- 1Department of Chemical and Biological Engineering, University of Saskatchewan, Saskatoon, SK, Canada
- 2Bangladesh University of Engineering and Technology, Dhaka, Bangladesh
The excessive dependency on fossil fuel resources could be curtailed by the efficient conversion of lignocellulosic biomass. Biochar, a porous carbonaceous product synthesized exploiting thermochemical conversion pathway, could be an environment-friendly replacement of fossil fuel resources. Slow pyrolysis, a sub-class among various thermochemical conversion techniques, has gained immense popularity owing to its potential to convert biomass to biochar. Furthermore, biochar obtained as the by-product of slow pyrolysis has attracted enormous popularity due to its proven role and application in the multidisciplinary areas of engineering and environmental remediation applications. The physicochemical quality of biochar and its performance is significantly dependent on the feedstock type and pyrolysis process parameters. Therefore, further experimental research and investigations in terms of lignocellulose biomass type and pyrolytic process parameters (temperature, heating rate and reaction time) are essential to produce biochar with desired physicochemical features for effective utilization. This review presents an updated report on slow pyrolysis of lignocellulosic biomass, impact of different pyrolysis parameters and degradation pathway involved in the evolution properties of biomass. The influence of the feedstock type and lignocellulosic composition on the biochar properties are also discussed meticulously. The co-relationship between biochar yield at different pyrolysis temperatures and the development of textural properties provides valuable information for their effective utilization as a functional carbon material. Additionally, an extensive study was undertaken to collate and discuss the excellent physicochemical characteristics of biochar and summarizes the benefits of biochar application for diverse industrial purposes. Biochar is acknowledged for its excellent physicochemical properties owing to the thermal treatment and as a result its prospective diverse industrial applications such as for soil treatment, carbon sequestration, adsorbent (wastewater treatment or CO2 capture), producing activated carbon for gold recovery, energy storage and supercapacitor are summarized systematically in this review paper. For instance, biochar when applied in soil have shown improvement in soil respiration by 1.9 times. Furthermore, biochar when used to capture CO2 from flue gas stream under post-combustion scenario has demonstrated superior capture performance (2.8 mmol/g) compared to commercial activated carbon. This paper identified the knowledge gaps and outlooks in the field of the advancements of biochar from slow pyrolysis for targeted engineering applications mainly in the field of environmental remediation and energy harvesting.
Highlights
• Lignocellulose pyrolysis is viable due to its low carbon footprint at the commercial level.
• Efficient valorization of lignocellulosic biomass to biochar via slow pyrolysis.
• Parameters influencing slow pyrolysis of lignocellulosic biomass were highlighted.
• The influential pyrolytic process parameters are temperature, residence time and heating rate.
• Biochar with excellent physicochemical properties becomes suitable for diverse industrial applications
1 Introduction
With the growing global population and rapid urbanization, the demand for energy is increasing substantially, leading to a scarcity of conventional energy sources and raising the major concern of searching for new renewable and sustainable ways of energy supply (Gordon et al., 2011; Primaz et al., 2018; Mukherjee et al., 2019). Currently, fossil fuel is dominating the global energy market and is responsible for supplying a gigantic share of the worldwide energy demand (Okolie et al., 2020). The usage of fossil fuels also causes the emission of recalcitrant carbon dioxide (CO2) with the consequent influence on global warming and climate change (Lee et al., 2017). Increasing environmental concerns over the significant emission of greenhouse gases (GHGs), climate change, exhaustion of fossil fuel resources (coal, petroleum and natural gas) and rising fossil fuel prices have driven the search to develop sustainable renewable sources of energy as an alternative to non-renewable conventional fuels (Shalini et al., 2017; Wang H. et al., 2018). The optimal use of renewable energy resources and sustainable technologies can be an optional solution since it would significantly contribute to reducing the negative environmental impacts and simultaneously reduce the dependence on the use of fossil fuels (Halder et al., 2019; Mukherjee et al., 2021b). During the last 3 decades, more than half of the global research has focused on the usage of biomass as a popular sustainable energy source and has become a prominent topic. Biomass has proved to have a stimulus towards economic growth attributed to its abundant availability ease of conversion and has been projected to meet the global renewable energy demand by 40% (Anupam et al., 2016). So, the search for alternative bio-renewable sources may meet the market demand as well to reduce the damage caused by global warming. This opens new opportunities to explore the high potential and low-cost materials such as lignocellulosic biomass as alternatives for developing a carbonaceous product called biochar alongside the production of value-added biomaterials under a controlled environment.
Biochar is basically a pyrogenic carbonaceous solid material with a high degree of aromatization derived from shells, stalks, husks or grasses through suitable thermochemical and biochemical conversion methods conducted under inert atmosphere or limited presence of air (Wang K. et al., 2015; Mandal et al., 2017). It is synthesized with the intent to deliberately apply for various applications such as fuel and adsorbents in agronomic and industrial sectors owing to rich carbon and mineral content, large surface area and high energy content (Mohan et al., 2014; Daful and Chandraratne, 2020). Biochar produced under identical preparing conditions but from different precursors may have different physical and chemical properties owing to variant feedstock composition. So, the availability of low-cost, abundant source materials gives an opportunity to synthesize a wide variety of effective biochar in absorbing environmental contaminants and waste management (Yargicoglu et al., 2015).
This study has focused on summarizing the recent advances in the fundamentals of various thermochemical conversion techniques and slow pyrolysis of lignocellulose-based biomass to synthesize biochar for diverse industrial and engineering applications. Furthermore, the relationship between the influence of pyrolytic process parameters on biochar yield, physicochemical properties, and structure is also revealed based on the profound understanding of the changes in physicochemical properties of lignocellulose biomass and through an extensive literature review of different analytical techniques discussed in literatures. In this regard, a momentous investigation had been executed to propose a more effective control of biochar synthesis with its corresponding properties for targeted industrial applications. Extensive review to highlight the process parameters affecting the physicochemical properties of lignocellulose-based biochar for diverse industrial applications are imperative. Currently, there are insufficient data in the literature to draw conclusions concerning physicochemical properties of biochar and its usage in soil conditioning and treatment, carbon sequestration, contaminant removal from wastewater, carbon dioxide adsorption from flue gas stream, synthesis of activated carbon for gold recovery, energy harvesting in terms of energy storage and supercapacitor applications. Thus, it has been emphasized systematically throughout this review paper and discusses the major engineering and industrial applications of biochar. This review paper has highlighted the synergy between the synthesis procedure and applications to fill the voids and make efficient usage of biochar and to propose a strategy where it can substitute costly carbon-based adsorbents like activated carbon for environmental remediation and energy harvesting.
1.1 Lignocellulose Biomass and Lignocellulosic Composition
The lignocellulosic biomass refers to a biogenic compound containing organic and inorganic fractions that have been considered as a potential renewable source of valorization to produce value added products (Rangabhashiyam and Balasubramanian, 2019). Lignocellulose biomass comprises a complex structure due to the inherent macromolecular components like lignocellulose with smaller amounts of extractives (hydrocarbons or lipids) and ash (the inorganic component in the form of oxides and carbonates). Hence, the lignocellulose biomass type influences the pyrolytic degradation pathway and products in several ways. Firstly, it is categorized into two main classes: woody and non-woody waste biomasses (Jafri et al., 2018), and it covers a broad range of materials from the following sectors: herbaceous and agricultural residues (energy crops, crop residues or starch energy crops), forest residues (dedicated forestry or forestry by-products) and industrial wastes (agro-industrial residues, wood industry residues) (Biswas et al., 2017; Wang H. et al., 2018; Primaz et al., 2018; Pattanaik et al., 2019). Other biomass ressources include aquatic plants, animal and human biomass residues, waste from food processing industries and municipal solid wastes (Anca-Couce, 2016; Kim D. et al., 2016). The lignocellulosic biomass exhibits different characteristics depending on the nature and source of the feedstock and the different characteristics of lignocellulosic biomass mainly in terms of moisture content, ash content, calorific value, bulk density and voidage is illustrated in Figure 1. Additionally, a list of various types of lignocellulosic biomass available from different sectors as the potential source of valorization to produce biochar is summarized in Table 1. The primary advantage of lignocellulosic biomass resources is their low sulphur (S) and nitrogen (N) content and no net emissions of atmospheric CO2. Therefore, lignocellulosic biomass, encompassing primarily carbon derived from agricultural residues, forest debris or industrial waste, is gaining interest due to the abundant availability and low cost. It is also found to be an opportune resource for conversion into value-added products (biochar) and the generation of renewable energy. Secondly, as stated earlier, the hazard of climate change can be minimized due to no net emission of CO2 into the atmosphere while undergoing thermal conversion of lignocellulose biomass. No release of elevated greenhouse gases (GHGs) into the atmosphere during conversion or utilization is considered as the main driving force behind utilizing these only renewable resources for the generation of energy (Jafri et al., 2018).

TABLE 1. Lignocellulose biomass available for valorization (Cai et al., 2017).
Lignocellulosic components in biomass are classified into three major components: hemicellulose (19–25%), cellulose (32–45%) and lignin (14–26%) (Anca-Couce, 2016). As can be seen in Figure 2, the proportions of lignocellulosic components in lignocellulosic biomass vary significantly along with their connection and crystallinity. Along the cell wall, the lignocellulosic components combining element and rigid solids are scattered in a haphazard way. The lignocellulosic complex comprises cellulose as the main building block that forms a skeletal framework along the cell wall. Additionally, the internal voids are filled with the binding compounds, matrix (hemicellulose) and encrusting (lignin). Through hydrogen bonding, hemicellulose and lignin are connected to each other in the cell wall whilst lignin, and hemicellulose components are connected by both hydrogen and covalent bonds. The connections among the lignocellulosic components such as hydrogen and covalent bond have shown considerable impact on the pyrolysis characteristics and pyrolysis product distribution owing to their variation in the extent of thermal degradation during the pyrolysis process. The interest in using lignocellulosic biomass to produce biochar comes from numerous explanations. Previous research has carried on in-depth studies about the properties of biochar in terms of lignocellulosic composition derived from various biomass feedstocks such as white pine and wood residues (Wang Y. et al., 2018), palm kernel shell, empty fruit bunch, palm oil sludge (Lee et al., 2017), sugarcane straw (Halder et al., 2019), corn cob (Shariff et al., 2016), flax straw, wheat straw and saw dust (Azargohar et al., 2013), corncob, sawdust, cornstalk (Liu et al., 2014), palm kernel shell (Ma et al., 2015) as presented in Table 2. Lee et al. (2017) summarized the proximate composition (fixed carbon and moisture content), HHVs, and yields of biochar from pyrolysis of palm kernel shell (PKS) and empty fruit bunch (EFB). They reported that pyrolysis had a significant contribution in enhancing the biofuel properties of PKS and EFB derived biochar. The PKS and EFB derived biochar exhibited enhanced HHV in the range of 26.18–27.50 MJ kg−1 and fixed carbon content in the range of 53.78–59.92%, and decreased moisture content ranging from 1.03 to 2.26%. The findings reported in terms of enhancement in the physicochemical properties and improved biofuel properties after undergoing slow pyrolysis implied the candidacy of PKS and EFB for biochar synthesis for diverse industrial applications. Similarly, previous studies have shown the influence of different feedstocks on the yield and nature of feedstock impacting the physiochemical features of the biochar (Azargohar et al., 2013; Liu et al., 2014). Hence, selecting the suitable lignocellulosic biomass would depend on the understanding of the final utilization of biochar as the feedstock type influences the physicochemical properties of biochar, such as textural properties, surface functional moieties and heterogeneous structure significantly.
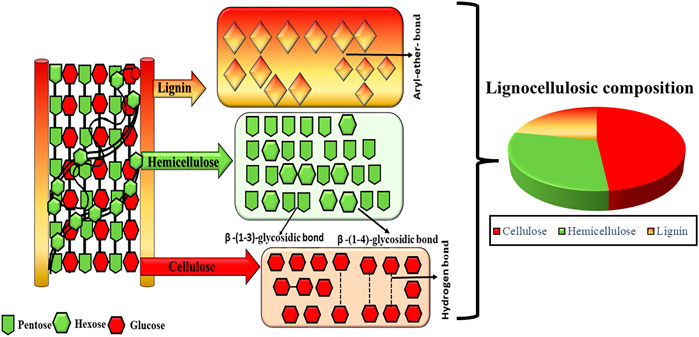
FIGURE 2. Structural representation of lignocellulosic biomass with hemicellulose, cellulose, and lignin.
1.2 Lignocellulose Biomass Degradation
The thermal degradation pathways and mechanism of lignocellulosic components (hemicellulose, cellulose, and lignin) to produce biochar is different due to differences in their composition and structure. In the pyrolysis process, cellulose undergoes depolymerization followed by several degradation pathways, including intramolecular rearrangement, dehydration, decarboxylation, aromatization, and intermolecular condensation between 315 and 400°C to produce biochar. Correspondingly, the degradation mechanism of hemicellulose follows the similar degradation pathway of cellulose. The thermal degradation of hemicellulose produces biochar and decomposes to low-molecular weight compounds (bio-oil and synthetic-gas) in the temperature ranging from 220 to 315°C. However, the lignin has a highly aromatic structure, and its decomposition is a complex mechanism (160–900°C) unlike hemicellulose and cellulose. In this regard, the degradation pathway is mainly dominated by the free radical reaction pathway (Zhang et al., 2019). Cellulose and hemicellulose contribute towards the synthesis of volatile products during the pyrolysis process. Whereas lignin is the major biochar precursor, which contributes towards the enhanced biochar yield and produces biochar with different physicochemical properties during the pyrolysis process. For instance, biochar yield obtained from woody biomass is 19 wt. % (900°C and 64 min) (Solar et al., 2016), whereas the biochar yield obtained from lignin reached up to 45.69% (Farrokh et al., 2018). To generate biochar with enhanced physicochemical characteristics such as high fixed carbon content (FC), surface area (SBET) and fine aromatic structure that are essential for diverse applications, residual biomass feedstock containing high lignin content is highly desirable for thermochemical conversion process like pyrolysis (Wang et al., 2020). A schematic pattern representing the lignocellulosic and organic extractives degradation pathway and generation of desired and by-products during the pyrolysis process is shown in Figure 3. Figure 3 clearly illustrates that every component in lignocellulose biomass degrades independently at various range of temperatures and generate desired products and by-products. The selection of a particular biomass feedstock may have a momentous impact on the total yield of biochar, physicochemical properties of biochar along with on the overall economic feasibility and viability of the pyrolysis process.
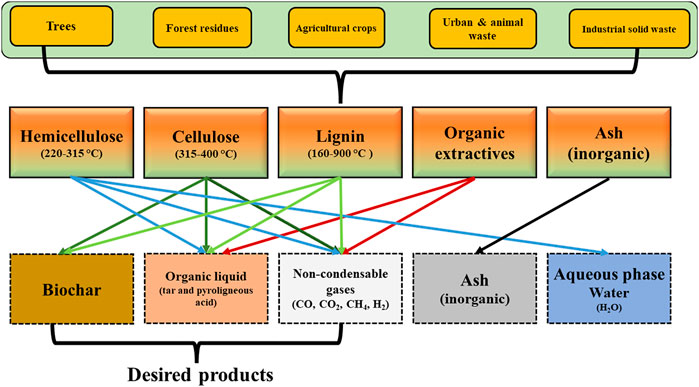
FIGURE 3. Lignocellulosic biomass degradation during pyrolysis (adapted and redrawn from Zhang et al., 2019).
2 Preparation of Biochar
In the past few years, many biomass conversion processing facilities and valorization pathways have been proposed and developed. The biomass conversion can occur through several alternative technologies such as: thermochemical conversion techniques (torrefaction, pyrolysis, hydrothermal liquefaction, hydrothermal combustion or gasification), biochemical conversion methods using bacteria or enzymes (anaerobic digestion, aerobic digestion or sugar fermentation) and physicochemical method such as lipid extraction (Anca-Couce, 2016; Wang H. et al., 2018). As a significant thermochemical conversion pathway, pyrolysis has gained immense attention from the scientific community and policymakers owing to its ease in valorizing residual biomass from environmental and economical perspective. Biochar can be derived as a low cost, carbon-rich efficient solid residue with excellent porous and heterogeneous structure by adopting slow pyrolysis as one of the significant thermochemical conversion strategies and hence is considered for the study in this review paper.
2.1 Thermochemical Conversion of Lignocellulosic Biomass
Valorization of lignocellulosic biomass through various thermochemical conversion techniques include torrefaction, hydrothermal liquefaction (HTL), hydrothermal carbonization (HTC), pyrolysis and gasification. Thermochemical conversion techniques uses heat (temperature of various range), pressure (atmospheric to high) and chemical processes under inert ambience or limited supply of oxygen to thermally breakdown the bonds of residual biomass to generate biochar and other value-added intermediates (liquid, gases and aqueous phase) through various degradation pathway as discussed earlier in section 1.2 (Kambo and Dutta 2015; Pattnaik et al., 2021; Patra et al., 2022). The desired products obtained in various phases (solid, liquid, and gas) from different categories of thermochemical conversion techniques at varying range of temperatures is highlighted in Figure 4.
An irreversible change in the physical state and chemical composition of the lignocellulosic components occurs after the thermochemical conversion in the residual biomass owing to the changes in the structural building blocks and binding compounds of biomass (lignocellulosic components). Thermochemical conversion pathway is referred to as a carbon-neutral pathway because the amount of CO2 emitted during the conversion process and the amount of CO2 consumed by the biomass during its growth period are same (Jafri et al., 2018). Amidst the variant thermochemical techniques available, slow pyrolysis has emanated as a front line research and grabbed attention for the generation of biochar with desired properties from lignocellulosic biomass. It can act as a sustainable waste-management strategy that would find way to explore the usage of residual biomass as the potential precursor, which are generally managed improperly, discarded in landfills and are unsuitable for any conventional usage. Pyrolysis decomposes thermally weak lignocellulosic constituents of biomass, and produce solid (biochar) as the desired product, a pyrolytic liquid (bio-oil), and non-condensable gas fraction (synthetic gas) under heat and inert atmospheric conditions as depicted in the following Eq. 1:
2.2 Pyrolysis
Pyrolysis of lignocellulosic biomass is a complex endothermic degradation process, which includes the thermal degradation of organic matter of the biomass mostly into solids and liquids when heated in an unsusceptible environment, i.e., in the absence of air/oxygen and atmospheric pressure condition. In case of lignocellulosic biomass, the long-chain hydrocarbons present in it are decomposed into smaller compounds under suitable pyrolysis conditions. Furthermore, the rate of thermal degradation and degree of decomposition of residual biomass is dependent significantly on the nature and composition of the feedstock and the pyrolytic process parameters mainly temperature (°C/K), residence time (s or min) and heating rate (°C s−1 or °C min−1). Based on the range of operating pyrolytic parameters mainly heating rate (°C s−1 or °C min−1). and residence time (s or min), the biomass pyrolysis process can be subdivided into the following subclasses: slow (conventional), intermediate, and fast pyrolysis techniques. The thermal degradation of biomass during pyrolysis can be described by three pathways: char formation, depolymerization reactions and fragmentation of the lignocellulosic components (Daful and Chandraratne, 2020). The yield of pyrolysis products (biochar, bio-oil, and synthetic gas) will depend on pyrolysis conditions and parameters chosen. The operating conditions (temperature, heating rate and residence time) of each category of pyrolysis is highlighted in Table 3. Correspondingly, the associated product distribution due to the thermal degradation of lignocellulosic content from each category of pyrolysis is illustrated in Figure 5. It shows the degradation of lignocellulosic components (hemicellulose, cellulose, and lignin), organic extractives and ash to produce product of different distribution.

TABLE 3. Thermochemical conversion technique (pyrolysis) and products (desired and by-products) (Wang et al., 2020; Kazawadi et al., 2021).
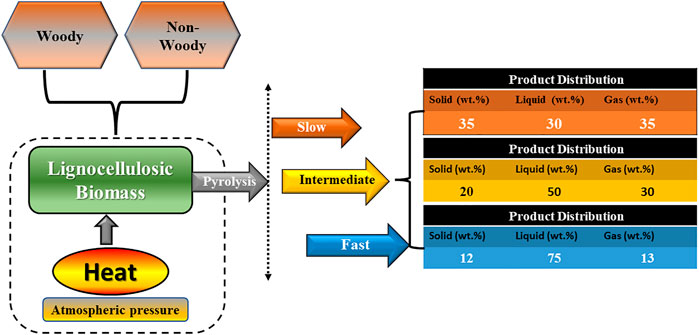
FIGURE 5. Product distribution from different categories of pyrolysis (adapted and redrawn from Meyer et al., 2011; Qambrani et al., 2017).
2.2.1 Slow Pyrolysis
Slow pyrolysis of lignocellulosic biomass feedstock is usually categorized by the slow heating rate in the range of <0.01–2°C s-1 at a relatively moderate temperature (300°C-700 C) in absence of the oxygen atmosphere and have longer-vapor residence time (minutes-days) (Qambrani et al., 2017). Owing to moderate temperature and longer residence time, secondary cracking level of biomass feedstock increases. The product distribution in slow pyrolysis is approximately equal in composition as shown in Figure 5 (Zhang et al., 2019). Biochar is obtained as the main product from this process and its yield and quality is significantly affected mainly by the temperature (°C) maintained during the pyrolysis process. In this process, the longer residence time (minutes to days) results in a wholly pyrolyzed biochar product with highly stable and recalcitrance form of the Carbon (C) which does not undergo microbial degradation for a longer duration. According to Bruun et al. (2012), biochar synthesized through slow pyrolysis has considerably shown to contain high carbon (69.6 wt%) compared to that obtained from the fast pyrolysis technique (49.3%) from the similar starting material. In slow pyrolysis, the heating rate is low, and biomass feedstocks undergo endothermic degradation at a slower rate with no heat and mass transfer limitations and consequently gives higher yield of biochar and lower yield of bio-oil and synthetic gas. It also generates heat during the thermal degradation of lignocellulosic components, which could be further recycled within the conversion unit to produce electricity or generate energy. Additionally, large particle size, high lignin and ash content in lignocellulosic biomass favors the relatively high yield of biochar during slow pyrolysis (Mohanty et al., 2013). Thus, biochar production potential and chemical compositions are affected by the feedstock composition, reaction environment and operating parameters during the pyrolysis of biomass. Compared to fast and intermediate pyrolysis techniques, slow pyrolysis has been reported to be a flexible thermochemical conversion technique mainly in terms of obtaining biochar as the main product with enhanced physicochemical properties. Accordingly, the slow pyrolysis technology is currently the most used commercial technology and a sustainable route for valorization of lignocellulosic biomass from environmental perspective to synthesize biochar owing to its higher biochar yield and fewer associated technological drawbacks. The product distribution (solid, liquid, and gaseous fraction) from three different pyrolysis processes is demonstrated in Figure 5 and the parameters affecting the product distribution synthesized from slow pyrolysis is emphasized further in section 3.0.
2.2.2 Intermediate Pyrolysis
The intermediate pyrolysis is the pyrolysis technique that lies between fast and slow pyrolysis process in terms of residence time and heating rate as shown in Table 3. In intermediate mode of pyrolysis, the rate of decomposition is faster compared to the slow pyrolysis technique but slower than the fast pyrolysis and could be attributed to the intermediate heating rate and moderate residence time. From Figure 5, it can be observed that intermediate pyrolysis has a good range of product distribution and hence can be used in the co-production of biochar, bio-oil, and syngas. However, the biochar yield from intermediate pyrolysis is less compared to slow pyrolysis technique as can be seen from the product distribution in Figure 5. The lower yield of biochar from intermediate pyrolysis compared to slow pyrolysis could be attributed to the higher heating rate maintained at the temperature range of 450–550°C. As intermediate pyrolysis occurs at controlled heating rate the generation of high molecular weight tar is inhibited with the formation of bio-oil and synthetic gas. In intermediate pyrolysis process the particle size and shape of the precursor has least impact compared to that in the fast pyrolysis technique.
2.2.3 Fast Pyrolysis
Fast pyrolysis is mainly characterized by thermal degradation at a very high heating rate of more than 2°C s−1, shorter residence times (<2 s) at a moderate to a higher temperature in the range of 500–1000°C, and consequently experience rapid decomposition of lignocellulosic constituents and organic matter during the thermal treatment. The conversion provides high bio-oil yield followed by a low yield of syngas and biochar. The desired product obtained from the fast pyrolysis is bio-oil, that could be used as the energy carrier in the industrial sectors. Furthermore, the biochar obtained from fast pyrolysis process consists of recalcitrant conjugated aromatic structures. Mohanty et al. (2013) compared and reported the effects of heating rate and residence time in terms of the production and quality of biochar exploring slow and fast pyrolysis techniques. They observed that at a slower heating rate of less than 10°C min−1 and minimum residence time of 30 min, the biochar yield was high and was in the range of 41–44 wt% as well as bio-oil yield was in the range of 18–24 wt%. Whereas in fast pyrolysis process with a very high heating rate of 450°C min−1 and minimum residence time of 30 s, the biochar yield reduced to almost half compared to that obtained during the slow pyrolysis, and the yield reported was in the range of 21–24 wt%. In fast pyrolysis, to suppress the gas production and maximize the liquid product yield, the residence time is controlled, and rapid cooling is employed for secondary cracking. In general, liquid product (bio-oil) is obtained as the desired product from the fast pyrolysis process, whereas biochar and synthetic gas are obtained as the by-products as summarized in Table 3.
3 Effects of Pyrolytic Process Parameters on Biochar Yield
The feedstock composition and the process parameters during the thermochemical conversion process significantly impacts the total yield of biochar, physicochemical properties and finally affects its end applications. Although, a considerable amount of research is imperative to establish a co-relationship between the thermochemical operating parameters with the total yield of biochar, its physicochemical properties, and final diverse applications. Hence, it led to considerable amount of research in recent times in terms of optimizing the thermochemical conversion process to maximize the biochar yield and enhance the physicochemical properties of biochar that can further be explored for various engineering applications (Chen et al., 2015; González et al., 2017; Tomczyk et al., 2020). The dominant factors that influence the product distribution and heterogeneous structure of the engineered biochar obtained from slow pyrolysis mainly include the pyrolysis temperature (°C), residence time (min) and heating rate (°C min−1). All the parameters significantly influencing the performance, product distribution mainly biochar and physicochemical properties of biochar generated from the slow pyrolysis process is highlighted in the following sections.
3.1 Effects of Pyrolysis Temperature
The temperature has a momentous impact on the product distribution and properties of biochar derived from slow pyrolysis. In general, the peak-concentration of the biochar is obtained at the lower range of pyrolysis temperature (400–500°C) and then declines with increasing the peak temperature owing to the further secondary cracking and other parallel reactions that produces more intermediate products from pyrolysis (bio-oil and synthetic gas). The physicochemical characteristics such as textural properties, calorific value, carbon content and aromatic structure depends on the pyrolysis temperature and biochar synthesized from different lignocellulosic biomass have been investigated in the past. The recalcitrant carbon content and the aromatic content enhances with increasing the pyrolysis temperature owing to the release of volatiles and aliphatic constituents. In case of slow pyrolysis, the feedstock properties and pyrolysis temperatures have a momentous impact on the total yield of biochar. Vieira et al. (2020)., investigated the impact of different pyrolytic process parameters on biochar yield undergoing slow pyrolysis of rice husk. They investigated the impact of different parameters and their interactions on the total biochar yield using Taguchi’s method and from the ANOVA results they revealed that temperature was the most important parameter influencing the yield of biochar. They obtained highest biochar yield of 37.71 wt% at 300°C. Ferjani et al. (2019)., investigated the effect of pyrolysis temperatures, ranging from 300 °C to 700 C on exhausted grape marc (EGM) on the biochar yield and physicochemical changes after undergoing the thermal conversion process. They found that at 300 °C and 400°C, the biochar yield obtained were highest and were in the range of 65.8 %-59.1%, respectively. However, they also reported the declining trend of biochar yield with increasing temperature from 500°C to 700 C. The biochar yield at that higher range of temperature were relatively less (33.8–30.9 wt%). Similarly, regarding the influence of both pyrolysis temperatures as well as heating rates on the pyrolysis products yield (biochar, bio-oil, and gas), Aysu and Küçük, (2014) investigated the effects of pyrolysis temperatures in the range of 300–600°C (with and without catalyst) at three different heating rates (15, 30, 50 C min−1). They reported that among the two parameters investigated, pyrolysis temperature is a crucial factor influencing the conversion of F. orientalis L. Among all the parameters examined, pyrolysis temperature has more pronounced effects on the bio-char yield and a decreasing trend is observed with increasing the temperature from 300 to 600 C. The range of biochar yield reported is between 40.26 and 23.38%. Also, the increasing heating rate had a detrimental impact on the biochar yield and was observed that the highest yield was obtained at 15°C min−1. Biswas et al. (2017) studied the impact of pyrolysis temperatures on different conventional agricultural residues namely wheat straw, corncob, rice straw and rice husk. They investigated the impact of different feedstock type on the total yield of biochar and other intermediate by-products at different pyrolysis temperatures ranging from 300–450°C at 50°C interval. The maximum biochar yield obtained from different agricultural residues were in the range of 33.0–43.3 wt%, respectively. They observed that irrespective of the agricultural residues, the biochar yield showed a decreasing trend as the temperature increased from 300 to 450°C. In this regard, Shalini et al. (2017)., compared the total biochar yield obtained from Coccus nucifera shells (CNS) and Prosopis glandulosa hard wood (PGH) by undergoing slow pyrolysis at 400–450°C. They also reported a similar observation of declining trend with increasing temperature, although the observation was not as pronounced as the previously reported values owing to the lower range of temperature under investigation. In this regard, they reported that the biochar yield reduced slightly from 28 wt% to 25 wt%. In general, the phenomena of declining biochar yield, and increasing bio-oil or synthetic gas yield with increasing pyrolysis temperature could be attributed to the destruction of lignocellulosic components mainly hemicellulose and cellulose followed by organic extractives (Figure 3) that are combusted at higher pyrolysis temperatures. Correspondingly, the primary decomposition is also more pronounced at higher temperatures. However, increasing the intensity of pyrolysis temperature during the pyrolysis process is necessary for improving the physicochemical properties of biochar since more volatile compounds are released from biochar, increasing its recalcitrant carbon (C) content and it also simultaneously enhances the textural properties of biochar as more porous network is formed at higher temperature due to the release of tars from the pores. In this regard, Al-Wabel et al. (2013)., reported biochar derived from conocarpus wastes synthesized at higher temperature (800 C) demonstrated increased carbon stability compared to that obtained at lower pyrolysis temperature (200°C). Similarly, Anupam et al. (2016) reported a sharp increment in surface area from 2.567 to 220.989 m2/g with increasing the temperature to 550°C from 350°C. Additionally, the pH in solution, calorific value (HHV), textural properties mainly specific surface area (BET) porosity and basic functional groups are also positively correlated with the elevated pyrolysis temperature and this enhancement in physicochemical properties are essential especially from diverse industrial applications perspective (Uroić Štefanko and Leszczynska, 2020).
3.2 Effects of Residence Time
In case of slow pyrolysis technique, the residence time range from few minutes to days. Along with the influence of biomass nature and pyrolysis temperature, the influence of residence time on biochar yield has also been confirmed as significant through several investigations (Xu et al., 2015). During slow pyrolysis, the prolonged residence time at moderate to higher ranging peak temperature plays a vital role in the pyrolysis efficiency, particularly when the heat transfer limit at this point of elevated thermal conditions is significant. Higher biochar yield obtained at maintaining moderate to prolonged residence time owing to the series of polymerization and secondary reactions that can take place for the constituents of biomass (Patra et al., 2021c). Furthermore, the prolonged residence time also has an enormous effect on the textural characteristics of biochar mainly in terms of specific surface area and pores-distribution and it could be attributed to the structural melting sintering and shrinkage of char that occurs during time of thermochemical conversion process. However, the residence time needs to be investigated for feedstock to reveal the optimized biochar production conditions. Anupam et al. (2016)., studied and reported the impact of pyrolysis process parameters in terms of temperature and processing time using five level central composite design (CCD) and numerical techniques on biochar yield and physicochemical properties derived from Leucenaena leucocephala bark. The optimum biochar yield of 47.29% was obtained at 367.47°C and at 135.38 min. They reported the existence of strong interaction between the pyrolytic process parameters. Although, pyrolysis temperature was more influential (0.91) compared to pyrolysis time (0.63) in terms of desirability. In a similar study, Liu et al. (2014), reported the effect of pyrolytic parameters on the biochar yield and physicochemical properties of corn cob derived biochar and compared with corn stalk and sawdust. They revealed that the biochar yield decreased with increasing residence time from 30 to 90 min at the same pyrolysis temperature ranging from 400–600°C. The yield reduces significantly when the pyrolysis temperature increased to 600 C and residence time to 90 min. Consequently, a moderate length of residence time is essential to optimize the biochar production condition both in perspective of yield and physicochemical properties.
3.3 Effects of Heating Rate
The biochar yield, release of volatile compounds and the characteristic of biochar is affected by the rate of change of heat during slow pyrolysis of biomass. Maintaining the pyrolysis heating rate to lower range reduces the chances of secondary pyrolysis reactions and further thermal cracking and considerably contributing to higher yield of biochar. When the heating rate of the biochar is maintained below 10°C min−1, the volatile compound formation is inhibited followed by slight rearrangement reactions of the polymer structure giving rise to stable carbon matrix. Additionally, the extent of heating rate also influences the textural properties of biochar through heat and mass transfer limitations (Mukherjee et al., 2021a). Wang H. et al. (2018) reported that under identical conditions of slow pyrolysis of white pines but at different heating rates have an impact on the product distribution. They observed that raising the heating rate from 2.3°C min−1–9.2°C min−1 the yield of char showed a decreasing trend (from 31.09% to 27.37%). Increment in biochar yield can also be made by reducing the heating rate which is a means to provide sufficient heat conduction and retainment of more volatile compounds in the fixed carbon structure are fixed and retained as the biochar (Russell et al., 2017; Wang et al., 2020). Moreover, increasing heating rate led to a decrease in biochar yield and the effect is more pronounced at higher pyrolysis temperature compared to the lower pyrolysis temperature. Furthermore, change in heating rate affects the elemental constituent of biochar and a similar finding was reported by Mohanty et al. (2013). They examined two different heating rates (2 and 450°C min −1) and observed that biochar synthesized at the lower heating rate presented higher alkaline concentration compared to higher heating rates owing to the retainment of minerals at lower heating rate. Figure 6 recommends the favorable pyrolytic conditions in terms of the highest biochar yield. Consequently, to have a balance between the total yield of biochar and the physicochemical properties, maintaining a moderate range of temperature, moderate to prolonged residence time and slower heating rate during the pyrolysis process is essential to ultimately exploit the excellent properties of biochar for diverse industrial applications.
3.4 Effects of Pyrolysis Process Conditions on Biochar Yield Derived From Food-Waste
Figures 7A–C shows the impact of pyrolytic parameters namely temperature, reaction time and heating rate on the biochar yield derived from food waste (Patra et al., 2021a). Patra et al. (2021a) used different kinds of food waste for slow pyrolysis and investigated process parameters like temperature, heating rate and residence time to enhance the biochar yield and quality. The authors used a mixture of waste fruits and vegetables such as carrot peel, pumpkin seeds, bell pepper, potato and onion peel, orange peel, watermelon shell celery head and leave, and pistachio shells. Authors enlightened the effects of process parameters (Figures 7A–C) on biochar yield and found the temperature as a key parameter with maximum influence, followed by heating rate and residence time. The biochar yield was massively reduced from 52.4 wt% to 31 wt% with the rise in the intensity of temperature from 300 to 600°C (Figure 7A). The probable reason behind the decrease in food waste biochar yield with increasing temperature is that higher temperature allows the thermal cracking of heavy hydrocarbon materials, which leads to the increase in liquid and gaseous products.
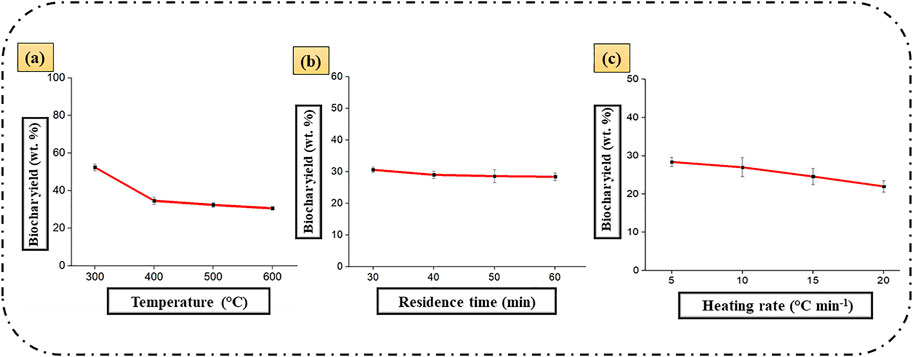
FIGURE 7. (A-C): Effect of pyrolysis temperature, residence time and heating rate on biochar yield (wt%) derived from food wastes (redrawn with permission from Patra et al., 2021a).
The residence time is also considered as a key factor, which affects the yield and chemical structure of the produced product in the pyrolytic conversion of biomass to biochar. As compared to the process temperature, the overall impact of the reaction time is considerably lower, but it still affects the percent yield of the biochar to some extent. In this experimental study, it was observed that the high quantity of biochar was produced at a low residence time that was 30 min and higher temperature that was 600°C. The biochar production was decreased from 29.9 to 28.3% product by increasing reaction time from 30 to 60 min, which was reflected in Figure 7B. In this study, the highest biochar yield of 52 wt% was achieved at lower pyrolysis temperature (300 C), slower heating rate (5 C min−1) and moderate residence time of 30 min, however the biochar possessed lower carbon content, calorific value (HHV) and surface area. However, the optimum conditions for biochar production with high carbon content of 60.7 wt%, calorific value of 23 MJ kg−1 was obtained at 600°C, 5°C min−1 and 60 min and the corresponding biochar yield recorded was 28 wt%.
Figure 7C demonstrates the overall impact of heating rate on the biochar yield derived from food waste. From the study, it was found that at a low heating rate ranging from 5–20°C min−1, the biochar yield was maximum, whereas the temperature and residence time was maintained at 600 C and 60 min, respectively. At a lower heating rate, secondary pyrolysis reactions get reduced while in higher heating rate, it backs the biomass fragmentation, which increases the bio-oil and gaseous product yield by limiting the formation of biochar. At a higher heating rate, it is likely to improve the depolymerization of biomass into volatile primary components, which at the end retards the biochar yield.
4 Analytical Methods
The physicochemical properties of biochar can vary with feedstock type and synthesis conditions. Simultaneously, it is challenging to utilize optimized biochar for soil conditioning, carbon sequestration, adsorption, and energy storage applications. In terms of the potential industrial applications as well as to examine the impact of pyrolytic parameters on the physiochemical changes in biochar, characterization of the physicochemical properties of engineered biochar is momentous (Zhao et al., 2013; Wilk and Magdziarz 2017). Numerous complimentary analytical techniques can be employed to examine changes in the physicochemical features of biochar obtained as the desired product of slow pyrolysis and is illustrated in Figure 8. Figure 8 highlights the complimentary analytical techniques that can be utilized to investigate the change in physicochemical characteristics of biochar and the transition occurred in the starting material through the thermal degradation pathway. The physicochemical properties and structural features in terms of proximate and ultimate analysis, pH analysis, HHV, specific surface area and SEM of biochar produced from both lignocellulosic biochar are discussed in the following section. The utilization of the analytical techniques also depends on the final application of the biochar (Amin et al., 2016). However, there are limitations with all the techniques currently available, and none can explain the transitions of biochar adequately. The investigation of the change in physicochemical properties of biochar is essential in terms of diverse applications.
4.1 Proximate Analysis, Ultimate Analysis and pH Analysis
The proximate and ultimate analyses of various lignocellulosic biomass is shown in Table 4. It is essential to investigate the proximate and ultimate composition before undergoing slow pyrolysis because high carbon (C) and low ash content is highly desirable for such high temperature conversion processes. The high C content is the characteristic of biochar produced from lignocellulosic biomass. In slow pyrolysis, increasing both pyrolysis reaction time and temperature and decreasing the heating rate increases the concentration of carbon (% C) in the biochar and decreases both the oxygen (% O) and hydrogen (%H) owing to the loss of moisture and other volatile components during the process. The loss in O and H reduces the surface hydrophilicity in biochar which is essential for transportation and long-term storage perspective. During the thermal treatment, the chemical environment of carbon in biochar is altered to produce recalcitrant aromatic structure that are highly resistant to biological (microbial) degradation. Mukherjee et al. (2021a) showed that with increasing the pyrolysis temperature to 600°C, the carbon content (C%) increases and oxygen content (O%) decreases, as result, the extent of aromatization enhanced but polarity reduces. The stability of lignocellulosic biochar is relatively high owing to the developed of aromatic structure and recalcitrant C. Residual biomass containing higher ash content influences the biochar yield and is positively correlated with the generation. The plot of H/C and O/C atomic ratios are now widely used to characterize the fuel properties of biochar and is defined as the Van-Krevelen diagram, and to estimate the enhancement in fuel properties compared to coal. The atomic ratio reduces at elevated temperature that also indicates the improvement in aromatic characteristics, hydrophobicity, or polarity in biochar. Examining pH in aqueous solution of biochar is essential especially when its end application is for soil treatment. The pH of biochar derived from lignocellulose-biomass ranges in the alkaline range typically ranging from 8–9.6 (Yu et al., 2019). The pH of biochar derived from lignocellulose-biomass ranges in the alkaline range. According to a study by Liu et al. (2014), the enhancement of pH in biochar is associated to the reduction of organic oxygenated acidic functional moieties at elevated thermal conditions, such as carboxylic functional (–COOH) and hydroxyl functional (–OH) groups from the biochar matrix mainly during the pyrolysis.
4.2 Higher Heating Value
When lignocellulose biomass undergoes complete combustion, it releases heat and the amount of heat released during this period is defined as the higher heating value (HHV) of the tested sample. The sample upon complete combustion produces water (H2O) and carbon dioxide (CO2). The latent heat of water and water vapor is given off upon condensation and HHV includes latent heat as the heating value. The HHV is influenced by the carbon content and ash content in the sample under test. The calorific values of the sample are positively correlated with the carbon content, whereas negatively correlated with the ash content. In general, lignocellulosic biochar has higher HHV, owing to a lower ash content in biomass feedstock. In this regard, Patra et al. (2021a) reported that the HHV of food waste was 17.0 MJ kg−1 and that improved for the biochar with the highest value of 23 MJ kg−1 obtained at the optimized pyrolysis conditions (600°C, 5°C min−1 and 60 min). Enhancement in HHV is beneficial from energy perspective as it will allow to substitute coal and be explored as the renewable source of energy and clean fuel.
4.3 Textural Characteristics and Surface Morphology
In general, the surface area of lignocellulosic biomass is low with undeveloped porosity and very low pore-volume. However, with treating at elevated temperature during slow pyrolysis, secondary reactions like thermal cracking, devolatilization, aromatization, decarboxylation, dehydration and polymerization reactions results in biochar’s surface disintegration, fragmentation, and development of porous network and structure. The surface area of the biochar is also strongly dependent mainly on the feedstock type and pyrolysis temperature. Liu et al. (2014) studied the change in textural properties of corncob derived biochar at different pyrolysis temperatures and further compared its characteristics with sawdust and corn stalk. They reported the improvement in the specific surface area (SBET) of corncob-derived biochar with increase in temperature from 300 to 500°C increased from 61.8 to 212.6 m2/g. However, the specific surface area reduced slightly to 192.9 m2/g at 600°C.
A plausible explanation to this observation can be attributed to secondary polymerization reaction, that can destroy the micropores due to excessive pore-widening in the resulting biochar. Although, the specific surface area of corncob synthesized at 500°C (CC500) was lower than that of sawdust synthesized at the same pyrolysis temperature (SD500), and it can also be attributed to the high ash content in corncob resisting the formation of microporous structure. Hence, the development of textural properties is also influenced by the feedstock type and composition. Figures 9A–C illustrates the SEM image of the biomass (food waste) and the derived biochar generated at the extreme conditions of temperature (300 and 600 C), respectively (Patra et al., 2021a). It is clear from Figures 9A,B that food waste biomass as well as the biochar derived at 300 C got undeveloped porosity as a result lower surface area (0.85 m2/g, 300°C-30 min) owing to not high enough thermal condition that could contribute towards the development of porous structure. However, with rising pyrolysis temperature to 600°C, secondary reactions like thermal cracking, devolatilization, aromatization, decarboxylation, dehydration results in biochar’s surface disintegration, fragmentation, and development of porous structure as can be clearly seen from Figure 9C.
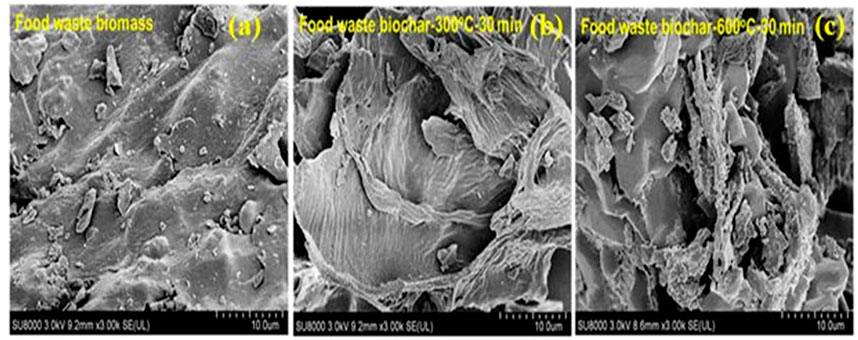
FIGURE 9. (A-C). SEM image of food waste biochar derived from different pyrolysis temperature (used with permission from Patra et al., 2021a).
5 Biochar for Diverse Industrial Applications
Due to its unique physical and chemical characteristics, biochar is often used in soil amendment (Zhang et al., 2019), carbon sequestration (Taskin et al., 2019), removal of pollutant from waste water (Nanda et al., 2016), carbon dioxide adsorption (Mukherjee et al., 2021b), energy storage (Bader et al., 2018) and supercapacitor (Lu and Zhao, 2017) as summarized in the following section. One of the most popular applications of biochar is in the field of soil amendment by improving its productivity and fertility followed by its ability to sequester carbon by enhancing soil organic carbon (SOC), purification technologies, energy storage and supercapacitor and finally as the precursor to be converted to activated carbon. Considering the excellent properties like large specific surface area, well-developed porous structure, availability of rich and variant surface functional groups, and mineral content also make biochar a potential alternative source material for environment cleanup and a potential candidate for energy harvesting. For instance, biochar’s performance and efficacy in removing organic or inorganic contaminants from wastewater or removing CO2 from waste flue gas stream emitted from the large point stationary sources are influenced by the enhanced porous structure and well-developed micro-porosity, improved hydrophobicity, which are dependent on the feedstock compositions, degradation pathway and can be controlled by the pyrolysis conditions. The various applications of biochar in different industrial sectors like for soil remediation, carbon dioxide sequestration, adsorbent, precursor to synthesize activated carbon, energy storage and supercapacitor is summarized in the following section through extensive literature review.
5.1 Biochar for Soil Remediation
The advantageous effects of biochar utilization for development of physical properties of soil have been comprehensively evaluated, for instance, the incorporation of biochar into soil can induce the net surface area of the soil and improve soil bulk-density and porosity (Devereux et al., 2012). Moreover, biochar addition to soil enhances the soil-water correlation by increasing water infiltration and water holding capacity (Qian et al., 2015). The alterations on the physical properties of soil are mainly ascribed to biochar’s low bulk density and large surface area. In addition, biochar added soil can modify its pH value as shown in Figure 10. The low pH in soil can result in plant toxicity due to the presence of heavy metals. Thus, the application of biochar is beneficial, especially for acid contaminated soils due to the release of alkaline substances contracting soil acidification. The properties and characteristics of the soil are substantially modified over the mid- and long-terms, which indicates a stout CO2 sink (Palansooriya et al., 2019). Presence of carboxyl groups in pyrolyzed biochar increases cation exchange capacity of soil, which efficiently limits cationic nutrient leaching and enhances water retention capacity. Cationic nutrient helps in enhancing seed germination and plant growth resulting in high crop yield (Ferreira et al., 2019). Contamination of soil with heavy metals affects soil productivity and have adverse health effects on crop consumers. Biochar has been known for its phenomenal sorption capacity in removing heavy metals and other contaminants from the soil. The effective adsorption of heavy metals requires high surface area, which biochar lacks. However, biochar surface comprises several oxygenated functional groups like phenolic, carboxyl, and hydroxyl, which acts as binding sites for soil pollutants (Qian et al., 2015).
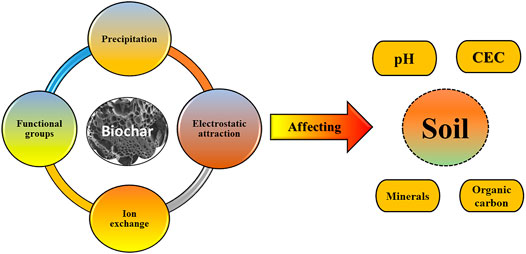
FIGURE 10. Features of biochar influencing the soil properties (adapted and redrawn from Wang et al., 2020).
5.2 Biochar for Soil Nutrition and Fertility
Incorporated biochar in soil can significantly aid as a source or sink for accessible nutrients, as biochar itself acts as source of nutrients derived from lignocellulosic biomass (Palansooriya et al., 2019). The physical, chemical, and microbial properties of the soil also get improved using biochar and its integration into soil substantiates to be an efficient technique for augmenting nutrient cycling, affecting overall plant performance (El-Naggar et al., 2019). Biochar can also implicitly alter the soil’s nutrient content and its obtainability for the crops and when surplus nutrients are overloaded on biochar, it slowly releases vital fertilizer for delivering nutrients to the crop (Zhou et al., 2015). Biochar can also absorb feedstock derived nutrients (N, P, K, Ca, Mg, S, Fe, and Mn) along with both macro and micro-nutrients.
The reason behind such aspect of the biochar is solely due the presence of large surface area and porous microstructure in biochar, thus nutrients associated with biochar, releases in a slow manner (Clough et al., 2013). Biochar has potential to alter soil biological properties, which involves amelioration of soil, enhancement of nutrient cycles, alteration of mineral composition. Such alteration improves the microbial community composition in soil and results in enhancement of soil organic matter, which promotes plant growth and high crop productivity. Moreover, biochar enhances soil fertility by limiting the emissions of N2O and NO (Ding et al., 2016).
5.3 Biochar for Carbon Sequestration
The process of up adsorbing and storing atmospheric CO2 is defined as the carbon sequestration. If added to soil, biochar can be a potential carbon sequestration tool and could contribute to enhance the soil-carbon sink. Adding biochar to the soil could eventually have a positive impact on the environment, reducing the net CO2 emission in the atmosphere. In this regard, for instance, since ages the charred organic matter in the carbon-rich Terra Preta soil located in the Amazon basin is sequestering carbon (Xiao et al., 2016). Thus, biochar has been widely accepted as a promising carbon sequestration tool by implementing it in soil as depicted in Figure 11. Figure 11 highlights the carbon-cycle and how implementing biochar in soil can help achieve a negative carbon-loop. The performance of biochar as soil-carbon sink can be attributed to resistance to biological and chemical degradation, as well as porous structure. The enhanced recalcitrant carbon content and developed aromatic structure contribute towards the high level of resistance towards biological or chemical degradation for a prolonged period. Through slow pyrolysis at elevated temperature conditions, the recalcitrance and stability of the carbon increases in biochar as also mentioned in section 4.1. Additionally, with progress of reaction time and temperature, the condensed aromatic content enhances along with the loss of aliphatic constituents in biochar and hence contribute towards enhanced chemical stability. Hence, applying biochar in soil potentially sequesters soil carbon for a prolonged period in the range of hundreds or thousands of years. Masto et al. (2013) reported that converting Eichornia biomass to biochar was a sustainable strategy and beneficial from the perspective of enhanced soil fertility. They also observed changes in the soil carbon sequestration as assessed by the improvement in soil respiration by 1.9 times after applying biochar in soil. Additionally, adding biochar to soil can also reduce emission of toxic gas such methane (CH4) from cultivated soils (Gómez et al., 2016).
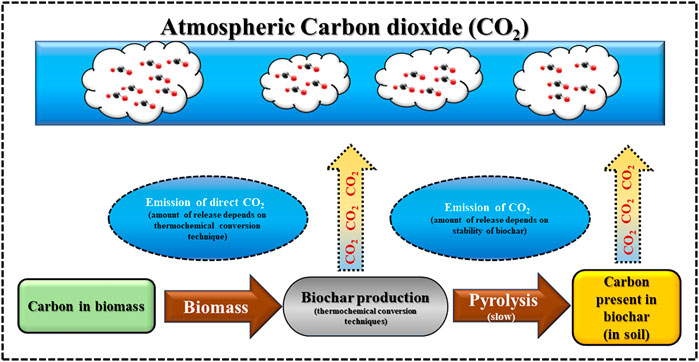
FIGURE 11. Impact of biochar production on the carbon cycle (adapted from Meyer et al., 2011).
5.4 Biochar as Adsorbent for Wastewater Treatment
The wide availability of biochar, its low cost, and environmental sustainability along with relatively high adsorption capacity make them a promising alternative for the removal of a broad range of pollutants namely heavy metals, pesticides, synthetic dyes, and antibiotics. The source of wastewater is generally associated with domestic and industrial activities, which includes organic and inorganic pollutants, pathogenic microorganisms, plastics, heavy metals, oil, hydrocarbons, dyes, pharmaceutical wastes, pesticides, toxic pollutants in wastewater. All such contaminants have immediate antagonistic effect on the metabolism of the living creatures (flora and fauna) and natural habitat (Sun et al., 2019; Elgarahy et al., 2021). The contaminated water from different sources when discharged to the freshwater bodies causes deterioration of the water. Domestic and industrial wastewater undergo different types of treatment processes like physical, biological, chemical before discharging to the environment. However, in some cases, these processes are found to be ineffective and costlier. The techniques often used for treatment of wastewater are found to be incompetent and lack in the efficient removal of toxic compounds from water. Therefore, advance, and specific treatments are required for wastewater before discharging it into natural water resources. Biochar with high organic carbon content has immense potential in eliminating contaminants from wastewater, and recent advancements in biochar application in wastewater treatment validates its proficient applicability in removing contaminants (Xiang et al., 2020). Different kinds of interaction like electrostatic attraction, H-bonding and co-precipitation plays significant role in removing contaminants from the wastewater.
Industrial wastewater contains heavy metals, which, even at very low concentrations, has adverse effects on human health causing cancer, pulmonary disorders, and cardiovascular (Patra et al., 2021b). Discharge of heavy metals into water bodies also threatens aquatic life. Biochar is considered as good absorbents, which can remove containments such as heavy metals like Al, Pb, Hg, Ni, U, As, Cd, Cr, Cu and Zn. Biochar is considered as cost-effective treatment material with well-developed textural properties (high specific surface area, porous structure) and developed surface functional moieties (mainly oxygenated), making it effective adsorbent for the removal of heavy metals from waste water. The adsorption capability of biochar depends upon the biomass feedstock as well as on the conversion technology and the processing conditions used for the synthesis of biochar. It also depends on the conversion technology and its process condition for the synthesis of biochar. Mainly pyrolysis and gasification derived biochar have been utilized as an adsorbent for water decontamination (Mohan et al., 2014; Cha et al., 2016; Patra et al., 2021b). Zhao J. et al. (2019) studied performances of different biochar derived from poplar, corn, Brassica napus, and sewage sludge via pyrolysis for the adsorption of mixture of heavy metals like Pb (II), Cr(III), Cu(II), and Cd(II). The research highlighted complexation, porous structure, and cation exchange (most significant) as the foremost mechanisms for the adsorption of multiple heavy metals from the aqueous solution. In another study, Zamani et al. (2017) reported the removal of zinc using biochar derived from oil palm empty fruit bunches. Authors achieved zinc adsorption capacity of biochar as 15.2 mg/g with a yield of 25.5 wt%. Elwakeel et al. (2015) studied the removal of Fe (II) and Mn (II) from water by using sugarcane bagasse derived activated carbon. The biochar was chemically activated at 500 C after impregnation with H3PO4 and showed surface area of 671.54 m2/g. At room temperature 25 C the activated carbon showed high adsorption capacity of 7.01 and 5.40 mg/g, respectively, for Fe (II) and Mn (II). The author reported that the acidity of the medium supported the better efficiency of the adsorbent in adsorbing the contaminants.
The wastewater from dye industries poses threats to the ecosystem as the effluent includes acids, bases, and other impurities with a coloring agent. Typical textile dyes often withstand aerobic digestion because of its stability to oxidizing agents, making its removal challenging (Patra et al., 2021b). Many studies have investigated the elimination of dyes using biochar derived from bamboo, rice husk, palm bark, weed, eucalyptus, sludge, and cow dung. Adsorption of malachite green using rice husk derived biochar was reported by Leng et al. (2015). The study reported that the liquefaction biochar obtained from rice husk using ethanol solvent was found to be effective in malachite green adsorption with a capacity between 32.5 and 67.6 mg−1. In another study, the sugarcane bagasse derived biochar prepared at pyrolysis temperature of 800 C was found to be effective for malachite green adsorption over biochar derived from paper waste, cotton waste, rice husk, and ground nutshell biomass (Vyavahare et al., 2018). Sun et al. (2013) used biochar derived from various lignocellulosic precursors for the adsorption of methylene blue dye and have reported the efficacy of biochar. Biomass such as eucalyptus, municipal solid waste, and palm bark were carbonized at 400 C and the resulted biochar were investigated for the adsorption performance. The efficiency of biochar derived from municipal solid waste, palm bark, and eucalyptus in removing methylene blue were found at 99.5, 99.3 and 86.1% respectively, at 40°C, concentration 5 mg/L and pH 7.
The disposal of pharmaceutical sludge is difficult and associated with several hazards. In order to reduce such waste, Wu et al. (2022) adopted an approach to produce biochar from pharmaceutical sludge via pyrolysis for adsorption of fluoroquinolone antibiotics from wastewater. The biochar produced at optimum conditions of temperature 800 C and reaction time of 90 min and further modified with ZnCl2 showed surface area of 543 m2/g. The biochar showed promising result for adsorption of the contaminant with high adsorption capacity of 159.26 mg/g. Biochar shows high sorption efficiency for pharmaceutical wastes and Essandoh et al. (2015) showed effective removal of pharmaceutical compounds such as salicylic acid and ibuprofen from aqueous solution using biochar derived from pine wood. The adsorption capacity of biochar for salicylic acid and ibuprofen was found to be 22.7 and 10.7 mg/g, respectively.
Petroleum wastes also possess a significant threat for the environment and need proper treatment before disposal. Singh et al. (2020) used agro-residue like rice straw, energy crop like bagasse, woody biomass like eucalyptus wood derived biochar for the remediation of naphthenic acids rich petroleum wastewater. The biochar was pyrolyzed at 500 C for 2 h and then treated with acids like HCl and HNO3. The rice straw biochar treated with HCl showed surface area of 49.01 m2/g and showed better efficacy for adsorption of benzoic acid with adsorptive capacity of 7.97 mg/g in column mode and 8.66 mg/g in batch mode. In another study Singh et al. (2021) reported adsorption of phenol from petroleum wastewater using eucalyptus biomass derived biochar. The biochar activated with KOH showed high surface area of 2048 m2/g with phenol adsorption capacity of 308.9 mg/g.
5.5 Biochar for Carbon Dioxide Adsorption
Biochar has been acknowledged for its unique physicochemical properties and have shown superior performance compared to other carbonaceous materials like activated carbon in many instances like sequestering carbon dioxide (CO2) from flue gas stream. This could be attributed to the presence of variant basic surface functional moieties as well as developed textural properties of biochar. A similar finding on an enhanced performance of biochar compared to commercial activated carbon was reported by Mukherjee et al. (2021a). They reported that biochar prepared from spent coffee grounds (SCG) by slow pyrolysis exhibited enhanced textural and physicochemical properties which were used to investigate the CO2 capture performance in a typical post-combustion scenario (30°C and 30 volume% of CO2 balanced by N2). They reported that biochar prepared at 600°C (SCG-600) showed comparatively better performance (2.8 mmol g−1) than commercial activated carbon (AC). Owing to the well-developed textural properties, aromatic structure and availability of basic functional moieties enhanced the CO2 capture performance under a typical post-combustion scenario. A similar finding was reported by Igalavithana et al. (2020) where they have compared the CO2 adsorption performance at 25°C of biochar derived from pine sawdust and paper mill sludge. They observed that compare to paper mill sludge derived biochar, pine saw dust derived biochar performed better (0.67 mmol g−1) owing to the higher surface area and well-developed microporous structure. In addition, Xu et al. (2019) studied the influence of N-doped (ammonium hydroxide) biochar derived from bagasse (BG) and hickory chips (HC) by ball milling for carbon dioxide capture. They observed and reported that the biochar derived from BG and HC synthesized at 450 C were doped with more N containing species (amine and nitrile) than the biochar that synthesized at 600 C. Additionally, they reported that to the increment of N containing groups the CO2 capture performance was enhanced owing to the improved acid-base interactions. For instance, the CO2 adsorption performance of N-doped and ball milled hickory chips biochar synthesized at 450 C (BMHC450-N) showed an improvement of 31.6–55.2% higher than the pristine and ball milled biochar. The enhanced performance of biochar compared to highly developed carbonaceous materials like activated carbon can be attributed to the retainment of essential surface functionalities mainly N-containing species in the adsorbent which are essential to create a bond between the adsorbate (CO2) which is Lewis acid in nature with slight toxicity (Shewchuk et al., 2021). Considering the wide availability of cheap lignocellulose biomass for biochar production, the use of biochar could be a cheaper remediation alternative for adsorption of variant contaminants like CO2 from flue gas stream emitted from large point stationary sources than synthesizing activated carbon. The reason could be attributed to the involvement of lot of cost owing to higher thermal treatment conditions, involvement of sophisticated and costly equipment and chemicals for surface modification. However, thermal treatment like activating the biochar at higher temperature conditions in presence of suitable activating agents or post-treatment of biochar with appropriate functionalizing agents are essential in an aim to produce carbon material with improved textural properties in terms of higher specific surface area, distribution of micropore volume and surface chemistry that could enhance CO2 adsorption capacity and selectivity under a typical post-combustion scenario.
5.6 Biochar-Based Activated Carbon for Gold Recovery
Biochar can also be explored as the starting material to synthesize activated carbon via two-step physical activation technique (Mukherjee et al., 2019). Activated carbon is synthesized via two modes, physical and chemical activation. Generally, in physical activation, activated carbon is synthesized via two-step process where biomass-based biochar is produced first via thermochemical conversion process like slow pyrolysis. Further the biochar is activated in presence of variant oxidizing agents and the most popular as well as commercially used physical activating agents include steam, CO2, or air. The activated carbon produced via two steps physical activation technique is an environmentally friendly strategy compared to one step chemical activation. Also, in chemical activation technique, the biomass feedstock is thermally treated in one step where it undergoes simultaneous pyrolysis and activation process.
Biochar based activated carbon is used for numerous industrial applications including gold recovery. Various agricultural residues based activated carbon samples are used for the efficient recovery of gold from the ore owing to their large specific surface area, strong micro-porous structure, high mechanical strength and loading capacity (Poinern et al., 2011; Khosravi et al., 2017). The granular shape activated carbon derived from high quality coal, coconut shell by high temperature steam activation process is used as an adsorbent in the gold processing industry to recover gold owing to unique advantages, such as large specific area, large pore volume, strong adsorption capacity, high mechanical hardness, good chemical stability, easy regeneration, and durability. To recover gold, first of all, the ore is finely ground and the gold is soluble in cyanide compound such as calcium cyanide, sodium cyanide, or potassium cyanide and water are added to ore to form slurry. The gold particle leaches out and mixes with the cyanide into a solution. The activated carbon is added to the leached slurry to adsorb the gold from the slurry solution and then removed from the slurry by coarse screening.
The activated carbons with uniform particle size (5–10, 8–30, 30–60 mesh), strong adsorption capacity, strong adsorption rate, and high wear resistance are most appropriate for gold extraction. Gold (Au) is a precious metal that exists in its natural state. Cyanide is a chemical compound of carbon-nitrogen (C≡N) that reacts with gold. Activated carbon is basically uncharged, and its huge inner surface area is neutral, so it can adsorb only neutral substances. The mechanism of physical adsorption onto the activated carbon is achieved by the formation of chemical bond with two opposite charges. An ion-pair is created due to electrostatic attraction of calcium positive ion and the gold cyanide negative ion by an ionic bond. The physical adsorption causes from the van der Waals interactions between calcium-gold cyanide ion-pairs and molecule of activated carbon. The porous structure combined with the attractive force enables activated carbon to capture material components and hold them for recycling. The formation of gold cyanide ion is described in the following Eqs. 2–4 (Rogans, 2012):
At first the gold is leached with sodium cyanide, water and oxygen. The mechanism of the extraction of gold is not easy; however, the formation of chemical reaction and the dissolution of gold from the ore in water and the gold cyanide ion approached as follows:
Gold cyanide ions in water prefer neutrals, so the negative charge is offset by any positive ions like Na+, K+, Ca2+, Mg2+, H+, etc. In case of calcium, gold cyanide ions combine with calcium ion to make a calcium gold cyanide ion-pair. The ability of adsorption of gold depends on the type of cation. H+ > Ca2+ > Mg2+ > K+ > Na+
A neutral new chemical species is formed in combination of calcium positive ion and gold cyanide negative ion together called ‘ion-pair’. Hence to form a neutral species, the ions of the two opposite charges balance each other without changing. In case of calcium, gold cyanide ions combine with calcium ion and makes calcium gold cyanide ion-pair.
Hence, chemical reaction does not take place in between the surface molecules of the carbon material and gold cyanide ion-pair. The adsorption of ‘Au’ ions on the surface of activated carbon can take place as follows:
Since the activated carbon is inert and does not carry any charge, so it absorbs only the neutral species. Hence the base metal cyanide adsorbed as neutral species. The stability of the formation of ion pair’s strongly dependent on the type of the anion charge. A greater probability of adsorption of gold performed by the inert activated carbon owing to the stable ion pair that is positively influenced by the lower anion charge.
Some influencing factors such as particle size of carbon, types of activated carbon, temperature, pH, cyanide concentration, mixing efficiency, pulp density, organic poisons, inorganic poisons, ionic strength, gold tenor, contact time, etc. are to be considered for both kinetic and equilibrium adsorption of gold.
5.7 Biochar for Energy Storage Application
In new advancements, biochar has been applied as modern source materials for energy storage, like hydrogen storage, oxygen electrocatalysts, and leading fuel cell technologies. The most used electrode materials are activated carbon, carbon nanotubes, and graphene, etc (Tan et al., 2018). Though some of these materials have high specific capacitance, but the production cost is relatively expensive. The profusion of activated biochar with its potential characteristics of physical and chemical properties (e.g., plentiful oxygen containing functional groups, huge surface area), and its simple processing methods make biochar an economical, ecofriendly materials for energy storage.
5.7.1 Biochar-Based Activated Carbon for Hydrogen Storage
Hydrogen is the lightest element. It is a non-toxic energy carrier and has a high energy value of 142 MJ/kg (Møller, et al., 2017). Hydrogen is considered as an ideal replacement for fossil fuels. Hydrogen has the highest energy density of any other fuel. The adsorption ability of hydrogen in biomass-derived activated carbon depends on its pore geometry, pore size distributions, surface porosity, micro-porous volume, surface functionality, heteroatoms’ content as well the storage pressure and temperature. The recent studies show that the synthesized activated carbon under the ultrasonic wave technique has been found the highest uptake of H2 because of having high surface area. The capacity of hydrogen storage increased in the activated carbon derived from without and with ultrasonic were 0.38, 0.43 g/g, respectively at 77 K and 80 bar (Dogan, et al., 2018). The uptake of hydrogen under the low pressure, generally dominated by pores in the scale of sub-nanometer size of porous materials. Hydrogen storage capacity can be increased either by increasing pressure or lowering the temperature. The interaction between the hydrogen molecules is weak Van der Waals force. The Van der Waals interaction between the adsorbent and hydrogen to be increased if temperature is lowered at 77 K or at higher pressures (Atkinson, et al., 2001; Darkrim, et al., 2002). For the standard activated carbon, high adsorption capacity (0.04 ∼ 0.06 kg/kg) of hydrogen has been found at very low cryogenic temperatures. The constant increase in the adsorption isotherms can be obtained in the near-mesoporous region of the activated carbon samples at high pressure.
The properties reducing the pore size, the interaction between the hydrogen and carbon adsorbents can be enhanced (Czakkel, et al., 2019). As the size of the pore decreases, then the absolute value of the heat of adsorption increases, which ultimately increases the interaction between hydrogen and the carbon molecule. For the smaller pores, the van der Waals potentials favor stronger physisorption due to the atoms of the adjacent walls (Bader, et al., 2018). Adsorption of hydrogen and methane are typically taking place in micropores range. A pore size in the range of 0.7–1.5 nm favors maximum uptake of hydrogen. The adsorption performance of H2 mainly depends on the pore dimensions, pore surface area, pore distributions, structure, bulk density, and adsorption conditions (temperature and pressure) (Takagi et al., 2004; Panella et al., 2005).
The activated carbon as prepared (Wang et al., 2009) by the KOH activation agent has very high surface area and narrow pores, and high hydrogen adsorption was observed at 7.08 wt% at 77 K and 2 MPa (Wang et al., 2009). About 10 wt% improvement in hydrogen storage capacity was observed after nitrogen doping with metal (Pt)- supported activated carbon composites at room temperature, provided the Pt composition in the composites remained the same (Zhao W. et al., 2019). The hydrogen storage capacity of activated carbon at room temperature remains challenging. Though a substantial amount of work has been done to exhibit the potential use of activated carbon for hydrogen storage capacity, but still some important studies, like role of surface morphology, surface functionality, physical conditions, thermodynamic properties, etc., are to be investigated for its profound uses.
5.8 Biochar as an Electrode Material for Supercapacitor
Since 1990, researchers and scientists have made significant progress in improving energy storage performance, specifically in respect of energy density and power capability. Most available electrochemical capacitors are electric double-layer capacitors. In this device, activated carbons are used in both the electrodes and propylene carbonate or acetonitrile as electrolyte solvent. Necessarily, factors such as, pore structure, pore surface area, structural defects, heteroatoms attachment and electrode design, etc., should be considered, thus, to determine the optimal electrochemical performance of porous activated carbons for supercapacitors. Although a great attention has been made by many researchers to produce the potential biochar for new uses, but still further research is needed to overcome the remaining challenges of using these products from the applications point of view.
Due to increasing demand and uses of numerous battery-operated electronic devices, more electrical energy is needed. It is thus cost effective high-efficient storage of energy is needed from renewable sources. Supercapacitor technology is one of the most promising and proficient technologies of energy storage devices for the next generation. Supercapacitor’s are getting popular owing to their capability of fast charging and discharging rates, high power density, high power capacity and long durable life cycle (Zhang and Zhao, 2009; Wang G. et al., 2012).
In general, biomass is structurally porous, bountiful, more sustainable, and less expensive in comparison to non-renewable fossil-fuel based carbon sources (Zhang et al., 2015). Biomass sources tend to be rich in heteroatoms like nitrogen (N), sulphur (S), phosphorus (P) and oxygen (O). The application of surface heteroatoms into the carbon framework accelerates the electrical transport properties, wettability, and the capacitive properties of biomass derived carbon materials (Jin et al., 2018). Heteroatoms cause to change the properties of the surface heterogeneity of the carbon materials and regulate their chemical properties. Due to the benefit of heteroatoms, carbonaceous precursor materials doped with heteroatoms can be transformed into activated carbon materials by activation processes. The role of N-heteroatoms has been studied to increase the electrochemical properties of the carbon materials. The presence of N atoms in carbonaceous materials could provide more effective acelectroactive sites because of having electron-donor properties. Due to the change of valence electron in the energy levels of closest interconnected carbon atoms, defects are created thus to increase the electrical conduction mechanism, the surface polarity of molecules and wettability behaviors (Luo et al., 2021).
Thus, to produce low-cost, environmentally friendly supercapacitors, electrode materials can be made from various biomasses derived carbon electrodes. In view of low cost, plentiful sources, outstanding physicochemical properties and rich carbon content and low inorganic matrix lignocellulose-based materials may have great potential to achieve higher efficiency of supercapacitor electrodes. Biochar derived activated carbon has the characteristics of renewable, sustainable, economic, outstanding chemical and thermal stability, and large surface area green material resource energy for the production of efficient electrode materials for supercapacitor (Inagaki et al., 2010; Lu and Zhao, 2017).
As an efficient conductor in the supercapacitor, carbon electrodes have been considered due to carrying of plenty of free electrons in the carbon for transfer of charges in electrolysis process. The carbon materials have high melting point, so it can facilitate a wide range of different reactions. The carbon-based nanostructured materials are basically hierarchical porous structure with well-adjusted distribution of interconnected micropores, mesopores and macropores. The macropores (over 50 nm) serve as the ion-buffering pools, mesopores (2–50 nm) act as the transport channels of electrolyte ions and micropores help as the storage sites of charges (Simon and Gogotsi, 2008). In addition, some other salient features of the carbon materials are pore dimension, pore shape, pore distributions, electrical conductivity and surface functionality, etc. These parameters have a great influence on the electrochemical performance of a capacitor. Because of low cost, abundant sources, eco-friendliness, excellent physicochemical stability, lignocellulose-based activated carbon may have great potential to increase the performance of supercapacitor electrodes due to their high carbon content and low inorganic matrix. A well-developed structure with the combination of micropores, mesopores and macropores derived from the different precursor source materials have been investigated as carbon electrode for supercapacitor (Frackowiak, 2007; Li et al., 2007).
Most available electrochemical capacitors use activated carbon as the electrodes and electrolyte solvent (acetonitrile/propylene carbonate) to manufacture double-layered electrochemical capacitors. The porous activated carbon as an electrode used in supercapacitors influence the electrochemical performance because of large surface area, well-built porous structure, surface functionalities in terms of available heteroatoms, structural defects, and electrode structure.
Nowadays, almost all manufacturers of supercapacitors use coconut shell for producing activated carbon as the active electrode material (Yang et al., 2010). Also a number of biomass precursors have been carbonized and transformed into activated carbons by chemical treatment for supercapacitors such as the cherry stone (Olivares-Marín et al., 2009), fish scale (Chen et al., 2010), waste paper (Kalpana et al., 2009), water bamboo (Li and Wu, 2015), yeast cells (Sun et al., 2013), pine-cone (Karthikeyan et al., 2014), willow catkins (Wang S. et al., 2015), celtuce leaves (Wang R. et al., 2012), waste tea leaves (Peng et al., 2013), sunflower seed shell (Li et al., 2011), ginkgo shells (Jiang et al., 2013), cow dung (Bhattacharjya and Yu, 2014), human hair (Qian et al., 2014) and sewage sludge (Feng et al., 2015). These materials have potential applications in electrochemical performance because of their large surface area, pore size, pore distribution (Cartula et al., 1991). As a result, they have a bigger area for storing more charge.
6 Future Research Directions and Challenges
Biochar can be tagged as a renewable source of carbon-based material. However, further investigations are essential to fill the gaps of the production and valorization of lignocellulose-based biomass in terms of selecting the appropriate lignocellulosic biomass as the precursor or optimizing the production conditions for diverse industrial applications of engineered biochar.
• Extensive investigation is necessary to establish a correlation between the feedstock nature, biochar yield and its structural features and its properties. To reveal the change in biochar structure, in depth qualitative and quantitative investigations using appropriate analytical tools are essential to characterize biochar.
• Production of biochar with optimized physicochemical features for suitable industrial applications is needed. The biochar properties are highly influenced by the pyrolytic operating parameters mainly pyrolysis temperatures (°C), residence time (s or min) and heating rate (°C s−1 or °C min−1). The influence of the pyrolytic parameters is necessary to be revealed individually along with their interactions should also be considered using statistical tools and numerical techniques like Taguchi’s method or Box-Behnken design (BBD) of response surface methodology (RSM) to properly design the pyrolysis reactor and optimize the desired product distribution.
• Post-treatment of biochar to increase its demand in larger scale. Different post-treatment methods, such as activation, can also enhance the biochar physicochemical properties mainly in terms of textural properties via formation of more porous network. Also, the selective functionalization of different functional groups or heteroatoms into biochar matrix could improve its surface functionalities via further surface modification. Besides, synthesis of renewable biochar composites with high specific surface area, well developed porosity and varying surface functionalities can be proposed as a promising strategy.
• To produce biochar with desirable properties, the synthesis and post-treatment of biochar should be synchronised. However, it is also imperative to consider the usage of green functional groups or dopants to treat the biochar surface to reduce the environmental impact. The regeneration of biochar is also needed to be considered from commercial perspective. Various incentives and schemes are necessary to attract market value of the pyrolysis products and to make it a viable renewable technology.
7 Conclusion
Slow pyrolysis is a potential thermochemical conversion technology that could be explored in converting lignocellulosic biomass into useful products mainly biochar. The yield of biochar and their physiochemical characteristics vary substantially and are dependent on numerous factors such as, biomass type, composition and characteristics, pyrolysis operating conditions namely pyrolysis temperature, heating rate and residence time. To obtain maximum amount of biochar, then maintaining the pyrolysis temperature at a moderate range, moderate to prolonged residence time and slower heating rate is preferable. With increasing the pyrolysis temperature, the content of stable carbon in the biochar matrix enhances along with the aromatic content which provides resistance to degradation for a considerable period. Beneficial impacts of biochar include in diverse industrial sectors namely, such as in soil amendment, carbon dioxide sequestration, adsorbent for carbon dioxide capture and wastewater treatment, energy storage and utilization as supercapacitors owing to its superior textural characteristics and appropriate surface functionalities. Several research are on track for the development of slow-pyrolysis technology to maximize their efficiency and economic benefits in terms of biochar yield and biochar properties. However, extensive investigations are essential to elucidate the relationship between the biochar production technology and biochar performance in various industrial applications.
Author Contributions
AM and BP and JP contributed to the writing and design of the study. Added their expertise in shaping the manuscript and contributed to developing high impactful graphs and figures. AD, played a vital role in planning, corrections and reviews, which helped in enhancing the quality of the manuscript. All authors contributed to manuscript revision, read, and approved the submitted version.
Conflict of Interest
The authors declare that the research was conducted in the absence of any commercial or financial relationships that could be construed as a potential conflict of interest.
Publisher’s Note
All claims expressed in this article are solely those of the authors and do not necessarily represent those of their affiliated organizations, or those of the publisher, the editors and the reviewers. Any product that may be evaluated in this article, or claim that may be made by its manufacturer, is not guaranteed or endorsed by the publisher.
Supplementary Material
The Supplementary Material for this article can be found online at: https://www.frontiersin.org/articles/10.3389/fmats.2022.870184/full#supplementary-material
References
Al-Wabel, M. I., Al-Omran, A., El-Naggar, A. H., Nadeem, M., and Usman, A. R. A. (2013). Pyrolysis Temperature Induced Changes in Characteristics and Chemical Composition of Biochar Produced from Conocarpus Wastes. Bioresour. Technol. 131, 374–379. doi:10.1016/j.biortech.2012.12.165
Amin, F. R. (2016). Biochar Applications and Modern Techniques for Characterization. Clean Technol. Environ. Policy 18 (5), 1457–1473. doi:10.1007/s10098-016-1218-8
Anca-Couce, A. (2016). Reaction Mechanisms and Multi-Scale Modelling of Lignocellulosic Biomass Pyrolysis. Prog. Energy Combust. 53, 41–79. doi:10.1016/j.pecs.2015.10.002
Anupam, K., Sharma, A. K., Lal, P. S., Dutta, S., and Maity, S. (2016). Preparation, Characterization and Optimization for Upgrading Leucaena Leucocephala Bark to Biochar Fuel with High Energy Yielding. Energy 106, 743–756. doi:10.1016/j.energy.2016.03.100
Atkinson, K., Roth, S., Hirscher, M., and Grünwald, W. (2001). Carbon Nanostructures: An Efficient Hydrogen Storage Medium for Fuel Cells. Fuel Cells Bull. 4 (38), 9–12. doi:10.1016/S1464-2859(01)80733-1
Aysu, T., and Küçük, M. M. (2014). Biomass Pyrolysis in a Fixed-Bed Reactor: Effects of Pyrolysis Parameters on Product Yields and Characterization of Products. Energy 64, 1002–1025. doi:10.1016/j.energy.2013.11.053
Azargohar, R., Jacobson, K. L., Powell, E. E., and Dalai, A. K. (2013). Evaluation of Properties of Fast Pyrolysis Products Obtained, from Canadian Waste Biomass. J. Anal. Appl. Pyrolysis. 104, 330–340. doi:10.1016/j.jaap.2013.06.016
Bader, N., Zacharia, R., Abdelmottaleb, O., and Cossement, D. (2018). How the Activation Process Modifies the Hydrogen Storage Behavior of Biomass-Derived Activated Carbons. J. Porous Mat. 25 (1), 221–234. doi:10.1007/s10934-017-0436-8
Bhattacharjya, D., and Yu, J. S. (2014). Activated Carbon Made from Cow Dung as Electrode Material for Electrochemical Double Layer Capacitor. J. Power Sources 262 (15), 224–231. doi:10.1016/j.jpowsour.2014.03.143
Biswas, B., Pandey, N., Bisht, Y., Singh, R., Kumar, J., and Bhaskar, T. (2017). Pyrolysis of Agricultural Biomass Residues: Comparative Study of Corn Cob, Wheat Straw, Rice Straw and Rice Husk. Bioresour. Technol. 237, 57–63. doi:10.1016/j.biortech.2017.02.046
Bruun, E. W., Ambus, P., Egsgaard, H., and Hauggaard-Nielsen, H. (2012). Effects of Slow and Fast Pyrolysis Biochar on Soil C and N Turnover Dynamics. Soil Biol. biochem. 46, 73–79. doi:10.1016/j.soilbio.2011.11.019
Cai, J., He, Y., Yu, X., Banks, S. W., Yang, Y., Zhang, X., et al. (2017). Review of Physicochemical Properties and Analytical Characterization of Lignocellulosic Biomass. Renew. Sust. Energy Rev. 76, 309–322. doi:10.1016/j.rser.2017.03.072
Cartula, F., Molina-Sabio, M., and Rodriguez-Reinoso, F. (1991). Preparation of Activated Carbon by Chemical Activation with ZnCl2. Carbon 29, 999–1007. doi:10.1016/0008-6223(91)90179-M
Cha, J. S., Park, S. H., Jung, S. C., Ryu, C., Jeon, J. K., Shin, M. C., et al. (2016). Production and Utilization of Biochar: A Review. J. Ind. Eng. Chem. 40, 1–15. doi:10.1016/j.jiec.2016.06.002
Chen, D., Liu, D., Zhang, H., Chen, Y., and Li, Q. (2015). Bamboo Pyrolysis Using TG–FTIR and a Lab-Scale Reactor: Analysis of Pyrolysis Behavior, Product Properties, and Carbon and Energy Yields. Fuel 148, 79–86. doi:10.1016/j.fuel.2015.01.092
Chen, W., Zhang, H., Huang, Y., and Wang, W. (2010). A Fish Scale Based Hierarchical Lamellar Porous Carbon Material Obtained Using a Natural Template for High Performance Electrochemical Capacitors. J. Mat. Chem. 20, 4773–4775. doi:10.1039/c0jm00382d
Chiodo, V., Zafarana, G., Maisano, S., Freni, S., and Urbani, F. (2016). Pyrolysis of Different Biomass: Direct Comparison Among Posidonia Oceanica, Lacustrine Alga and White-Pine. Fuel 164, 220–227. doi:10.1016/j.fuel.2015.09.093
Clough, T. J., Condron, L. M., Kammann, C., and Müller, C. (2013). A Review of Biochar and Soil Nitrogen Dynamics. Agronomy 3 (2), 275–293.
Czakkel, O., Nagy, B., Dobos, G., Fouquet, P., Bahn, E., and László, K. (2019). Static and Dynamic Studies of Hydrogen Adsorption on Nanoporous Carbon Gels. Int. J. Hydrog. Energy. 44 (33), 18169–18178. doi:10.1016/j.ijhydene.2019.05.131
Daful, A. G., and Chandraratne, M. R. (2020). Biochar Production from Biomass Waste-Derived Material. Encycl. Renew. Sust. Mat. 4, 370–378. doi:10.1016/b978-0-12-803581-8.11249-4
Darkrim, F. L., Malbrunot, P., and Tartaglia, G. P. (2002). Review of Hydrogen Storage by Adsroption in Carbon Nanotubes. Int. J. Hydrogen Energy 27 (2), 193–202. doi:10.1016/S0360-3199(01)00103-3
de Caprariis, B., De Filippis, P., Petrullo, A., and Scarsella, M. (2017). Hydrothermal Liquefaction of Biomass: Influence of Temperature and Biomass Composition on the Bio-Oil Production. Fuel 208, 618–625. doi:10.1016/j.fuel.2017.07.054
Devereux, R. C., Sturrock, C. J., and Mooney, S. J. (2012). The Effects of Biochar on Soil Physical Properties and Winter Wheat Growth. Earth Environ. Sci. Trans. R. Soc. Edinb. 103 (1), 13–18. doi:10.1016/j.geoderma.2018.09.03410.1017/s1755691012000011
Ding, Y., Liu, Y., Liu, S., Li, Z., Tan, X., Huang, X., et al. (2016). Biochar to Improve Soil Fertility. A Rev. Agron. sustain. Dev. 36 (2), 1–18. doi:10.1007/s13593-016-0372-z10.1016/j.ultsonch.2014.07.005
Dogan, E. E., Tokcan, P., and Kizilduman, B. K. (2018). Storage of Hydrogen in Activated Carbons and Carbon Nanotubes. Adv. Mater. Sci. 18 (4), 5–16. doi:10.1515/adms-2017-0045
El-Naggar, A., Lee, S. S., Rinklebe, J., Farooq, M., Song, H., Sarmah, A. K., et al. (2019). Biochar Application to Low Fertility Soils: A Review of Current Status, and Future Prospects. Geoderma 337, 536–554. doi:10.1016/j.geoderma.2018.09.034
Elgarahy, A. M., Elwakeel, K. Z., Mohammad, S. H., and Elshoubaky, G. A. (2021). A Critical Review of Biosorption of Dyes, Heavy Metals and Metalloids from Wastewater as an Efficient and Green Process. Clean. Eng. Technol. 4, 100209. doi:10.1016/j.clet.2021.100209
Elmay, Y., Le Brech, Y., Delmotte, L., Dufour, A., Brosse, N., and Gadiou, R. (2015). Characterization of Miscanthus Pyrolysis by DRIFTs, UV Raman Spectroscopy and Mass Spectrometry. J. Anal. Appl. Pyrolysis. 113, 402–411. doi:10.1016/j.jaap.2015.03.004
Elwakeel, K. Z., El-Sayed, G. O., and Abo El-Nassr, S. M. (2015). Removal of Ferrous and Manganous from Water by Activated Carbon Obtained from Sugarcane Bagasse. Desalin. Water Treat. 55 (2), 471–483. doi:10.1080/19443994.2014.919606
Essandoh, M., Kunwar, B., Pittman, C. U., Mohan, D., and Mlsna, T. (2015). Sorptive Removal of Salicylic Acid and Ibuprofen from Aqueous Solutions Using Pine Wood Fast Pyrolysis Biochar. Chem. Eng. J. 265, 219–227. doi:10.1016/j.cej.2014.12.006
Farrokh, N. T., Suopajärvi, H., Mattila, O., Umeki, K., Phounglamcheik, A., Romar, H., et al. (2018). Slow Pyrolysis of By-Product Lignin from Wood-Based Ethanol Production– A Detailed Analysis of the Produced Chars. Energy 164, 112–123. doi:10.1016/j.energy.2018.08.161
Feng, H., Zheng, M., and Dong, H. (2015). Three-Dimensional Honeycomb-Like Hierarchically Structured Carbon for High-Performance Supercapacitors Derived from High-Ash-Content Sewage Sludge. J. Mat. Chem. A 3, 15225–15234. doi:10.1039/C5TA03217B
Ferjani, A. I., Jeguirim, M., Jellali, S., Limousy, L., Courson, C., Akrout, H., et al. (2019). The Use of Exhausted Grape Marc to Produce Biofuels and Biofertilizers: Effect of Pyrolysis Temperatures on Biochars Properties. Renew. Sust. Energy Rev. 107, 425–433. doi:10.1016/j.rser.2019.03.034
Ferreira, S. D., Manera, C., Silvestre, W. P., Pauletti, G. F., Altafini, C. R., and Godinho, M. (2019). Use of Biochar Produced from Elephant Grass by Pyrolysis in a Screw Reactor as a Soil Amendment. Waste Biomass Valor 10 (10), 3089–3100. doi:10.1007/s12649-018-0347-1
Frackowiak, E. (2007). Carbon Materials for Supercapacitor Application. Phys. Chem. Chem. Phys. 9, 1774. doi:10.1039/B618139M
Gómez, N., Rosas, J. G., Cara, J., Martínez, O., Alburquerque, J. A., and Sánchez, M. E. (2016). Slow Pyrolysis of Relevant Biomasses in the Mediterranean Basin. Part 1. Effect of Temperature on Process Performance on a Pilot Scale. J. Clean. Prod. 120, 181–190. doi:10.1016/j.jclepro.2014.10.082
González, M. E., Romero-Hermoso, L., González, A., Hidalgo, P., Meier, S., Navia, R., et al. (2017). Effects of Pyrolysis Conditions on Physicochemical Properties of Oat Hull Derived Biochar. Bioresources 12 (1), 2040–2057. doi:10.15376/biores.12.1.2040-2057
Gordon, D. R., Tancig, K. J., Onderdonk, D. A., and Gantz, C. A. (2011). Assessing the Invasive Potential of Biofuel Species Proposed for Florida and the United States Using the Australian Weed Risk Assessment. Biomass Bioenerg. 35 (1), 74–79. doi:10.1016/j.biombioe.2010.08.029
Halder, P., Kundu, S., Patel, S., Parthasarathy, R., Pramanik, B., Paz-Ferreiro, J., et al. (2019). TGA-FTIR Study on the Slow Pyrolysis of Lignin and Cellulose-Rich Fractions Derived from Imidazolium-Based Ionic Liquid Pre-Treatment of Sugarcane Straw. Energy Convers. Manag. 200, 112067. doi:10.1016/j.enconman.2019.112067
Huang, Y. F., Chiueh, P. T., and Lo, S. L. (2016). A Review on Microwave Pyrolysis of Lignocellulosic Biomass. Sustain. Environ. Res. 26 (3), 103–109. doi:10.1016/j.serj.2016.04.012
Igalavithana, A. D., Choi, S. W., Shang, J., Hanif, A., Dissanayake, P. D., Tsang, D. C. W., et al. (2020). Carbon Dioxide Capture in Biochar Produced from Pine Sawdust and Paper Mill Sludge: Effect of Porous Structure and Surface Chemistry. Sci. Total Environ. 739, 139845. doi:10.1016/j.scitotenv.2020.139845
Inagaki, M., Konno, H., and Tanaike, O. (2010). Carbon Materials for Electrochemical Capacitors. J. Power Sources 195 (24), 7880–7903. doi:10.1016/j.jpowsour.2010.06.036
Jafri, N., Wong, W. Y., Doshi, V., Yoon, L. W., and Cheah, K. H. (2018). A Review on Production and Characterization of Biochars for Application in Direct Carbon Fuel Cells. Process Saf. Environ. Prot. 118, 152–166. doi:10.1016/j.psep.2018.06.036
Jiang, L., Yan, J., and Hao, L. (2013). High Rate Performance Activated Carbons Prepared from Ginkgo Shells for Electrochemical Supercapacitors. Carbon 56, 146–154. doi:10.1016/j.carbon.2012.12.085
Jin, H., Li, J., Yuan, Y., Wang, J., Lu, J., and Wang, S. (2018). Recent Progress in Biomass‐Derived Electrode Materials for High Volumetric Performance Supercapacitors. Adv. Energy Mat. 8 (23), 1801007. doi:10.1002/aenm.201801007
Kalpana, D., Cho, S. H., Lee, S. B., Lee, Y. S., Misrac, R., and Renganathan, N. G. (2009). Recycled Waste Paper–A New Source of Raw Material for Electric Double-Layer Capacitors. J. Power Sources 190 (2), 587–591. doi:10.1016/j.jpowsour.2009.01.058
Kambo, H. S., and Dutta, A. (2015). A Comparative Review of Biochar and Hydrochar in Terms of Production, Physico-Chemical Properties and Applications. Renew. Sust. Energ Rev. 45, 359–378. doi:10.1016/j.rser.2015.01.050
Karthikeyan, K., Amaresh, S., Lee, S. N., Sun, X., Aravindan, V., Lee, G. Y., et al. (2014). Construction of High-Energy-Density Supercapacitors from Pine-Cone-Derived High-Surface-Area Carbons. Chem. Sus. Chem. 7 (5), 1435–1442. doi:10.1002/cssc.201301262
Kazawadi, D., Ntalikwa, J., and Kombe, G. (2021). A Review of Intermediate Pyrolysis as a Technology of Biomass Conversion for Coproduction of Biooil and Adsorption Biochar. J. Renew. Energy. 2021, 1–10. doi:10.1155/2021/5533780
Khosravi, R., Azizi, A., Ghaedrahmati, R., Gupta, V. K., and Agarwal, S. (2017). Adsorption of Gold from Cyanide Leaching Solution onto Activated Carbon Originating from Coconut Shell—Optimization, Kinetics and Equilibrium Studies. J. Ind. Eng. Chem. 54, 464–471. doi:10.1016/j.jiec.2017.06.036
Kim, D., Lee, K., and Park, K. Y. (2016). Upgrading the Characteristics of Biochar from Cellulose, Lignin, and Xylan for Solid Biofuel Production from Biomass by Hydrothermal Carbonization. J. Ind. Eng. Chem. 42, 95–100. doi:10.1016/j.jiec.2016.07.037
Kim, H., Ahn, Y., and Kwak, S. Y. (2016). Comparing the Influence of Acetate and Chloride Anions on the Structure of Ionic Liquid Pretreated Lignocellulosic Biomass. Biomass Bioenerg. 93, 243–253. doi:10.1016/j.biombioe.2016.07.022
Lee, X. J., Lee, L. Y., Gan, S., Thangalazhy-Gopakumar, S., and Ng, H. K. (2017). Biochar Potential Evaluation of Palm Oil Wastes Through Slow Pyrolysis: Thermochemical Characterization and Pyrolytic Kinetic Studies. Bioresour. Technol. 236, 155–163. doi:10.1016/j.biortech.2017.03.105
Leng, L., Yuan, X., Zeng, G., Shao, J., Chen, X., Wu, Z., et al. (2015). Surface Characterization of Rice Husk Bio-Char Produced by Liquefaction and Application for Cationic Dye (Malachite Green) Adsorption. Fuel 155, 77–85. doi:10.1016/j.fuel.2015.04.019
Li, J., and Wu, Q. (2015). Water Bamboo-Derived Porous Carbons as Electrode Materials for Supercapacitors. New J. Chem. 39, 3859–3864. doi:10.1039/C4NJ01853B
Li, W., Chen, D., Li, Z., Shi, Y., Wan, Y., Wang, G., et al. (2007). Nitrogen-Containing Carbon Spheres with Very Large Uniform Mesopores: The Superior Electrode Materials for EDLC in Organic Electrolyte. Carbon 45 (9), 1757–1763. doi:10.1016/j.carbon.2007.05.004
Li, X., Strezov, V., and Kan, T. (2014). Energy Recovery Potential Analysis of Spent Coffee Grounds Pyrolysis Products. J. Anal. Appl. Pyrolysis. 110, 79–87. doi:10.1016/j.jaap.2014.08.012
Li, X., Xing, W., Zhuo, S., Zhou, J., Li, F., Qiao, Z. S., et al. (2011). Preparation of Capacitor’s Electrode from Sunflower Seed Shell. Bioresour. Technol. 102 (2), 1118–1123. doi:10.1016/j.biortech.2010.08.110
Liu, X., Zhang, Y., Li, Z., Feng, R., and Zhang, Y. (2014). Characterization of Corncob-Derived Biochar and Pyrolysis Kinetics in Comparison with Corn Stalk and Sawdust. Bioresour. Technol. 170, 76–82. doi:10.1016/j.biortech.2014.07.077
Lu, H., and Zhao, X. S. (2017). Biomass-Derived Carbon Electrode Materials for Supercapacitors. Sustain. Energy Fuels 1 (6), 1265–1281. doi:10.1039/C7SE00099E
Luo, X.-Y., Chen, Y., and Mo, Y. (2021). A Review of Charge Storage in Porous Carbon-Based Supercapacitors. New Carbon Mater. 36 (1), 49–68. doi:10.1016/s1872-5805(21)60004-5
Ma, Z., Chen, D., Gu, J., Bao, B., and Zhang, Q. (2015). Determination of Pyrolysis Characteristics and Kinetics of Palm Kernel Shell Using TGA–FTIR and Model-Free Integral Methods. Energy Convers. Manag. 89, 251–259. doi:10.1016/j.enconman.2014.09.074
Mandal, A., Singh, N., and Purakayastha, T. J. (2017). Characterization of Pesticide Sorption Behaviour of Slow Pyrolysis Biochars as Low Cost Adsorbent for Atrazine and Imidacloprid Removal. Sci. Total Environ. 577, 376–385. doi:10.1016/j.scitotenv.2016.10.204
Manić, N. G., Janković, B. Ž., Stojiljković, D. D., Jovanović, V. V., and Radojević, M. B. (2019). TGA-DSC-MS Analysis of Pyrolysis Process of Various Agricultural Residues. Therm. Sci. 23, 1457–1472. doi:10.2298/TSCI180118182M
Masto, R. E., Kumar, S., Rout, T. K., Sarkar, P., George, J. C., and Ram, L. C. (2013). Biochar from Water Hyacinth (Eichornia Crassipes) and its Impact on Soil Biological Activity. Catena 111, 64–71. doi:10.1016/j.catena.2013.06.025
Meyer, S., Glaser, B., and Quicker, P. (2011). Technical, Economical, and Climate-Related Aspects of Biochar Production Technologies: A Literature Review. Environ. Sci. Technol. 45 (22), 9473–9483. doi:10.1021/es201792c
Mohan, D., Sarswat, A., Ok, Y. S., and Pittman, C. U. (2014). Organic and Inorganic Contaminants Removal from Water with Biochar, a Renewable, Low Cost and Sustainable Adsorbent–A Critical Review. Bioresour. Technol. 160, 191–202. doi:10.1016/j.biortech.2014.01.120
Mohanty, P., Nanda, S., Pant, K. K., Naik, S., Kozinski, J. A., and Dalai, A. K. (2013). Evaluation of the Physiochemical Development of Biochars Obtained from Pyrolysis of Wheat Straw, Timothy Grass and Pinewood: Effects of Heating Rate. J. Anal. Appl. Pyrolysis. 104, 485–493. doi:10.1016/j.jaap.2013.05.022
Møller, K. T., Jensen, T. R., Akiba, E., and Li, H. W. (2017). Hydrogen-A Sustainable Energy Carrier. Prog. Nat. Sci. 27 (1), 34–40. doi:10.1016/j.pnsc.2016.12.014
Mukherjee, A., Borugadda, V. B., Dynes, J. J., Niu, C., and Dalai, A. K. (2021a). Carbon Dioxide Capture from Flue Gas in Biochar Produced from Spent Coffee Grounds: Effect of Surface Chemistry and Porous Structure. J. Environ. Chem. Eng. 9, 106049. doi:10.1016/j.jece.2021.106049
Mukherjee, A., Okolie, J. A., Abdelrasoul, A., Niu, C., and Dalai, A. K. (2019). Review of Post-Combustion Carbon Dioxide Capture Technologies Using Activated Carbon. J. Environ. Sci. (China) 83, 46–63. doi:10.1016/j.jes.2019.03.014
Mukherjee, A., Okolie, J. A., Tyagi, R., Dalai, A. K., and Niu, C. (2021b). Pyrolysis Kinetics and Activation Thermodynamic Parameters of Exhausted Coffee Residue and Coffee Husk Using Thermogravimetric Analysis. Can. J. Chem. Eng. 99, 1683–1695. doi:10.1002/cjce.24037
Nanda, S., Dalai, A. K., Berruti, F., and Kozinski, J. A. (2016). Biochar as an Exceptional Bioresource for Energy, Agronomy, Carbon Sequestration, Activated Carbon and Specialty Materials. Waste Biomass Valorization 7 (2), 201–235. doi:10.1007/s12649-015-9459-z
Okolie, J. A., Nanda, S., Dalai, A. K., and Kozinski, J. A. (2020). Hydrothermal Gasification of Soybean Straw and Flax Straw for Hydrogen-Rich Syngas Production: Experimental and Thermodynamic Modeling. Energy Convers. Manag. 208, 112545. doi:10.1016/j.enconman.2020.112545
Olivares-Marín, M., Fernández, J. A., Lázaro, M. J., Fernández-González, C., Macías-García, A., Gómez-Serrano, V., et al. (2009). Cherry Stones as Precursor of Activated Carbons for Supercapacitors. Mat. Chem. Phys. 114, 323–327. doi:10.1016/j.matchemphys.2008.09.010
Palansooriya, K. N., Wong, J. T. F., Hashimoto, Y., Huang, L., Rinklebe, J., Chang, S. X., et al. (2019). Response of Microbial Communities to Biochar-Amended Soils: A Critical Review. Biochar. 1 (1), 3–22. doi:10.1007/s42773-019-00009-2
Panella, B., Hirscher, M., and Roth, S. (2005). Hydrogen Adsorption in Different Carbon Nanostructures. Carbon 43 (10), 2209–2214. doi:10.1016/j.carbon.2005.03.037
Patra, B. R., Nanda, S., Dalai, A. K., and Meda, V. (2021a). Slow Pyrolysis of Agro-Food Wastes and Physicochemical Characterization of Biofuel Products. Chemosphere 285, 131431. doi:10.1016/j.chemosphere.2021.131431
Patra, B. R., Nanda, S., Dalai, A. K., and Meda, V. (2021b). Taguchi-Based Process Optimization for Activation of Agro-Food Waste Biochar and Performance Test for Dye Adsorption. Chemosphere 285, 131531. doi:10.1016/j.chemosphere.2021.131531
Patra, B. R., Mukherjee, A., Nanda, S., and Dalai, A. K. (2021c). Biochar Production, Activation and Adsorptive Applications: A Review. Environ. Chem. Lett. 19 (3), 2237–2259. doi:10.1007/s10311-020-01165-9
Patra, D., Patra, B. R., Pattnaik, F., Hans, N., and Kushwaha, A. (2022). “Recent Evolution in Green Technologies for Effective Valorization of Food and Agricultural Wastes,” in Emerging Trends to Approaching Zero Waste (Elsevier), 103–132. doi:10.1016/B978-0-323-85403-0.00001-3
Pattanaik, L., Pattnaik, F., Saxena, D. K., and Naik, S. N. (2019). “Biofuels from Agricultural Wastes,” in Second and Third Generation of Feedstocks (Elsevier), 103–142. doi:10.1016/B978-0-12-815162-4.00005-7
Pattnaik, F., Tripathi, S., Patra, B. R., Nanda, S., Kumar, V., Dalai, A. K., et al. (2021). Catalytic Conversion of Lignocellulosic Polysaccharides to Commodity Biochemicals: a Review. Environ. Chem. Lett. 19 (6), 4119–4136. doi:10.1007/s10311-021-01284-x
Peng, C., Yan, X. B., Wang, R. T., Lang, W. J., Ou, Y., and Xue, J. Q. (2013). Promising Activated Carbons Derived from Waste Tea-Leaves and Their Application in High Performance Supercapacitors Electrodes. Electrochim. Acta. 87, 401–408. doi:10.1016/j.electacta.2012.09.082
Poinern, G. E. J., Senanayake, G., Shah, N., Thi-Le, X. N., Parkinson, G. M., and Fawcett, D. (2011). Adsorption of the Aurocyanide, Au (CN) 2-Complex on Granular Activated Carbons Derived from Macadamia Nut Shells–A Preliminary Study. Min. Eng. 24 (15), 1694–1702. doi:10.1016/j.mineng.2011.09.011
Primaz, C. T., Schena, T., Lazzari, E., Caramao, E. B., and Jacques, R. A. (2018). Influence of the Temperature in the Yield and Composition of the Bio-Oil from the Pyrolysis of Spent Coffee Grounds: Characterization by Comprehensive Two Dimensional Gas Chromatography. Fuel 232, 572–580. doi:10.1016/j.fuel.2018.05.097
Qambrani, N. A., Rahman, M. M., Won, S., Shim, S., and Ra, C. (2017). Biochar Properties and Eco-Friendly Applications for Climate Change Mitigation, Waste Management, and Wastewater Treatment: A Review. Renew. Sust. Energy Rev. 79, 255–273. doi:10.1016/j.rser.2017.05.057
Qian, K., Kumar, A., Zhang, H., Bellmer, D., and Huhnke, R. (2015). Recent Advances in Utilization of Biochar. Renew. Sust. Energy Rev. 42, 1055–1064. doi:10.1016/j.rser.2014.10.074
Qian, W., Sun, F., and Xu, Y. (2014). Human Hair-Derived Carbon Flakes for Electrochemical Supercapacitors. Energy Environ. Sci. 7, 379–386. doi:10.1039/C3EE43111H
Rangabhashiyam, S., and Balasubramanian, P. (2019). The Potential of Lignocellulosic Biomass Precursors for Biochar Production: Performance, Mechanism and Wastewater Application-A Review. Ind. Crops Prod. 128, 405–423. doi:10.1016/j.indcrop.2018.11.041
Russell, S. H., Turrion-Gomez, J. L., Meredith, W., Langston, P., and Snape, C. E. (2017). Increased Charcoal Yield and Production of Lighter Oils from the Slow Pyrolysis of Biomass. J. Anal. Appl. Pyrolysis 124, 536–541. doi:10.1016/j.jaap.2017.01.028
Saldarriaga, J. F., Aguado, R., Pablos, A., Amutio, M., Olazar, M., and Bilbao, J. (2015). Fast Characterization of Biomass Fuels by Thermogravimetric Analysis (TGA). Fuel 140, 744–751. doi:10.1016/j.fuel.2014.10.024
Setter, C., Silva, F. T. M., Assis, M. R., Ataíde, C. H., Trugilho, P. F., and Oliveira, T. J. P. (2020). Slow Pyrolysis of Coffee Husk Briquettes: Characterization of the Solid and Liquid Fractions. Fuel 261, 116420. doi:10.1016/j.fuel.2019.116420
Shalini, R., Pugalendhi, S., Subramanian, P., and Gopal, N. O. (2017). Characteristic Study on Biochar Production from Biological Substrates by Slow Pyrolysis for Carbon Sequestration. Int. J. Curr. Microbiol. Appl. Sci. 6 (4), 314–323. doi:10.20546/ijcmas.2017.604.034
Shariff, A., Mohamad Aziz, N. S., Ismail, N. I., and Abdullah, N. (2016). Corn Cob as a Potential Feedstock for Slow Pyrolysis of Biomass. J. Phys. Sci. 27 (2), 123–137. doi:10.21315/jps2016.27.2.9
Shewchuk, S. R., Mukherjee, A., and Dalai, A. K. (2021). Selective Carbon-Based Adsorbents for Carbon Dioxide Capture from Mixed Gas Streams and Catalytic Hydrogenation of CO2 into Renewable Energy Source: A Review. Chem. Eng. Sci. 243, 116735. doi:10.1016/j.ces.2021.116735
Simon, P., and Gogotsi, Y. (2008). Materials for Electrochemical Capacitors. Nat. Mater 7, 845–854. doi:10.1038/nmat2297
Singh, R., Dutta, R. K., Naik, D. V., Ray, A., and Kanaujia, P. K. (2021). High Surface Area Eucalyptus Wood Biochar for the Removal of Phenol from Petroleum Refinery Wastewater. Environ. Chal. 5, 100353. doi:10.1016/j.envc.2021.100353
Singh, R., Naik, D. V., Dutta, R. K., and Kanaujia, P. K. (2020). Biochars for the Removal of Naphthenic Acids from Water: A Prospective Approach Towards Remediation of Petroleum Refinery Wastewater. J. Clean. Prod. 266, 121986. doi:10.1016/j.jclepro.2020.121986
Solar, J., De Marco, I., Caballero, B. M., Lopez-Urionabarrenechea, A., Rodriguez, N., Agirre, I., et al. (2016). Influence of Temperature and Residence Time in the Pyrolysis of Woody Biomass Waste in a Continuous Screw Reactor. Biomass and Bioener. 95, 416–423.
Subhedar, P. B., Babu, N. R., and Gogate, P. R. (2015). Intensification of Enzymatic Hydrolysis of Waste Newspaper Using Ultrasound for Fermentable Sugar Production. Ultrason. Sonochem. 22, 326–332. doi:10.1016/j.ultsonch.2014.07.005
Subhedar, P. B., Ray, P., and Gogate, P. R. (2018). Intensification of Delignification and Subsequent Hydrolysis for the Fermentable Sugar Production from Lignocellulosic Biomass Using Ultrasonic Irradiation. Ultrason. Sonochem. 40, 140–150. doi:10.1016/j.ultsonch.2017.01.030
Sun, H., He, W., Zong, C., and Lu, L. (2013). Template-Free Synthesis of Renewable Macroporous Carbon via Yeast Cells for High Performance Supercapacitor Electrode Materials. ACS Appl. Mat. Interfaces 5 (6), 2261–2268. doi:10.1021/am400206r
Sun, J., Dai, X., Wang, Q., van Loosdrecht, M. C., and Ni, B. J. (2019). Microplastics in Wastewater Treatment Plants: Detection, Occurrence and Removal. Water Res. 152, 21–37. doi:10.1016/j.watres.2018.12.050
Takagi, H., Hatori, H., Soneda, Y., Yoshizawa, N., and Yamada, Y. (2004). Adsorptive Hydrogen Storage in Carbon and Porous Materials. Mat. Sci. Eng. B 108 (1-2), 143–147. doi:10.1016/j.mseb.2003.10.095
Tan, L., Li, X., Wang, Z., Guo, H., Wang, J., and An, L. (2018). Multifunctional Separator With Porous Carbon/Multi-Walled Carbon Nanotubes Coating for Advanced Lithium-Sulphur Batteries. ChemElectrochem. 5 (1), 71–77. doi:10.1002/celc.201700986
Taskin, E., de Castro Bueno, C., Allegretta, I., Terzano, R., Rosa, A. H., and Loffredo, E. (2019). Multianalytical Characterization of Biochar and Hydrochar Produced from Waste Biomasses for Environmental and Agricultural Applications. Chemosphere 233, 422–430. doi:10.1016/j.chemosphere.2019.05.204
Tomczyk, A., Sokołowska, Z., and Boguta, P. (2020). Biochar Physicochemical Properties: Pyrolysis Temperature and Feedstock Kind Effects. Rev. Environ. Sci. Biotechnol. 19 (1), 191–215. doi:10.1007/s11157-020-09523-3
Uroić Štefanko, A., and Leszczynska, D. (2020). Impact of Biomass Source and Pyrolysis Parameters on Physicochemical Properties of Biochar Manufactured for Innovative Applications. Front. Energy Res. 8, 138. doi:10.3389/fenrg.2020.00138
Vieira, F. R., Luna, C. M. R., Arce, G. L., and Ávila, I. (2020). Optimization of Slow Pyrolysis Process Parameters Using a Fixed Bed Reactor for Biochar Yield from Rice Husk. Biomass Bioenerg. 132, 105412. doi:10.1016/j.biombioe.2019.105412
Vyavahare, G. D., Gurav, R. G., Jadhav, P. P., Patil, R. R., Aware, C. B., and Jadhav, J. P. (2018). Response Surface Methodology Optimization for Sorption of Malachite Green Dye on Sugarcane Bagasse Biochar and Evaluating the Residual Dye for Phyto and Cytogenotoxicity. Chemosphere 194, 306–315. doi:10.1016/j.chemosphere.2017.11.180
Wang, D., Jiang, P., Zhang, H., and Yuan, W. (2020). Biochar Production and Applications in Agro and Forestry Systems: A Review. Sci. Total Environ. 723, 137775. doi:10.1016/j.scitotenv.2020.137775
Wang, G., Zhang, L., and Zhang, J. (2012). A Review of Electrode Materials for Electrochemical Supercapacitors. Chem. Soc. Rev. 41 (2), 797–828. doi:10.1039/C1CS15060J
Wang, H., Gao, Q., and Hu, J. (2009). High Hydrogen Storage Capacity of Porous Carbons Prepared by Using Activated Carbon. J. Am. Chem. Soc. J. 131 (20), 7016–7022. doi:10.1021/ja8083225
Wang, H., Wang, X., Cui, Y., Xue, Z., and Ba, Y. (2018). Slow Pyrolysis Polygeneration of Bamboo (Phyllostachys Pubescens): Product Yield Prediction and Biochar Formation Mechanism. Bioresour. Technol. 263, 444–449. doi:10.1016/j.biortech.2018.05.040
Wang, K., Zhao, N., and Lei, S. (2015). Promising Biomass Based Activated Carbons Derived from Willow Catkins for High Performance Supercapacitors. Electrochim. Acta. 166, 1–11. doi:10.1016/j.electacta.2015.03.048
Wang, R., Wang, P., Yan, X., Lang, J., Peng, C., and Xue, Q. (2012). Promising Porous Carbon Derived from Celtuce Leaves with Outstanding Supercapacitance and CO2 Capture Performance. Appl. Mat. Interfaces. 4 (11), 5800–5806. doi:10.1021/am302077c
Wang, S., Gao, B., Zimmerman, A. R., Li, Y., Ma, L., Harris, W. G., et al. (2015). Physicochemical and Sorptive Properties of Biochars Derived from Woody and Herbaceous Biomass. Chemosphere 134, 257–262. doi:10.1016/j.chemosphere.2015.04.062
Wang, Y., Kang, K., Yao, Z., Sun, G., Qiu, L., Zhao, L., et al. (2018). Effects of Different Heating Patterns on the Decomposition Behavior of White Pine Wood During Slow Pyrolysis. Int. J. Agric. Biol. 11 (5), 218–223. doi:10.25165/j.ijabe.20181105.3156
Wilk, M., and Magdziarz, A. (2017). Hydrothermal Carbonization, Torrefaction and Slow Pyrolysis of Miscanthus Giganteus. Energy 140, 1292–1304. doi:10.1016/j.energy.2017.03.031
Wu, Q., Zhang, Y., Cui, M. H., Liu, H., Liu, H., Zheng, Z., et al. (2022). Pyrolyzing Pharmaceutical Sludge to Biochar as an Efficient Adsorbent for Deep Removal of Fluoroquinolone Antibiotics from Pharmaceutical Wastewater: Performance and Mechanism. J. Hazard. Mat. 426, 127798. doi:10.1016/j.jhazmat.2021.127798
Xiang, W., Zhang, X., Chen, J., Zou, W., He, F., Hu, X., et al. (2020). Biochar Technology in Wastewater Treatment: A Critical Review. Chemosphere 252, 126539. doi:10.1016/j.chemosphere.2020.126539
Xiao, X., Chen, Z., and Chen, B. (2016). H/C Atomic Ratio as a Smart Linkage Between Pyrolytic Temperatures, Aromatic Clusters and Sorption Properties of Biochars Derived from Diverse Precursory Materials. Sci. Rep. 6, 1–13. doi:10.1038/srep22644
Xu, X. Q., Wang, Y. G., Chen, Z. D., Chen, X. J., Zhang, H. Y., Bai, L., et al. (2015). Variations in Char Structure and Reactivity Due to the Pyrolysis and In-Situ Gasification Using Shengli Brown Coal. J. Anal. Appl. Pyrolysis 115, 233–241. doi:10.1016/j.jaap.2015.08.001
Xu, X., Zheng, Y., Gao, B., and Cao, X. (2019). N-Doped Biochar Synthesized by a Facile Ball-Milling Method for Enhanced Sorption of CO2 and Reactive Red. Chem. Eng. J. 368, 564–572. doi:10.1016/j.cej.2019.02.165
Yu, S., Park, J., Kim, M., Ryu, C., and Park, J. (2019). Characterization of Biochar and Byproducts From Slow Pyrolysis of Hinoki Cypress. Biores. Tech. Rep. 6, 217–222.
Yang, K., Peng, J., Srinivasakannan, C., Zhang, L., Xia, H., and Duan, X. (2010). Preparation of High Surface Area Activated Carbon from Coconut Shells Using Microwave Heating. Bioresour. Technol. 101 (15), 6163–6169. doi:10.1016/j.biortech.2010.03.001
Yargicoglu, E. N., Sadasivam, B. Y., Reddy, K. R., and Spokas, K. (2015). Physical and Chemical Characterization of Waste Wood Derived Biochars. Waste Manage 36, 256–268. doi:10.1016/j.wasman.2014.10.029
Zamani, S. A., Yunus, R., Samsuri, A. W., Salleh, M. A., and Asady, B. (2017). Removal of Zinc from Aqueous Solution by Optimized Oil Palm Empty Fruit Bunches Biochar as Low-Cost Adsorbent. Bioinorg. Chem. Appl. 2017, 9. doi:10.1155/2017/7914714
Zhang, L., Liu, Z., Cui, G., and Chen, L. (2015). Biomass-Derived Materials for Electrochemical Energy Storages. Prog. Polym. 43, 136–164. doi:10.1016/j.progpolymsci.2014.09.003
Zhang, L. L., and Zhao, X. S. (2009). Carbon-Based Materials as Supercapacitor Electrodes. Chem. Soc. Rev. 38 (9), 2520–2531. doi:10.1039/B813846J
Zhang, Z., Zhu, Z., Shen, B., and Liu, L. (2019). Insights into Biochar and Hydrochar Production and Applications: A Review. Energy 171, 581–598. doi:10.1016/j.energy.2019.01.035
Zhao, J., Shen, X. J., Domene, X., Alcañiz, J. M., Liao, X., and Palet, C. (2019). Comparison of Biochars Derived from Different Types of Feedstock and Their Potential for Heavy Metal Removal in Multiple-Metal Solutions. Sci. Rep. 9, 9869. doi:10.1038/s41598-019-46234-4
Zhao, L., Cao, X., Mašek, O., and Zimmerman, A. (2013). Heterogeneity of Biochar Properties as a Function of Feedstock Sources and Production Temperatures. J. Hazard. Mat. 256, 1–9. doi:10.1016/j.jhazmat.2013.04.015
Zhao, W., Luo, L., Chen, T., Li, Z., Zhang, Z., Wang, H., et al. (2019). Synthesis and Characterization of Pt-N-Doped Activated Biocarbon Composites for Hydrogen Storage. Compos. B. Eng. 161, 464–472. doi:10.1016/j.compositesb.2018.12.122
Zhao, Y., Feng, D., Zhang, Y., Huang, Y., and Sun, S. (2016). Effect of Pyrolysis Temperature on Char Structure and Chemical Speciation of Alkali and Alkaline Earth Metallic Species in Biochar. Fuel Process. Technol. 141, 54–60. doi:10.1016/j.fuproc.2015.06.029
Keywords: lignocellulose, biomass, slow pyrolysis, biochar, soil nutrition, energy storage
Citation: Mukherjee A, Patra BR, Podder J and Dalai AK (2022) Synthesis of Biochar From Lignocellulosic Biomass for Diverse Industrial Applications and Energy Harvesting: Effects of Pyrolysis Conditions on the Physicochemical Properties of Biochar. Front. Mater. 9:870184. doi: 10.3389/fmats.2022.870184
Received: 06 February 2022; Accepted: 12 May 2022;
Published: 03 June 2022.
Edited by:
Mauro Giorcelli, Politecnico di Torino, ItalyReviewed by:
Khalid Z. Elwakeel, Jeddah University, Saudi ArabiaRaul Ocampo-Pérez, Autonomous University of San Luis Potosí, Mexico
Copyright © 2022 Mukherjee, Patra, Podder and Dalai. This is an open-access article distributed under the terms of the Creative Commons Attribution License (CC BY). The use, distribution or reproduction in other forums is permitted, provided the original author(s) and the copyright owner(s) are credited and that the original publication in this journal is cited, in accordance with accepted academic practice. No use, distribution or reproduction is permitted which does not comply with these terms.
*Correspondence: Ajay K. Dalai, YWpheS5kYWxhaUB1c2Fzay5jYQ==