureus, Staphylococcus epidermidis, Escherichia coli and Pseudomonas aeruginosa than larger-sized gold nanoparticles via attacking membrane disruption and interfering metabolism (Zheng et al., 2017). Another study reported that AuNCs functionalized with quaternary ammonium (QA) salts exhibited antibacterial therapeutic effects against MDR bacteria in vivo without any drug resistance or detectable toxicity (Xie et al., 2018). Furthermore, AuNC-constructed metal-organic network films had strong and broad antibacterial performance against methicillin-resistant Staphylococcus aureus (MRSA) and extended-spectrum β-lactamases Escherichia coli (ESBL E. coli) for combating implant-associated infections (Chu et al., 2020). Numerous studies have linked the antibacterial behaviour of AuNCs with the abundant production of excessive reactive oxygen species (ROS), which can cause oxidative stress to lipids, DNA, and proteins, resulting in significant damage to cell structures, leakage of intracellular contents and cell death (Ahmed et al., 2018; Tian et al., 2020; Zheng et al., 2021). However, the antibacterial effect of AuNCs on F. nucleatum has not yet been well explored.
To survive in the host environment, a key strategy for pathogens is to interfere with normal cell signaling to defuse the defenses that are aimed at controlling and eliminating incoming pathogens (Rosenberger and Finlay, 2003). As the front line of immune defenses, macrophages release various pro-inflammatory cytokines, reactive oxygen and nitrogen mediators to combat invading pathogens, and recruit other immune cells to the affected area (Garlet, 2010). In particular, at the early stage of an infection, macrophages are critical participant in phagocytosing microbes from the blood stream and tissue by ligand-receptor combination and downstream signals trigger (Weiss and Schaible, 2015). Reduced phagocytic ability and impaired cytokine production of macrophages often result in compromised host defenses and prolonged infections (Kodali et al., 2013). Some studies have indicated nanomedicine may impair macrophages response to bacteria and result in bacterial infections. For instance, Aluminum NPs were found to impair phagocytosis function and repress immune response to respiratory pathogens (Braydich-Stolle et al., 2010). Increased susceptibility to lung infection was associated with exposure to nanoparticles via macrophages cytotoxicity, diminished phagocytic function and damaged innate immune responses (Kodali et al., 2013). RAW 264.7 cells pre-exposed to 10 nm SiO2 NPs showed a dramatic reduction of phagocytosis against E. coli in vitro (Bancos et al., 2015). Therefore, except for direct antibacterial efficiency, the impact of AuNCs on macrophage function is a key issue that should also be anticipated before their biological applications. However, previous researches usually focus on the interaction between AuNCs and bacteria, and the role of AuNCs in macrophages’ behavior has not been reported at present.
In our study, we first determined the antibacterial effect of AuNCs on F. nucleatum in vitro and investigated the possible mechanisms. And then the impact of AuNCs on the phagocytic ability of macrophages of F. nucleatum was further evaluated to determine the potential therapeutic applications of AuNCs in the bacterial infections.
Materials and Methods
Materials and Chemicals
All chemicals used for the synthesis of AuNCs were obtained from Sigma-Aldrich (St. Louis, MO, United States). The bacteria F. nucleatum was purchased from the Guangdong Microbial Culture Collection Center (GDMCC, 1.1290). Human periodontal ligament cells (PDLCs) were obtained from ScienCell Research Laboratories (Carlsbad, CA, United States) and RAW264.7 macrophage cells were obtained from the American Type Culture Collection.The LIVE/DEAD BacLight Bacterial Viability Kit was obtained from Invitrogen (Carlsbad, CA, United States), and the Alamar Blue Cell Viability Kit was purchased from KeyGEN BioTECH (Nanjing, Jiangsu, China). Dulbecco’s Modified Eagle Medium (DMEM) and fetal bovine serum (FBS) were obtained from Gibco (Grand Island, NY, United States). Cell Counting Kit-8 (CCK-8) was purchased from Dojindo Molecular Technologies, Inc. (Tokyo, Japan). The Reactive Oxygen Species Assay Kit, CFDA–SE (carboxyfluorescein diacetate, succinimidyl ester) fluorescent probes, and DAPI staining solution were purchased from Beyotime Biotechnology (Shanghai, China).
Synthesis and Characterization of AuNCs
AuNCs were synthesized by the sodium borohydride (NaBH4) reduction method as described elsewhere (Wang et al., 2021). Transmission electron microscopy (TEM, JEM-2100; JEOL Ltd., Tokyo, Japan), high-resolution transmission electron microscopy (HR–TEM, Tecnai G2 F20 S-TWIN; FEI Company, Hillsboro, OR, United States), ultraviolet–visible (UV–Vis) spectrophotometry (CARY 300 Bio UV–Vis Spectrophotometer; Agilent Technologies, Santa Clara, CA, United States), and dynamic light scattering (DLS, Malvern Zetasizer Nano ZS90; Malvern Panalytical, Malvern, United Kingdom) were used to characterize the AuNCs.
Bacterial Identification and Culture
F. nucleatum was cultivated on Columbia blood agar plates supplemented with hemin (5 mg L−1), yeast extract (5 g L−1), menadione (1 mg L−1) and 5% sterilized defibrinated sheep blood at 37°C in an anaerobic incubator (N2, 80%; H2, 10%; CO2, 10%). After identified using 16S ribosomal RNA gene sequencing (Novogen, Tianjin, China), a single colony was inoculated in brain heart infusion broth (BHI broth) and continuously cultured in the same condition above. During the exponential growth phase, the bacterial suspension was centrifuged at 8,000 rpm and 4°C for 5 min, washed three times with PBS and resuspended in buffer for subsequent use.
Spread Plate Method
Bacterial suspensions at concentrations of 2.5 × 106 CFU mL−1 were treated with AuNCs (0.1, 0.2, and 0.4 mM) or PBS and placed in a rotary shaker set at 200 rpm and 37°C for 6 h. Then the F. nucleatum suspension was centrifuged at 8,000 rpm for 5 min and washed three times with PBS to remove AuNCs that had not been internalized. Then the treated bacteria were resuspended in PBS and diluted, aliquots of 10 μL were plated on Columbia blood agar plates and cultured in anaerobic conditions for 48 h to allow the formation of bacterial colonies. Finally, the bacterial colonies were counted and analyzed to evaluate the killing efficiency of AuNCs.
Growth Curve Analysis
Bacteria were treated with AuNCs for 6 h as described above. Then they were resuspended in BHI broth, seeded in 96-well microtitre plates and cultured at 37°C for 30 h. The absorbance of the suspension at 600 nm was recorded every 6 h by a SpectraMax M3 (Molecular Devices, San Jose, CA, United States) to obtain the growth curves of the bacteria.
Alamar Blue Cell Viability Assay
Alamar Blue is a resazurin-based solution that indicates cell health conditions by employing the reducing power of living cells. F. nucleatum was treated with AuNCs at 37°C as described above for 6 h; then the absorbance of a solution containing Alamar Blue was measured at 570 and 600 nm. Finally, the reduction rate was calculated according to the formula:
where A570 and A600 refer to the absorbances of all sample groups after subtraction of Ao570 and Ao600 from the control group. Higher absorbance values represent a higher bacterial metabolic activity.
Live/Dead Bacterial Imaging
SYTO9 dye was used to label all bacteria with green fluorescence, while propidium iodide (PI), a cell-impermeable, red fluorerescent dye, was employed to stain only dead cells with permeable membranes. The excitation/emission (Ex/Em) maximum of SYTO9 was 480/500 nm and the excitation/emission (Ex/Em) maximum of PI was 490/635 nm. Bacteria were treated with AuNCs at 37°C for 6 h, centrifuged at 8,000 rpm for 5 min, and incubated with SYTO9/PI dyes in the dark for 15 min. Finally, 5–10 μL of the bacterial suspension was dropped on the bottom dishes and images were captured by confocal fluorescent microscopy at magnifications of 60× (NikonA1; Nikon Inc., Tokyo, Japan). The fluorescent intensity was calculated using Image J to determine the percentage of dead F. nucleatum after being treated with to AuNCs for 6 h.
Morphological Analysis of the Bacteria
F. nucleatum was treated with the AuNCs (0.2 mM) for 6 h in the same way, completely immobilized with 2.5% glutaraldehyde at 4°C overnight and dehydrated with a graded ethanol series (50, 70, 80, 90, 95, and 100%) for 15 min in each solution. Following dehydration, the bacteria were placed on cell slides, freeze-dried and observed by field emission–scanning electron microscopy (FE–SEM, S-4700; Hitachi, Tokyo, Japan). Meanwhile, another batch of samples treated in the same process were embedded in epoxy resin, cut into ultrathin sections by a microtome, and observed at 80 kV by TEM (HT7800; Hitachi) to analyze ultrastructural changes.
Levels of Intracellular ROS
DCFH-DA (6-carboxy-2′,7′-dichlorodihydrofluorescein; Molecular Probes, Eugene, OR, United States) dye was applied to test intracellular ROS levels according to the manufacturer’s instructions. Briefly, a bacterial suspension at a concentration of 106 CFU mL−1 was incubated with DCFH-DA probes for 30 min at 37°C. Then the cells were treated with the AuNCs (0.2 mM) for 2 h, with PBS as the control. Finally, the fluorescence intensity of all samples was observed by confocal fluorescence microscopy and a microplate reader under Ex/Em wavelengths of 488/525 nm.
Phagocytic Activity of Macrophages
F. nucleatum was inoculated in BHI medium and grown at 37°C overnight before incubation with CFDA–SE for 30 min to obtain fluorescent labeling F. nucleatum. Lipopolysaccharides (LPS) of E. coli at 20 ng·mL-1 was used as a proinflammatory stimulus to induce macrophages into activated condition. Macrophages were seeded at densities of 5×105 cells mL−1 in glass bottom cell culture dishes and treated with AuNCs (0.2 mM), LPS (20 ng·mL-1), or LPS (20 ng·mL-1) +AuNCs (0.2 mM) for 24 h, with medium as the control group. Subsequently, fluorescently-tagged F. nucleatum were added to the RAW264.7 macrophages at a ratio of 10:1 (bacteria to macrophages) for 2 h to allow cellular uptake to occur. Finally, samples were washed with PBS, fixed with 4% paraformaldehyde, stained with DAPI, and observed by confocal laser scanning microscopy to evaluate the efficiency of phagocytosis.
The Cytotoxicity Assessment of AuNCs
The CCK-8 assay was performed to investigate the cytotoxicity of the AuNCs toward PDLCs and RAW264.7 macrophage cells. Briefly, cells were seeded at densities of 10,000 per well in a 96-well plate and cultured in DMEM medium supplemented with heat-inactivated FBS (10%) and penicillin/streptomycin (1%) at 37°C and 5% CO2 overnight. Then AuNCs were added to the cells at final concentrations of 0.1, 0.2, and 0.4 mM and cultured for 24 h to perform the CCK-8 assay with untreated cells as the control.
All data were presented as means ± standard deviations of triplicate samples. Statistical analysis was performed using GraphPad Prism 8 (GraphPad Software, Inc., San Diego, CA, United States) using the two-tailed unpaired Student’s t test, and statistical significance was set as P< 0.05.
Results and Discussion
Characterization of AuNCs
AuNCs appeared as a reddish-brown solution (Figure 1A). The morphology and diameters of the AuNCs were analyzed by TEM and HR-TEM. AuNCs were uniformly dispersed and approximately 2–3 nm in size, as shown in Figure 1B. The UV-Vis absorption spectrum of AuNCs indicated distinct absorption peaks at 400, 450, 670, and 770 nm (Figure 1C), which was in concordance with previous research (Wang et al., 2021). The hydrodynamic size distribution of the AuNCs was approximately 7 nm confirmed by DLS (Figure 1D). These results confirmed the successful synthesis of AuNCs for the subsequent experiments.
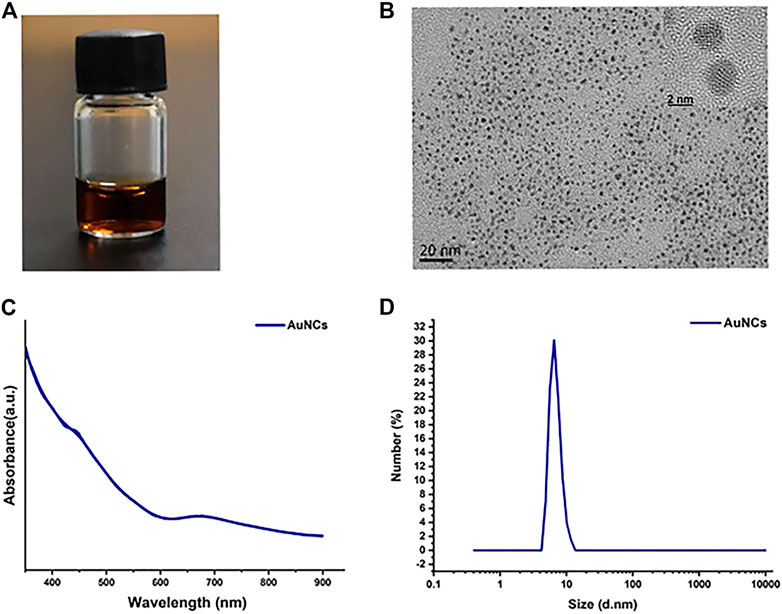
FIGURE 1. Characterization of AuNCs. (A) A photograph of AuNCs solution. (B) Transmission electron microscopic (TEM) and HR-TEM images. (C) UV-vis absorption spectra. (D) DLS size distribution.
Antibacterial Activity of AuNCs on F. Nucleatum
The antibacterial activity of AuNCs on F. nucleatum was firstly investigated by Spread Plate Method. Compared with the control, the antibacterial efficiency of AuNCs was dose-dependent with reduction rates of 91.98 ± 0.05%, 55.67 ± 0.03%, 4 ± 0.001% at concentrations of 0.1, 0.2, and 0.4 mM, respectively (Figure 2A). Bacterial growth curves showed that at the 6 h time point, bacteria reached their exponential growth phase and AuNCs’ antibacterial activity was time- and dose-dependent (Figure 2B). When the concentration was 0.2 mM, they showed remarkable antibacterial ability, especially at 6 h. The results of the Alamar Blue metabolic assay indicated that the activity of F. nucleatum was as low as 68.52 ± 10.39% and 36.60 ± 6.9% of the control at concentrations of 0.2 and 0.4 mM, respectively (Figure 2C), which was consistent with the above results. Finally, bacterial live/dead fluorescent staining assays were performed to determine the killing efficiency of AuNCs via confocal laser scanning microscopy. Figure 3A showed that bacteria treated with AuNCs exhibited higher red fluorescence intensity than that of the control group, and ∼40% F. nucleatum population membranes were effectively destroyed as illustrated in Figure 3B, indicating AuNCs exhibited potential bactericidal effect on F. nucleatum.
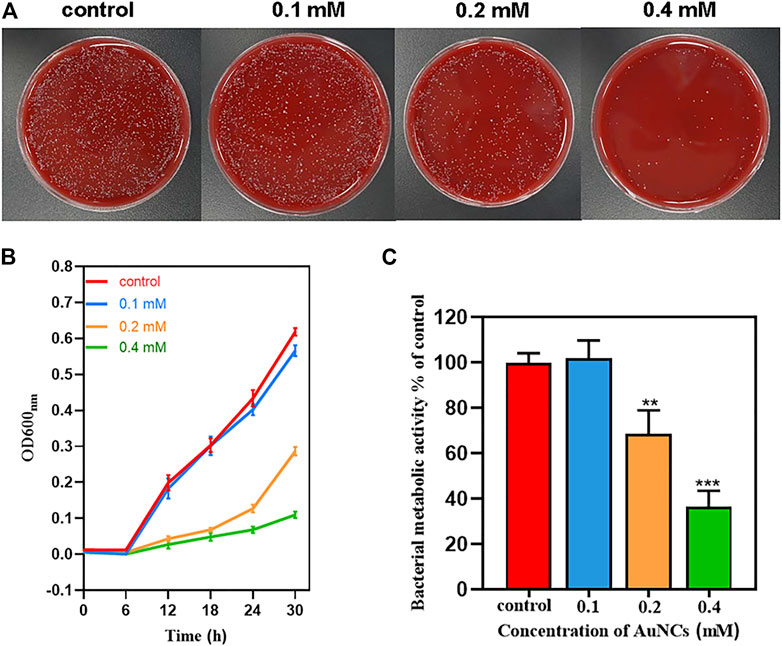
FIGURE 2. Antibacterial effects of AuNCs against F. nucleatum. (A) Growth inhibition of AuNCs (0.1, 0.2, and 0.4 mM) against F. nucleatum at 6 h on blood agar plates. (B) Growth curves of F.nucleatum exposed to AuNCs (0.1, 0.2, and 0.4 mM) for 30 h. (C) Bacterial metabolic activity of F. nucleatum exposed to AuNCs (0.1, 0.2, and 0.4 mM for 6 h (**P< 0.01, ***P< 0.001).
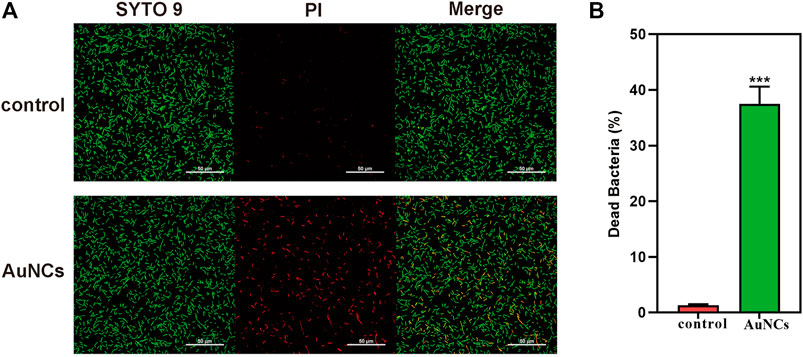
FIGURE 3. (A) Live/dead fluorescent staining images of F. nucleatum and (B) percentage of dead F. nucleatum after being exposed to AuNCs (0.2 mM) for 6 h (***P< 0.001).
Together, we observed that when the concentration of AuNCs was 0.2 mM, bacterial viability and growth was notably affected in time-dependent manner. Especially the inhibitory effect on bacterial growth was obvious when the exponential growth phase was reached at 6 h, which may be contributed to the unbalanced metabolism induced by nanomaterials treatment. Energy metabolism is necessary to the growth and survival of microorganisms. Under low metabolic activity, the bacteria are injured and their growing rate will reduce significantly, sometimes even stopping growing. Recently, Jiang and his coworkers recently found AuNCs at low concentration (100–150 μM) exhibited a potent killing activity for Gram-negative bacteria E. coli within 30–60 min (Wang et al., 2021). In a similar study, AuNCs showed half-maximal inhibitory concentrations of about 2.6 and 3.2 μM for Gram-positive (S. aureus, S. epidermidis, and B. subtilis) and Gram-negative bacteria (E. coli and P. aeruginosa), respectively, suggesting that AuNCs have a broad antibacterial spectrum (Zheng and Xie, 2020). The different results of bacterial sensitivity toward AuNCs may ascribe to their difference in structure rigid, membrane composition and metabolism activity. Compared with Gram-positive bacteria, Gram-negative bacteria exhibited higher binding efficiency with ultra-small AuNCs (Wang et al., 2021). However, Gram-negative strains F. nucleatum have shown more resistant to antibiotics or NPs than gram-positive bacteria because of the presence of outer membrane proteins (OMPs), which serve as a solid barrier to protect them from chemical drugs and harmful irritation (Bolstad et al., 1996). Furthermore, anaerobes like F. nucleatum in lower oxygen environment use other reduction-potential substrates like nitrogen as acceptor instead of oxygen, resulting in lower respiratory metabolism efficiency and less sensitivity to antibiotics than aerobic organisms (Jabłońska and Tawfik, 2019). Therefore, AuNCs may provide a new platform for the treatment of F. nucleatum associated infections.
Mechanism of Antibacterial Activity
To clarify the underlying mechanism of the AuNCs’ antibacterial activity on F. nucleatum, SEM and TEM were firstly utilized to observe the morphological changes and microstructural disruption of cells. Normally, F. nucleatum was a spindle-rod shape with an intact cell membrane and a distinct profile (indicated by a black arrow), as shown in Figure 4A. In the presence of AuNCs, the bacterial cell became obviously wrinkled and developed nano-sized pores on the membrane, suggesting increased membrane permeability (indicated by a white arrow). Moreover, the intracellular density of treated F. nucleatum was also decreased because of broken membranes and leakage of intracellular contents, as displayed by TEM in Figure 4B. In addition, intracellular ROS generation was further tested using DCFH-DA that could be oxidized to fluorescent 2′,7′-dichlorofluorescein (DCF) by oxidants. Figure 5A indicated the fluorescence intensity in the bacteria following AuNCs treatment was much higher than that of control, which was 2–2.5 folds when quantified by microreader at 488/525 nm (Figure 5B).
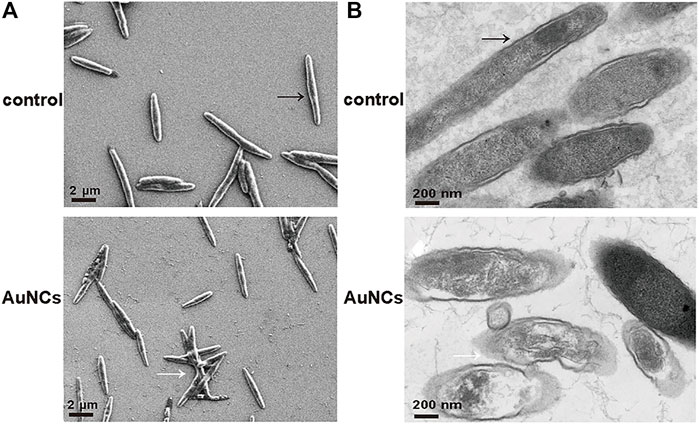
FIGURE 4. Morphological changes of F. nucleatum exposed to AuNCs (0.2 mM) for 6 h (A) SEM images and (B) TEM images.
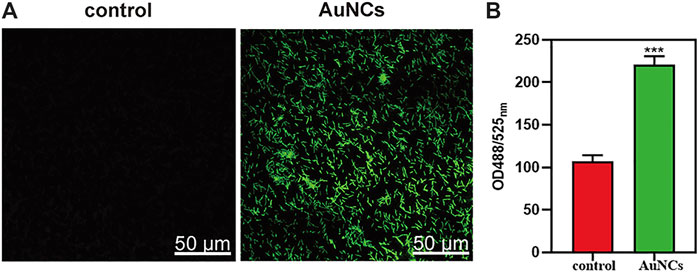
FIGURE 5. ROS levels of F. nucleatum exposed to AuNCs (0.2 mM) for 2 h. (A) Visualization of ROS concentration in F. nucleatum exposed to AuNCs by confocal microscope. (B) Quantification of ROS fluorescence intensities in F. nucleatum at OD488/525 nm. (***P< 0.001).
Based on the results, it was concluded that significant ROS augmentation may oxidize the bacterial membrane lipid efficiently and result in the large holes on the membrane, which could facilitate the further internalization and accumulation of the AuNCs inside bacteria to enhance bacteria killing efficacy. Many previous studies have explored that AuNCs can promote pro-oxidative gene expression (e.g., dmpI, narJ, and nark) and inhibit pro-reduction gene expression (e.g., sod) to cause redox imbalance (Tian et al., 2020). As a result, genes related to cell structures (e.g., DEGs), energy metabolism (e.g., glycolysis and TCA cycle) and DNA repair were significantly inhibited following AuNCs treatment (Wang et al., 2021). In a biological context, ROS represent metabolic byproducts that play an important role in cellular homeostasis. However, excess ROS generation is responsible for lipid peroxidation, protein denaturation, DNA damage, and eventually, bacterial death, which has become a strategy for treating chronic infections with heavy bacterial loads (Dryden et al., 2017). Based on this, we focused on the ROS-induction ability of AuNCs to determine their antimicrobial activity. Compared with nanoparticle, atomically precise AuNCs with more active sites also possess efficient catalytic activity that elicits excessive ROS generation for enhanced antibacterial performance (Zhang et al., 2018). Therefore, AuNCs were efficient at killing F. nucleatum by destroying bacterial membranes and enhancing ROS oxidative stress. However, details on the ROS-generating process are scarce and require further investigation.
Impact of AuNCs on the Phagocytic Ability of Macrophages
As professional phagocytes, macrophages are critical immune cells of first line of defense that response to many different infections, including bacterial and fungal pathogens (Rosowski, 2020). A canonical pathway for macrophages to control pathogens is promoting killing by phagocytosis, which involves selective macrophages receptor recognizes pathogens-associated surface molecular patterns and host-derived inflammatory mediators release (Masud et al., 2019). When microorganisms are engulfed by macrophages, they are exposed to a series of defense reactions such as protease, acidification within the phagosome and reactive oxygen stress to trigger intracellular signaling pathways and host defense (Weiss and Schaible, 2015). Phagocytosis deficiency, especially in the early stage of infectious disease, will subsequently leads to a high bacterial burden and chronic infectious diseases (Amin Yavari et al., 2020). Therefore, macrophages have represented a promising target of nanoparticle-based therapeutic interventions to successfully promote pathogen control at a time of rapidly emerging multiple drug resistance of bacteria (Amin Yavari et al., 2020). However, the impact of nanomedicines on macrophage behavior reported in many studies was contradictory. For example, Yao et al. reported CuInS2 and CdTe nanoparticles could inhibit phagocytosis of S. aureus by THP-1 and showed an immunosuppressive effect in vitro (Yao et al., 2019). In contrast, ZnO-nano films also were found to play a dual role in antimicrobial and reinforced innate defense on implant surfaced for implant-associated infections therapy (Wang et al., 2017). Furthermore, Tian and his coworkers found ZnO-nano reservoirs could enhance the anti-infective efficacy of macrophages through a combination of intrinsic antibacterial properties and reinforced host defenses (Tian et al., 2020). Iron oxide nanoparticles (IONPs) have been shown to improved macrophages-mediated bactericidal effect against Staphylococcus aureus by triggering a Fenton reaction (Yu et al., 2020). Differences in the nanomaterial (topography, charges, size, and internal architecture), experimental conditions (exposure time, concentration), cell types and solution chemistry could significantly affect their results (Rayahin and Gemeinhart, 2017; Petithory et al., 2021).
In our study, we found after AuNCs treatment, the trend of bacterial uptake ability was as follows: control<AuNCs<LPS<LPS + AuNCs, suggesting AuNCs treatment could enhance macrophages’ phagocytic ability, in particular when they were activated by LPS (Figure 6). Moderate stimuli such as LPS activate macrophages with potential damaging ability against invading pathogens (Jiang et al., 2017). In condition of low inflammation, macrophages are found to undergo necrotic death with defective bacterial killing responses. While in high inflammatory condition, macrophages are actively induced into highly phagocytic activity in ROS-mediated response (Rosowski, 2020). Insufficient ROS concentration in macrophages would contribute to a failure of bacterial clearness (Fang, 2011). Two new controllers of ROS production have been identified to targeting of pathogens by macrophages (Torraca et al., 2014). Bernut et al. recently found reduced ROS production by macrophages could inhibit their ability to control bacteria and subsequently lead to their death (Bernut et al., 2019). In contrast, Mg (OH)2 nanoparticles have been implicated in enhanced antibacterial activities of macrophages by activating the reactive oxygen species (Zhu et al., 2021). Therefore, a strategy to enhance ROS generation of macrophages and promote immunoregulatory response is a potential therapy to combat bacterial pathogens and control infections. Small-sized AuNCs have been found to possess unique properties, such as discrete energy, catalytic properties and quantum size effects (Zhang and Wang, 2014; Zhang et al., 2018). In catalytic reactions, energy transfer to oxygen molecules can induce the production of ROS, including peroxides, superoxides, hydroxyl radicals, and singlet oxygen (Li et al., 2012). And the presence of AuNCs could promote intracellular ROS induction to a higher but within normal level, subsequently enhanced the ROS-mediated anti-infective performance of macrophages (Nishimura et al., 2016; Jiang et al., 2017). However, the exact mechanisms underlying the enhanced phagocytic activity of macrophages is incompletely understood and required further research.
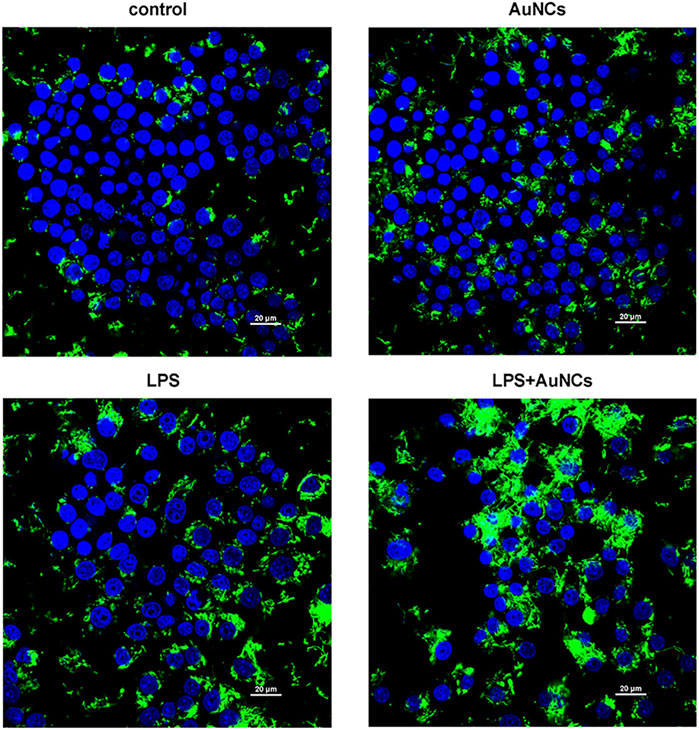
FIGURE 6. Phagocytic activity changes of macrophages (blue) to F. nucleatum (green) following AuNCs (0.2 mM) treatment under normal and inflammatory conditions.
Biocompatibility is an important factor that must be taken into consideration for the biomedical application of nanomaterials. Periodontal ligament cells are a population of heterogeneous cells that characterized by collagen production and osteoblastic features, playing an important role in the health of periodontal ligament tissues. Furthermore, they are in close proximity to bacterial invasion and antibacterial treatment (Jönsson et al., 2011). Therefore, in this study, the viability of macrophages and PDLCs after treatment with AuNCs at concentrations of 0.1, 0.2, and 0.4 mM for 24 h was evaluated. Results displayed in Figure 7A suggested that AuNCs did not negatively impact the viability of macrophages but had a slightly positive effect on the proliferation of PDLCs, although the differences were not statistically significant (Figure 7B). Previous researchers found that AuNCs could enter cells by pinocytosis, be transported to endosomes, and disposed of in lysosomes (Tian et al., 2020). However, these organelles are absent in bacteria; therefore, ultra-small nanomaterials could readily enter through physical pores by simple diffusion, eventually reaching lethal concentrations in the bacteria (Tian et al., 2020). Compared with bacteria, cellular balanced redox homeostasis is ensured by more complicated ROS generator and antioxidants system, which is of great significance in reducing the incidence of pathological dysfunction and diseases (Yang et al., 2019). Furthermore, the presence of cholesterol and the self-repair program that restores damaged structures in mammalian cells could make them more resistant to external stimuli than bacteria (Tang and Marshall, 2017; Tian et al., 2020). Therefore, AuNCs exhibited less side effect on cells than bacteria.
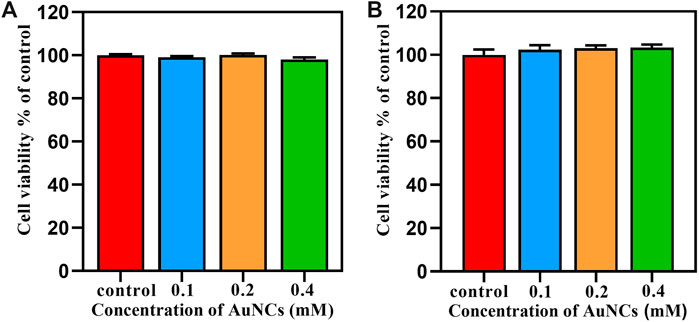
FIGURE 7. Cytocompatibility assessment of AuNCs on (A) macrophages RAW264.7 and (B) PDLCs by CCK8 at 24 h.
Conclusion
In conclusion, AuNCs were found to exhibit a potent antibacterial effect on F. nucleatum in concentration- and time-dependent manner by a combined effect of oxidative stress and membrane damage (Figure 8). Furthermore, they could also promote the phagocytic capability of macrophages with satisfying biocompatibility. Therefore, AuNCs may provide a promising alternative therapy for the prevention and treatment of F. nucleatum associated infections.
Data Availability Statement
The datasets presented in this study can be found in online repositories. The names of the repository/repositories and accession number(s) can be found below: https://www.ncbi.nlm.nih.gov/genbank/, No. OL477529.
Author Contributions
RC contributed to conceptualization, investigation, data curation, statistical analysis and wrote the original article. DQ and PW contributed to the resources and writing-reviewing and editing. LL contributed to the writing-reviewing and editing. YZ contributed to conceptualization, supervision, validation, writing-reviewing and editing. FY contributed to the design of the study, supervision, project administration, writing-reviewing and editing. All authors have reviewed, read and approved the final version of this paper.
Funding
The authors would like to thank the financial support from Natural Science Foundation of Jiangsu Province (No. BK20190133), the National Natural Science Foundation of China (No. 82101010), the Nanjing Clinical Research Center for Oral Disease (No. 2019060009).
Conflict of Interest
The authors declare that the research was conducted in the absence of any commercial or financial relationships that could be construed as a potential conflict of interest.
Publisher’s Note
All claims expressed in this article are solely those of the authors and do not necessarily represent those of their affiliated organizations, or those of the publisher, the editors, and the reviewers. Any product that may be evaluated in this article, or claim that may be made by its manufacturer, is not guaranteed or endorsed by the publisher.
References
Ahmed, B., Hashmi, A., Khan, M. S., and Musarrat, J. (2018). ROS Mediated Destruction of Cell Membrane, Growth and Biofilms of Human Bacterial Pathogens by Stable Metallic AgNPs Functionalized from bell Pepper Extract and Quercetin. Adv. Powder Technology 29 (7), 1601–1616. doi:10.1016/j.apt.2018.03.025
Amin Yavari, S., Castenmiller, S. M., Strijp, J. A. G., and Croes, M. (2020). Combating Implant Infections: Shifting Focus from Bacteria to Host. Adv. Mater. 32 (43), 2002962. doi:10.1002/adma.202002962
Bancos, S., Stevens, D. L., and Tyner, K. M. (2015). Effect of Silica and Gold Nanoparticles on Macrophage Proliferation, Activation Markers, Cytokine Production, and Phagocytosis In Vitro. Ijn 10, 183–206. doi:10.2147/IJN.S72580
Bernut, A., Dupont, C., Ogryzko, N. V., Neyret, A., Herrmann, J.-L., Floto, R. A., et al. (2019). CFTR Protects against Mycobacterium Abscessus Infection by Fine-Tuning Host Oxidative Defenses. Cell Rep. 26 (7), 1828–1840. doi:10.1016/j.celrep.2019.01.071
Bolstad, A. I., Jensen, H. B., and Bakken, V. (1996). Taxonomy, Biology, and Periodontal Aspects of Fusobacterium Nucleatum. Clin. Microbiol. Rev. 9, 55–71. doi:10.1128/cmr.9.1.55
Braydich-Stolle, L. K., Speshock, J. L., Castle, A., Smith, M., Murdock, R. C., and Hussain, S. M. (2010). Nanosized Aluminum Altered Immune Function. ACS Nano 4 (7), 3661–3670. doi:10.1021/nn9016789
Brennan, C. A., and Garrett, W. S. (2019). Fusobacterium Nucleatum - Symbiont, Opportunist and Oncobacterium. Nat. Rev. Microbiol. 17 (3), 156–166. doi:10.1038/s41579-018-0129-6
Chang, T.-K., Cheng, T.-M., Chu, H.-L., Tan, S.-H., Kuo, J.-C., Hsu, P.-H., et al. (2019). Metabolic Mechanism Investigation of Antibacterial Active Cysteine-Conjugated Gold Nanoclusters in Escherichia coli. ACS Sustainable Chem. Eng. 7 (18), 15479–15486. doi:10.1021/acssuschemeng.9b03048
Chu, G., Zhang, C., Liu, Y., Cao, Z., Wang, L., Chen, Y., et al. (2020). A Gold Nanocluster Constructed Mixed-Metal Metal-Organic Network Film for Combating Implant-Associated Infections. ACS Nano 14 (11), 15633–15645. doi:10.1021/acsnano.0c06446
Dryden, M. S., Cooke, J., Salib, R. J., Holding, R. E., Biggs, T., Salamat, A. A., et al. (2017). Reactive Oxygen: A Novel Antimicrobial Mechanism for Targeting Biofilm-Associated Infection. J. Glob. Antimicrob. Resist. 8, 186–191. doi:10.1016/j.jgar.2016.12.006
Fang, F. C. (2011). Antimicrobial Actions of Reactive Oxygen Species. mBio 2 (5), e00141–11. doi:10.1128/mBio.00141-11
Garlet, G. P. (2010). Destructive and Protective Roles of Cytokines in Periodontitis: a Re-appraisal from Host Defense and Tissue Destruction Viewpoints. J. Dent Res. 89 (12), 1349–1363. doi:10.1177/0022034510376402
Hajishengallis, G., and Lamont, R. J. (2012). Beyond the Red Complex and into More Complexity: the Polymicrobial Synergy and Dysbiosis (PSD) Model of Periodontal Disease Etiology. Microbiol. Oral Immunol. 27 (6), 409–419. doi:10.1111/j.2041-1014.2012.00663.x
Hajishengallis, G. (2015). Periodontitis: from Microbial Immune Subversion to Systemic Inflammation. Nat. Rev. Immunol. 15 (1), 30–44. doi:10.1038/nri3785
Jabłońska, J., and Tawfik, D. S. (2019). The Number and Type of Oxygen-Utilizing Enzymes Indicates Aerobic vs. Anaerobic Phenotype. Free Radic. Biol. Med. 140, 84–92. doi:10.1016/j.freeradbiomed.2019.03.031
Jiang, Y., Kou, J., Han, X., Li, X., Zhong, Z., Liu, Z., et al. (2017). ROS-dependent Activation of Autophagy through the PI3K/Akt/mTOR Pathway Is Induced by Hydroxysafflor Yellow A-Sonodynamic Therapy in THP-1 Macrophages. Oxidative Med. Cell Longevity 2017, 8519169. doi:10.1155/2017/8519169
Jönsson, D., Nebel, D., Bratthall, G., and Nilsson, B.-O. (2011). The Human Periodontal Ligament Cell: a Fibroblast-like Cell Acting as an Immune Cell. J. Periodontal Res. 46 (2), 153–157. doi:10.1111/j.1600-0765.2010.01331.x
Kitamoto, S., Nagao-Kitamoto, H., Hein, R., Schmidt, T. M., and Kamada, N. (2020). The Bacterial Connection between the Oral Cavity and the Gut Diseases. J. Dent Res. 99 (9), 1021–1029. doi:10.1177/0022034520924633
Kodali, V., Littke, M. H., Tilton, S. C., Teeguarden, J. G., Shi, L., Frevert, C. W., et al. (2013). Dysregulation of Macrophage Activation Profiles by Engineered Nanoparticles. Acs Nano 7 (8), 6997–7010. doi:10.1021/nn402145t
Kolenbrander, P. E., Palmer, R. J., Periasamy, S., and Jakubovics, N. S. (2010). Oral Multispecies Biofilm Development and the Key Role of Cell-Cell Distance. Nat. Rev. Microbiol. 8 (7), 471–480. doi:10.1038/nrmicro2381
Li, Y., Zhang, W., Niu, J., and Chen, Y. (2012). Mechanism of Photogenerated Reactive Oxygen Species and Correlation with the Antibacterial Properties of Engineered Metal-Oxide Nanoparticles. Acs Nano 6 (6), 5164–5173. doi:10.1021/nn300934k
Masud, S., Prajsnar, T. K., Torraca, V., Lamers, G. E. M., Benning, M., Van Der Vaart, M., et al. (2019). Macrophages Target Salmonella by Lc3-Associated Phagocytosis in a Systemic Infection Model. Autophagy 15 (5), 796–812. doi:10.1080/15548627.2019.1569297
Nishimura, K., Shindo, S., Movila, A., Kayal, R., Abdullah, A., Savitri, I. J., et al. (2016). TRAP-positive Osteoclast Precursors Mediate ROS/NO-dependent Bactericidal Activity via TLR4. Free Radic. Biol. Med. 97, 330–341. doi:10.1016/j.freeradbiomed.2016.06.021
Pelgrift, R. Y., and Friedman, A. J. (2013). Nanotechnology as a Therapeutic Tool to Combat Microbial Resistance. Adv. Drug Deliv. Rev. 65 (13-14), 1803–1815. doi:10.1016/j.addr.2013.07.011
Petithory, T., Pieuchot, L., Josien, L., Ponche, A., Anselme, K., Vonna, L., et al. (2021). Size-Dependent Internalization Efficiency of Macrophages from Adsorbed Nanoparticle-Based Monolayers. Nanomaterials 11 (8), 1963. doi:10.3390/nano11081963
Rather, I. A., Kim, B.-C., Bajpai, V. K., and Park, Y.-H. (2017). Self-medication and Antibiotic Resistance: Crisis, Current Challenges, and Prevention. Saudi J. Biol. Sci. 24 (4), 808–812. doi:10.1016/j.sjbs.2017.01.004
Rayahin, J. E., and Gemeinhart, R. A. (2017). Activation of Macrophages in Response to Biomaterials. Macrophages: Origin, Functions and Biointervention 62, 317–351. doi:10.1007/978-3-319-54090-0_13
Rosenberger, C. M., and Finaly, B. B. (2003). Phagocyte Sabotage: Disruption of Macrophage Signaling by Bacterial Pathogens. Nat. Rev. Mol. Cell Biol. 4 (5), 385–396. doi:10.1038/nrm1104
Rosowski, E. E. (2020). Illuminating Macrophage Contributions to Host-Pathogen Interactions In Vivo : the Power of Zebrafish. Infect. Immun. 88 (7), e00906–19. doi:10.1128/IAI.00906-19
Song, X.-R., Goswami, N., Yang, H.-H., and Xie, J. (2016). Functionalization of Metal Nanoclusters for Biomedical Applications. Analyst 141 (11), 3126–3140. doi:10.1039/c6an00773b
Tang, J., Shi, H., Ma, G., Luo, L., and Tang, Z. (2020). Ultrasmall Au and Ag Nanoclusters for Biomedical Applications: A Review. Front. Bioeng. Biotechnol. 8, 1019. doi:10.3389/fbioe.2020.01019
Tang, S. K. Y., and Marshall, W. F. (2017). Self-repairing Cells: How Single Cells Heal Membrane Ruptures and Restore Lost Structures. Science 356, 1022–1025. doi:10.1126/science.aam6496
Tian, S., Cao, Y., Chen, T., Zang, S., and Xie, J. (2020). Ligand-protected Atomically Precise Gold Nanoclusters as Model Catalysts for Oxidation Reactions. Chem. Commun. 56 (8), 1163–1174. doi:10.1039/c9cc08215h
Torraca, V., Masud, S., Spaink, H. P., and Meijer, A. H. (2014). Macrophage-pathogen Interactions in Infectious Diseases: New Therapeutic Insights from the Zebrafish Host Model. Dis. Model. Mech. 7 (7), 785–797. doi:10.1242/dmm.015594
Wang, H.-Y., Hua, X.-W., Wu, F.-G., Li, B., Liu, P., Gu, N., et al. (2015). Synthesis of Ultrastable Copper Sulfide Nanoclusters via Trapping the Reaction Intermediate: Potential Anticancer and Antibacterial Applications. ACS Appl. Mater. Inter. 7 (13), 7082–7092. doi:10.1021/acsami.5b01214
Wang, J., Zhou, H., Guo, G., Tan, J., Wang, Q., Tang, J., et al. (2017). Enhanced Anti-infective Efficacy of ZnO Nanoreservoirs through a Combination of Intrinsic Anti-biofilm Activity and Reinforced Innate Defense. ACS Appl. Mater. Inter. 9 (39), 33609–33623. doi:10.1021/acsami.7b08864
Wang, Y., Malkmes, M. J., Jiang, C., Wang, P., Zhu, L., Zhang, H., et al. (2021). Antibacterial Mechanism and Transcriptome Analysis of Ultra-small Gold Nanoclusters as an Alternative of Harmful Antibiotics against Gram-Negative Bacteria. J. Hazard. Mater. 416, 126236. doi:10.1016/j.jhazmat.2021.126236
Weiss, G., and Schaible, U. E. (2015). Macrophage Defense Mechanisms against Intracellular Bacteria. Immunol. Rev. 264, 182–203. doi:10.1111/imr.12266
Xie, Y., Liu, Y., Yang, J., Liu, Y., Hu, F., Zhu, K., et al. (2018). Gold Nanoclusters for Targeting Methicillin-Resistant Staphylococcus aureus In Vivo. Angew. Chem. Int. Ed. 57 (15), 3958–3962. doi:10.1002/anie.201712878
Yang, B., Chen, Y., and Shi, J. (2019). Reactive Oxygen Species (ROS)-Based Nanomedicine. Chem. Rev. 119 (8), 4881–4985. doi:10.1021/acs.chemrev.8b00626
Yao, C.-X., Lin, T.-Y., Su, Y.-L., Zou, H., Yan, Z.-Y., and Wu, S.-M. (2019). Inhibitory Effects of CuInS2 and CdTe Nanoparticles on Macrophage Cytokine Production and Phagocytosis In Vitro. Enzyme Microb. Technology 127, 50–57. doi:10.1016/j.enzmictec.2019.04.011
Yougbare, S., Chang, T.-K., Tan, S.-H., Kuo, J.-C., Hsu, P.-H., Su, C.-Y., et al. (2019). Antimicrobial Gold Nanoclusters: Recent Developments and Future Perspectives. Ijms 20 (12), 2924. doi:10.3390/ijms20122924
Yu, B., Wang, Z., Almutairi, L., Huang, S., and Kim, M.-H. (2020). Harnessing Iron-Oxide Nanoparticles towards the Improved Bactericidal Activity of Macrophage against Staphylococcus aureus. Nanomedicine: Nanotechnology, Biol. Med. 24, 102158. doi:10.1016/j.nano.2020.102158
Zhang, C., Li, C., Liu, Y., Zhang, J., Bao, C., Liang, S., et al. (2015). Gold Nanoclusters-Based Nanoprobes for Simultaneous Fluorescence Imaging and Targeted Photodynamic Therapy with Superior Penetration and Retention Behavior in Tumors. Adv. Funct. Mater. 25 (8), 1314–1325. doi:10.1002/adfm.201403095
Zhang, L., and Wang, E. (2014). Metal Nanoclusters: New Fluorescent Probes for Sensors and Bioimaging. Nano Today 9 (1), 132–157. doi:10.1016/j.nantod.2014.02.010
Zhang, Y., Song, P., Chen, T., Liu, X., Chen, T., Wu, Z., et al. (2018). Unique Size-dependent Nanocatalysis Revealed at the Single Atomically Precise Gold Cluster Level. Proc. Natl. Acad. Sci. USA 115 (42), 10588–10593. doi:10.1073/pnas.1805711115
Zheng, K., Setyawati, M. I., Leong, D. T., and Xie, J. (2017). Antimicrobial Gold Nanoclusters. ACS Nano 11 (7), 6904–6910. doi:10.1021/acsnano.7b02035
Zheng, K., Setyawati, M. I., Leong, D. T., and Xie, J. (2021). Overcoming Bacterial Physical Defenses with Molecule-like Ultrasmall Antimicrobial Gold Nanoclusters. Bioactive Mater. 6 (4), 941–950. doi:10.1016/j.bioactmat.2020.09.026
Zheng, K., and Xie, J. (2020). Composition-Dependent Antimicrobial Ability of Full-Spectrum AuxAg25-X Alloy Nanoclusters. ACS Nano 14 (9), 11533–11541. doi:10.1021/acsnano.0c03975
Keywords: gold nanoclusters, Fusobacterium nucleatum, antibacterial activity, reactive oxygen species, phagocytosis
Citation: Chen R, Qiao D, Wang P, Li L, Zhang Y and Yan F (2021) Gold Nanoclusters Exert Bactericidal Activity and Enhance Phagocytosis of Macrophage Mediated Killing of Fusobacterium nucleatum. Front. Mater. 8:803871. doi: 10.3389/fmats.2021.803871
Received: 28 October 2021; Accepted: 22 November 2021;
Published: 09 December 2021.
Edited by:
Yunfeng Lin, Sichuan University, ChinaReviewed by:
Tsung-Rong Kuo, Taipei Medical University, TaiwanHui Jiang, Southeast University, China
Copyright © 2021 Chen, Qiao, Wang, Li, Zhang and Yan. This is an open-access article distributed under the terms of the Creative Commons Attribution License (CC BY). The use, distribution or reproduction in other forums is permitted, provided the original author(s) and the copyright owner(s) are credited and that the original publication in this journal is cited, in accordance with accepted academic practice. No use, distribution or reproduction is permitted which does not comply with these terms.
*Correspondence: Yangheng Zhang, emhhbmd5aGRlbnRAbmp1LmVkdS5jbg==; Fuhua Yan, eWFuZmhAbmp1LmVkdS5jbg==