- 1Jiangsu Key Laboratory of Materials and Technology for Energy Conversion, College of Materials Science and Technology, Nanjing University of Aeronautics and Astronautics, Nanjing, China
- 2Beijing Institute of Nanoenergy and Nanosystems, Chinese Academy of Sciences, Beijing, China
The contact electrification of materials plays an important role in developing and applying triboelectric nanogenerators (TENGs). By exploring the contact electrification phenomena at different interfaces, we can improve the understanding of the electrification mechanism and expand the application field of TENGs. In this way, the rate of energy utilization can be improved while the harm caused by the electrostatic effect is reduced. This article systematically summarized the different interface contacts between the research status quo of electricity. This article expounds the solid–solid interface, liquid–solid interface, and liquid–liquid interface, as well as the gas and other interface contact electrification mechanism, and the research and application of these are introduced; finally, it prospects the contact between the different interfaces of electric potential applications as well as the challenge.
Introduction
With the development of science and technology, energy has become an indispensable material basis and dynamic support for the development and progress of human society and has become the lifeblood of national development. Fossil fuels such as coal, oil, and natural gas have become the mainstream energy consumption in today’s society due to their early development and wide application. Excessive dependence on traditional fossil energy has brought about problems such as energy crisis and environmental pollution. As the world enters the era of the Internet of Things (IoT), sensor networks, big data, robotics, and artificial intelligence, more than 30 billion objects will be connected via the Internet of Things in the future (Atzori et al., 2010). The energy supply for these has presented a significant challenge. The conventional electromagnetic induction generator is a mechanical device that converts other forms of energy into mechanical energy, and then converts the mechanical energy into electrical energy by using the generator. It can convert wind energy, water energy, and other mechanical energy into electrical energy and carry it out, and has a wide range of applications in people’s daily life. It has the advantages of solidity, less auxiliary equipment, easy operation, and maintenance, but its complex power generation process leads to high cost and low system reliability, large volume and weight, harsh working environment requirements, and other disadvantages. As a result, it is particularly important to develop a new method to harvest the clean energy. In 2012, Wang et al, developed the triboelectric nanogenerators (TENGs) (Fan et al., 2012). The TENGs are a novel method of generating electricity by utilizing mechanical energy. The TENGs are a new method of using mechanical energy to generate electricity. It has the ability to convert low-frequency, high-entropy, and irregular energy in the environment, such as wind energy, ocean energy, and human movement energy, into electric energy for utilization (Tian et al., 2020). These distributed energy sources are not only a kind of new energy, but also the energy for us to move toward the Internet of Things era, which can reduce the dependence on traditional fossil energy and reduce energy pressure, and play an important role in achieving carbon neutrality. The triboelectric nanogenerators (TNGs) have attracted widespread interest due to their important applications in micronano energy, self-powered sensing, blue energy, and high-voltage power supply (Luo and Wang, 2020). Recently, there have been a lot of reports on the TENGs in self-powered sensing (Zhang et al., 2020), flexible wearable pending e-skin (Chen et al., 2022), human–machine interfaces (Sun et al., 2021), pressure sensing (Lei et al., 2021a), and hybrid energy harvesting (Xie et al., 2021a).
The triboelectric nanogenerator uses Maxwell displacement current as its internal driving force and converts mechanical energy into electrical energy based on the contact electrification and electrostatic coupling effect (Wang, 2020). Triboelectrification is also known as contact electrification in physics, but the two are not the same. Triboelectrification is the convolution of contact electrification and friction process, whereas contact electrification does not require friction and can cause charge transfer even if two different materials are charged after contact separation (Lin et al., 2019). Previous research has shown that electron transfer is the primary mechanism of contact electrification between different interfaces, which can occur in any material, state, or application process. As a result, studying contact electrification between special interfaces is critical. There are many chemistry reactions, such as electrochemical (Oja et al., 2012; Favaro et al., 2016; Strasser et al., 2018; Magnussen and Gross, 2019) and catalysis (Kolmakov and Moskovits, 2004; Tao and Crozier, 2016; Wang et al., 2022b), happening at special interfaces. Like electrowetting (Li et al., 2016), colloidal suspension (Liufu et al., 2005; Hierrezuelo et al., 2010), photovoltaic effect (Iqbal et al., 2016), adsorption, and corrosion, are related to contact electrification at special interfaces. Therefore, it is very important to understand the contact electrification that occurs at special interfaces. It has the potential to reduce the harm caused by static electricity in everyday life. Figure 1 depicts the mechanism and application of contact electrification at special interfaces.
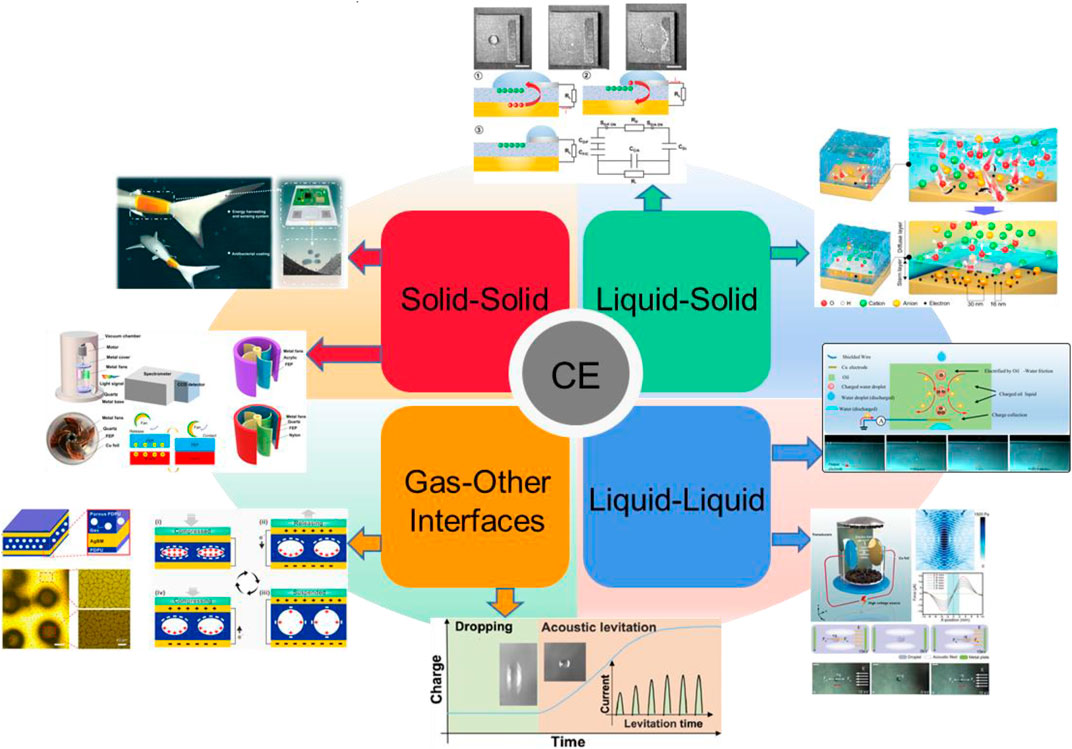
FIGURE 1. Classification and application of special interface contact electrification [reproduced from the work of Xiong et al. (2020), Xu et al. (2020), Li et al. (2021), Tang et al. (2021), Wang et al. (2021), Zhao et al. (2021), Wang et al. (2022a), and Lin et al. (2022) with the permission of the American Association for the Advancement of Science, American Chemical Society, Elsevier Ltd., and the WILEY-VCH Verlag GmbH & Co. KGaA, Weinheim].
In order to better understand the TENG, Li et al. (2019a) established a general method to quantitatively analyze the TENG, which is called edge approximation based equivalent capacitance (EDACE), which can demonstrate the electric field and charge distribution in the TENG and is consistent with the comsol simulation results, and the method provides structural design guidance for the efficient energy harvesting in the TENG. At the same time, Li et al. (2019b) developed the standardized experimental methods and theoretical models for the performance of TENG, and the standardization of TENG is beneficial to the further utilization of TENG and the future commercialization and industrialization. Xie et al. (2021b) summarized and explored the working mechanism of TENG using different materials as intermediates, and proposed an optimization method for the intermediate layer and future challenges, which provided a valuable theoretical basis for improving the maximum effective power output of the TENG.
This review summarizes the contact electrification occurring at different interfaces, including the solid–solid interface, liquid–solid interface, liquid–liquid interface, and gas and other interfaces. First, solid–solid contact electrification is briefly introduced. The overlapping electron cloud model of electron transfer at contact initiation was demonstrated using the photon emission experiments. Then, the application of the solid–solid contact electrification is introduced. Second, the mechanism of electron transfer in the liquid–solid contact electrification is described in detail, as well as the hybrid Electric Double-Layer (EDL) model based on electron transfer and ion adsorption. The applications of TENG for energy collection and self-powered sensing at the liquid–solid interface are summarized. Third, the mechanism of liquid–liquid contact electrification and the application of TENG in the liquid–liquid interface is introduced. Finally, the mechanism of contact electrification between gas and other interfaces is discussed, as well as its application.
Theory Origin and Working Mode of Triboelectric Nanogenerators
The fundamental theoretical basis and source of TENG are derived from the Maxwell’s displacement current, which is distinguished from the normally observed current conducted by free electrons, but due to the time-varying vacuum or medium coupled with the time-varying tiny motion of atomic bound charges and dielectric polarization in materials. In 2017, the expression of the displacement current was expanded. The Maxwell equation for the displacement current is expressed as follows (Wang, 2017):
where
where Ps is mainly due to the presence of surface charge and time variation of boundary shape.
The first term in the formula is the induced current generated by the changing electric field, which is the theory of electromagnetic wave existence. The second term is the current caused by the polarization field generated by the static charge on the surface, which is the fundamental theoretical basis and source of the nanogenerator. This could lead to significant applications in energy and sensing (Wang, 2017).
The TENG is a new type of energy conversion technology. It can collect low-frequency and high-entropy energy from its environment to generate power. When two dielectric materials make contact, the contact surfaces of the two materials are charged by the contact and carry an equal amount of heterogeneous charge. Under the action of mechanical disturbance, the charge can flow in the external circuit to form a current, thus generating the current output and maintaining electrostatic balance. There are four basic working modes of the TENG: vertical contact-separation mode (Wang et al., 2012; Zhu et al., 2012; Zhu et al., 2013), horizontal sliding mode (Wang et al., 2013), single-electrode mode (Yang et al., 2013b), and freestanding triboelectric layer mode (Jiang et al., 2015). With further research, the application of TENG is still based on four basic working modes. Instead of being limited to a single operating mode, multiple operating modes are combined to improve the performance.
Triboelectric Materials for Triboelectric Nanogenerator
Material Selection
Almost all materials, including metal, polymer, and wood, have a triboelectric effect. For the fabrication of the triboelectric nanogenerators, a wide variety of materials are available. The ability of a material to gain or lose electrons is determined by its polarity. The more prominent contrast of electron partiality can prompt the higher triboelectric charge thickness and the electrical contact execution of the TENG. In addition, the materials with appropriate physical and chemical properties (Chen et al., 2016; Zhong et al., 2019), such as corrosion resistance, high-temperature resistance, wear resistance, and hydrophobicity should be selected for the TENG’s working environment. For example, when the TENG is used for marine energy collection, the corrosion resistance of friction materials should be fully considered in order to increase the service life of the TENG.
In addition, the surface topography of the material can be micromachined to improve the contact area and triboelectric properties of the material, such as the preparation of pyramid, square, and hemispherical micronano structures on the surface of the material. Lin et al. (2013) prepared the first water-based friction nanogenerator by the PDMS contact separation with water. PDMS film with a pyramidal structure was prepared by rotating the liquid PDMS elastomer onto a silicon substrate. In the process of PDMS contact separation with water, 82 V open-circuit voltage, peak current density is 1.05 mA/m2, and 60 LEDs can be lit. The experimental results show that the electrical output of PDMS films is improved significantly after surface micromachining. The surface electrification performance of the material can also be improved by incorporating the nanostructure molecules, nanotubes, nanowires, and nanoparticles. Yang et al. (2013a) prepared nanowire arrays on the surface of PDMS for the TENG mechanical energy collection. The energy of the nanowire generator can be stored in a lithium-ion battery or directly used in a self-driven electrocatalytic oxidation system to decompose methyl orange. Scanning electron microscopy revealed that the nanowires had a diameter of 200 nm, that the output voltage of TENG was up to 12 V, and that the output current was around 0.2 μA.
Surface functionalization has the potential to significantly alter surface potential. The nanostructures can be introduced into the surface to change the contact properties of materials, or composite materials can be selected as the contact materials, for example, nanoparticles are embedded in the polymer matrix to change the starting point and dielectric properties of the material surface. Tao et al. (2021a) introduced strong electron-absorbing groups such as the trifluoromethyl group and sulfone group into the main chain of polyimide for modification. The friction charge density of the modified polyimide reached 170 μC m−2, which was four times that of the ordinary polyimide film, and the BaTiO3 nanoparticles were doped in the modified polyimide film, which further improved the charge density and thermoelectric charge stability. It can maintain 32% output performance at 200°C.
Brief Introduction About Solid–Solid Contact Electrification
In the past, the research on contact electrification was mainly focused on the solid–solid contact electrification (Lowell, 1977; Wiles et al., 2004; Byun et al., 2016). In the process of solid–solid contact electrification, the transferred charge type is the electron. Li et al. (2021) investigated the characteristic photon emission spectra of the electrified interfacial atoms, and the researchers used FEP-acrylic acid as an example to find sharp spectral lines with the atomic spectral characteristics under vacuum conditions. The collected photon signals demonstrate the electron cloud overlap model for electron transfer during solid–solid contact. This work provides evidence for the transfer of interfacial electrons from one atom in one material to another atom in another material during CE.
As shown in Figure 2, the electron cloud overlap model is adopted to explain electron transfer (Xu et al., 2018). When two types of atoms are close enough to each other that the distance between them is less than the bonding length, their electron clouds overlap, the potential barrier between the atoms decrease, and the electrons transition from one atom to another resulting in electron transfer. This model accurately describes the phenomenon of contact electrification between different materials and can also be applied to other special interfaces.
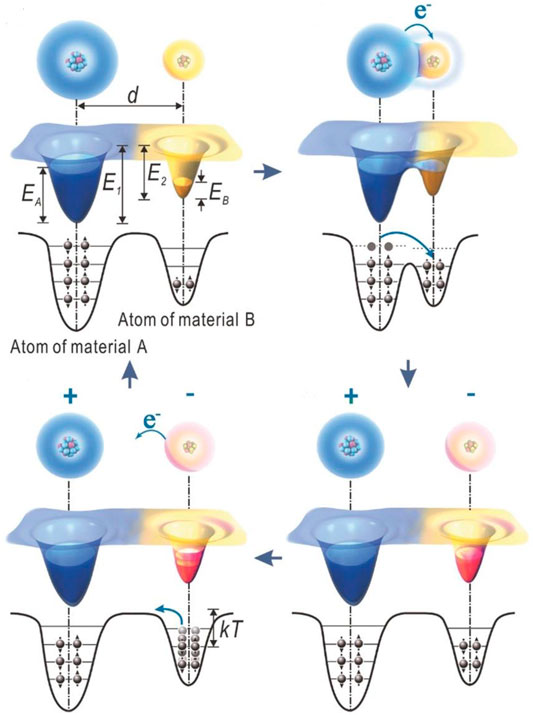
FIGURE 2. Electron cloud overlap model of charge transfer [reproduced with permission from the work of Xu et al. (2018), Copyright 2018 WILEY-VCH Verlag GmbH & Co. KGaA, Weinheim].
The TENG has carried out an in-depth research on the solid–solid contact electrification in the past and has made great achievements in micronano energy (Lei et al., 2019; Shi et al., 2021b), high-voltage power supply (Lei et al., 2020; Lei et al., 2021b), and self-driven sensing (Wang et al., 2019; Shi et al., 2021a; Tian et al., 2021; Yang et al., 2022).
TENG can also be used as an energy converter. Chen et al. (2020) combined the friction nanogenerators with microelectromechanical technology to develop a microfriction electro-echo device. The device has only a 50 μm-sized diaphragm and operates at megahertz frequency, greatly improving the miniaturization and chip integration of the friction nanogenerators.
As shown in Figure 3A, (Wang et al., 2022a) first applied the nanogenerator device to the field of fish movement monitoring. A wearable fish motion data monitoring platform (FDSP), that can study the kinematics of fish by real-time wireless sensor technology, is proposed. FDSP is composed of gasbag TENG (AS-TENG), antibacterial nanocoating, and a wireless signal transceiver module, which can realize the remote monitoring of fish movement. The output performance of AS-TENG underwater is greatly improved by using a gasbag structure that reduces the shielding effect of water and PTFE dielectric film treated by magnetron sputtering. The AS-TENG, on the other hand, also can be used as a kind of highly sensitive motion sensor, the output voltage can reflect the real-time behavior of the tail, the wireless signal transceiver module under the action of the sensing data transmission to the receiver, at the receiving end can get fish movement parameters in detail, including the swing angle and swing frequency, or even a typical swing gesture. FDSP has greatly expanded the applicability of TENGs as a wearable electronic product underwater, and it has broad application prospects in the construction of wearable tracking devices, underwater robots, and underwater wireless sensor networks (UWSN). As shown in Figure 3B, Lei et al. (2020) proposed a triboelectric nanogenerator (TENG) with a charge accumulation strategy. As a high-voltage power supply, it can achieve continuous ultra-high-voltage output and 8000 LED lights simultaneously. The calculated output voltage is over 20 kV, which is the highest voltage output realized by TENG. The device can also be used to prepare ozone from high-pressure air for sewage treatment. As a kind of high-voltage power supply, TENG has a wide range of applications in electrostatic treatment and air pollution treatment.
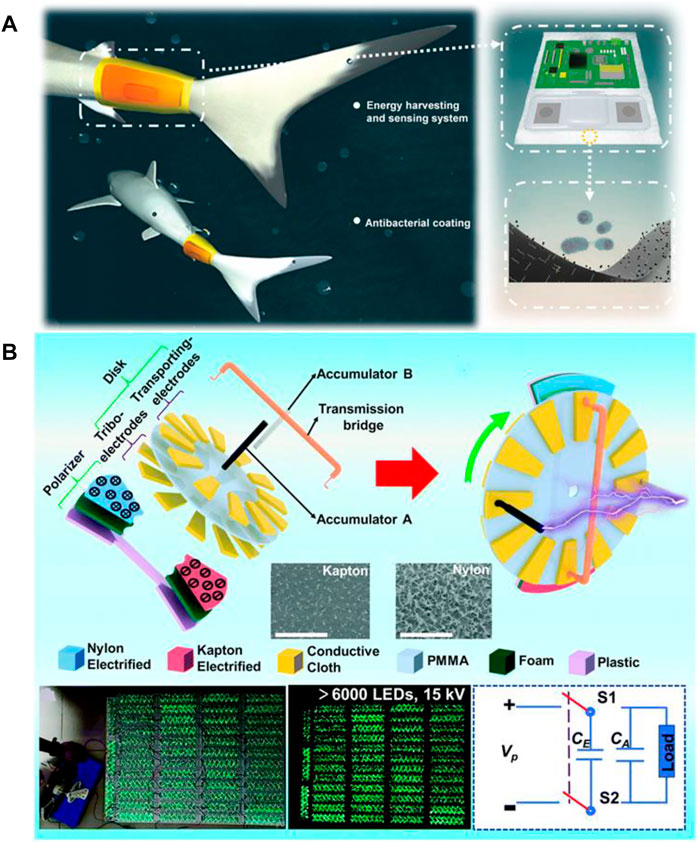
FIGURE 3. (A) Underwater energy harvesting and fish behavior monitoring devices [reproduced with permission from the work of Wang et al. (2022a), Copyright 2022 WILEY-VCH Verlag GmbH & Co. KGaA, Weinheim]. (B) Continuous high-voltage power supply based on charge accumulation [reproduced with permission from the work of Lei et al. (2020), Copyright 2020 The Royal Society of Chemistry].
Liquid–Solid Contact Electrification
Mechanism
People have known about the solid–solid contact electrification, and there has been a large number of literature reports on the electrification mechanism and application. People believe that the solid–solid contact electrification is caused by electron transfer, and this is not disputed.
In a study of liquid–solid electrification dating back to a world in the 80 s, E I - Kazzaz and others found that when the liquid metal is in contact with the insulator, it can be generated on the insulator surface electric charge (El-Kazzaz and Rose-Innes, 1985), and then found that when water is in contact with the polymer, it would produce electric charge (Matsui et al., 1993; Yatsuzuka et al., 1994; Yatsuzuka et al., 1996), because the water charge type is complicated. However, the mechanism of liquid–solid contact electrification is always a source of contention. Many people believe that the contact electrification between water and polymer is caused by ions (Diaz, 1998; McCarty and Whitesides, 2008; Zhang et al., 2015). However, the recent emergence of the liquid–solid contact triboelectric nanogenerators poses a challenge to the previous liquid–solid contact electrification caused by ion transfer. As shown in Figure 4A, Zhan et al. (2020) prepared a droplet polymer TENG, which generated electrical signals when the droplets slid on the inclined PTFE surface. The droplets continued to drop when the charge on the PTFE surface gradually reached saturation. Then, the PTFE was fully immersed in the ion-rich solution, and then the contact with water droplets was found to still have contact electrification. Experiments with pure water and droplets with high ion concentrations revealed that the output electrical signal became weak. It was claimed that surface ion adsorption was not the most important factor in contact electrification and that ions would inhibit charge transmission. This indicates that the electrification of liquid–solid contact is dominated by electrons with the participation of ions. As shown in Figure 4B, Lin et al. (2020b) explored the contact electrification between the solid and liquid through a thermal-electron emission experiment. By comparing the charge amount on a solid surface before and after the experiment, they found that there was both electron transfer and ion transfer in the liquid–solid contact surface. The presence of an ion would inhibit electron transfer, and ion transfer would be affected by the pH of the solution. Furthermore, the results of the experiments show that ion transfer is dominant when the solid surface is hydrophilic. When a solid surface is hydrophobic, electron transfer takes precedence.
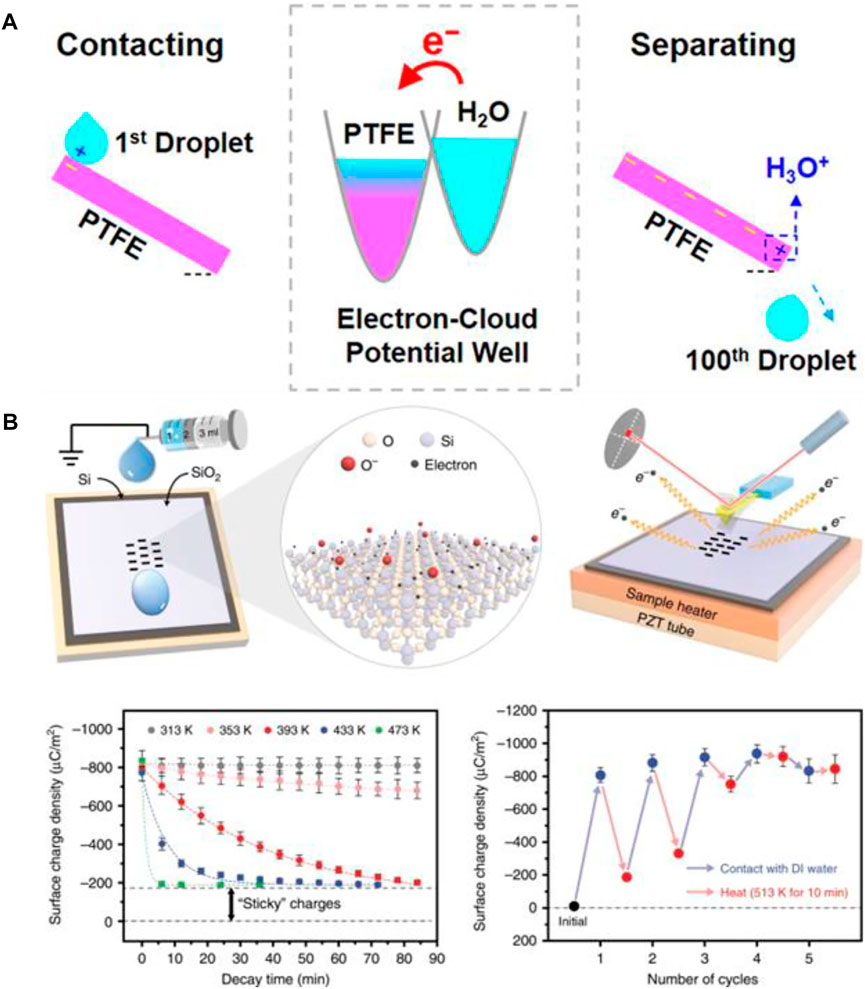
FIGURE 4. (A) Electron transfer of droplets in contact with the PTFE [reproduced with permission from the work of Zhan et al. (2020), Copyright 2020 American Chemical Society]. (B) Thermal-electron emission experiments at the liquid–solid interface [reproduced with permission from the work of Lin et al. (2020b), Copyright 2020 Springer Nature].
As shown in Figure 5A, Li et al. (2020) found through the polymer-water-polymer experiments that when PTFE and FEP were used, the contact area between water and polymer increased, the CE charge of PTFE film increased from 1.2 to 3.2 nC, and that of FEP film increased from 2.1 to 4.3 nC, whereas ion adsorption did not lead to friction-induced charge increase. Thus, the charge increase is due to electron transfer caused by the electron-absorbing groups on the polymer surface. As shown in Figure 5B,C, Nie et al. (2020) used the PTFE extruding droplet to investigate the liquid–solid electrification mechanism of PTFE and deionized water contact the electric charge generated by 10 times higher than the ion transfer model and demonstrate its contact electrification is dominated by electrons and ions. As the ion concentration in aqueous solution increases, there will be ion shield, which leads to reduced charge transfer. However, contact electrification still occurs after replacing the deionized water with organic matter, indicating that contact electrification can be caused by electron transfer.
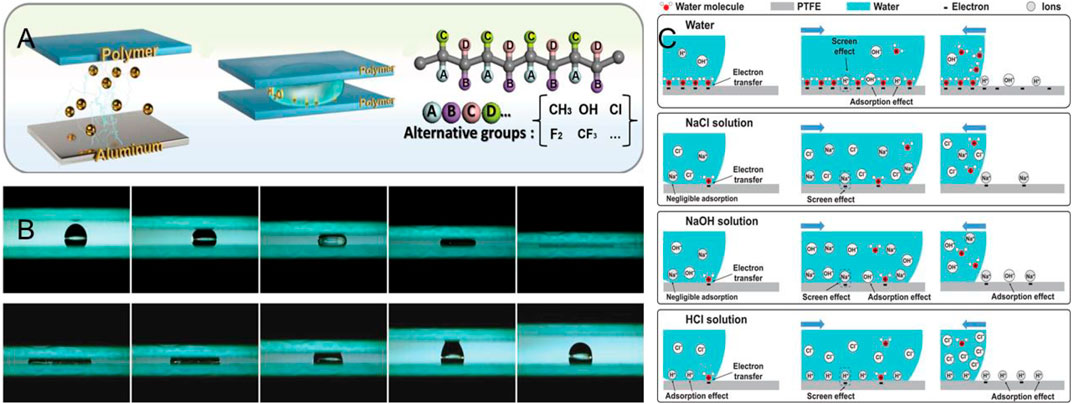
FIGURE 5. (A) Experiment of polymer extrusion droplet with a strong electron-absorbing ability [reproduced with permission from the work of Li et al. (2020), Copyright 2020 WILEY-VCH Verlag GmbH & Co. KGaA, Weinheim]. (B) Double-layer PTFE extruded droplets [reproduced with permission from the work of Nie et al. (2020), Copyright 2019 WILEY-VCH Verlag GmbH & Co. KGaA, Weinheim]. (C) Mechanism of contact electrification between water and PTFE [reproduced with permission from the work of Nie et al. (2020), Copyright 2019 WILEY-VCH Verlag GmbH & Co. KGaA, Weinheim].
As shown in Figure 6A, Wang et al. (2022b) added 20 mg FEP powder to 50 ml methyl orange solution, and after the powder was evenly distributed in the solution, the methyl orange solution was discolored after 3 h ultrasonic treatment. The experimental results demonstrated that the contact electro-catalysis theory was well supported, with the electrons transferred during liquid–solid contact in the fading of methyl orange. As shown in Figure 6B, Zheng et al, (Lin et al., 2020a; Zheng et al., 2021) also on semiconductor contact between liquid and electricity, when water droplets on the surface of the semiconductor slide, due to the Fermi level differences between the water molecules and semiconductor, there will be the electric field between sliding when water molecules, water molecules, and semiconductor between the formation of the chemical bond rupture and releasing energy quantum. On the experimental surface, the liquid–solid contact electrification is not only caused by ion transfer but is dominated by electron transfer and involves ion transfer. An interesting phenomenon was also found in the experiments. When the water droplets rolled on the tilted PTFE film, the saturation charge density of the PTFE could be increased by using UV light irradiation, which can be well explained by Wang’s electron transfer model (Tao et al., 2021b).
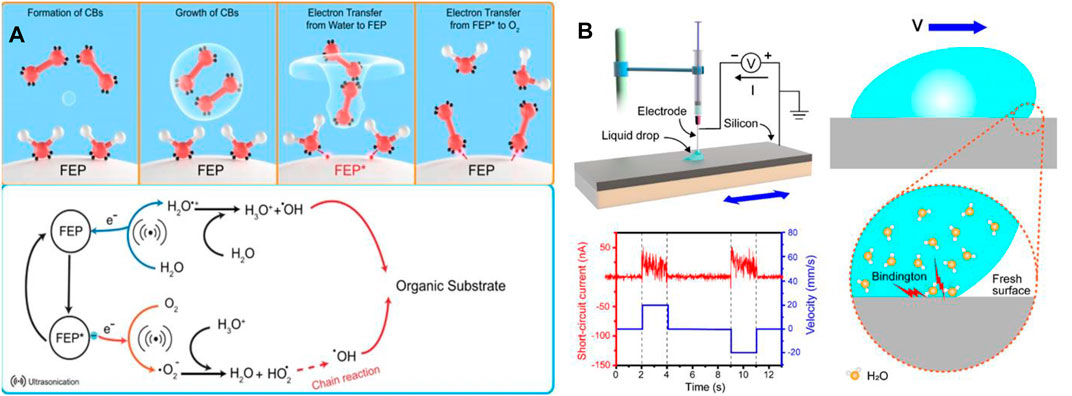
FIGURE 6. (A) Liquid–solid contact electron transfer in contact electro-catalysis [reproduced with permission from the work of Wang et al. (2022b), Copyright 2022 Springer Nature]. (B) Tribovoltaic effect on the interface between semiconductor and droplet [reproduced with permission from the work of Lin et al. (2020a) and Zheng et al. (2021), Copyright 2020 Elsevier Ltd., 2021 WILEY-VCH Verlag GmbH & Co. KGaA, Weinheim].
Hybrid Electric Double Layer
Wang proposed Wang’s model, which differs from the traditional electric double-layer theory, also known as the hybrid electric double-layer theory, in order to better understand the mechanism of the liquid–solid contact electrification. The traditional electric double-layer theory, as shown in Figures 7A, is to adsorb a layer of ions on the solid surface, which will attract ions of different charges and repel ions of the same charge, forming an electric double layer. As shown in Figure 7B, electron transfer in the liquid–solid contact is taken into account in the hybrid electric bilayer theory (Lin et al., 2022). Ions are formed at the liquid–solid interface as a result of electron transfer, and the ions at the solid-phase interface will have coulombic interactions with the anion and anion in solution, resulting in a potential gradient distribution at the liquid–solid interface.
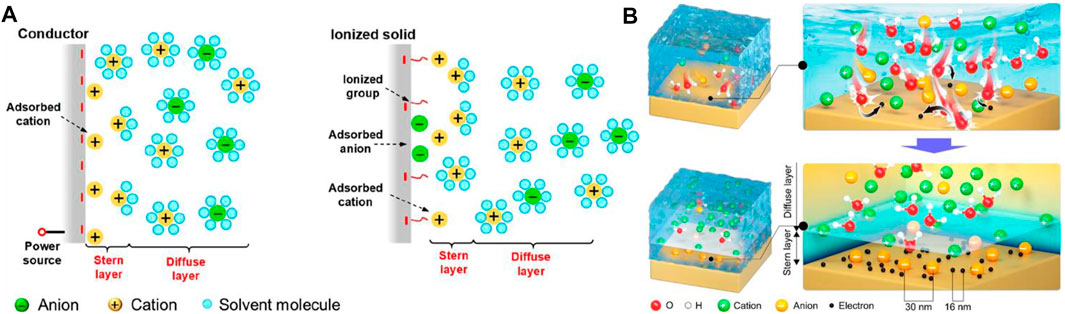
FIGURE 7. (A) Traditional EDL model (Lin et al., 2022). (B) Hybrid EDL model (Lin et al., 2022) [reproduced with permission from the work of Lin et al. (2022), Copyright 2021 American Chemical Society].
Applications
There are numerous water resources in our lives, such as oceans, rivers, lakes, rainwater, and so on. These water resources are accompanied by a significant amount of mechanical energy. The TENG is a new power generation method that converts mechanical energy into electrical energy and has the advantages of being small in size, low in cost, simple in structure, and environmental friendly. As a result, the triboelectric nanogenerator based on the liquid–solid contact has a wide range of applications in everyday life. It can be used to collect ocean energy, collect raindrop energy, self-drive sensing, and micro and nano energy.
In terms of energy collection, Xu et al. (2020) created a liquid–solid triboelectric nanogenerator (TENG) based on water droplets to achieve a high instantaneous energy output density. A PTFE film was coated on an ITO substrate and an aluminum electrode structure was installed. When the water droplets hit the PTFE film, the maximum voltage output could reach 143.5 V and light up more than 100 LED lamps due to the formation of a closed circuit. The instantaneous power density was several orders of magnitude higher than before. Zhang et al. (2021), as shown in Figure 8A, introduced an electric double-layer capacitor at the liquid–solid contact electrification interface of DEG, and established an equivalent circuit model of DEG with a switching effect, achieving a power output of two orders of magnitude higher than the previous electrostatic induction power generation without polarizing the solid surface. As shown in Figure 8B, Qin et al. (2022) realized a double-layer nanogenerator (EDL-NG) by introducing the oil phase in the water–solid interface. Through the oil phase, the generator sweeps up ions in the water–solid interface double layer. It can operate efficiently in a variety of water environments, is energy-dense, and can be used directly for underwater energy collection and self-driven sensing without encapsulation. Liu et al. (2022) proposed a ferrofluid-based triboelectric tactile sensor based on solid–liquid contact electrification, which achieved an ultra-high sensitivity of 21.48 kPa−1. Li et al. (2022) proposed a new type of triboelectric generator (B-TENG) based on the moving bubbles in the liquid, which has a simple structure and stable and reliable operation performance. When a 1.08 cm3 bubble moves slowly in a 1 cm inner diameter polytetrafluoroethylene (PTFE) tube filled with deionized water, B-TENG can generate an open-circuit voltage of up to 17.5 V, while remaining stable after 600 working cycles. It has a high mechanical energy collection capability as well as fast and sensitive water level monitoring capabilities.
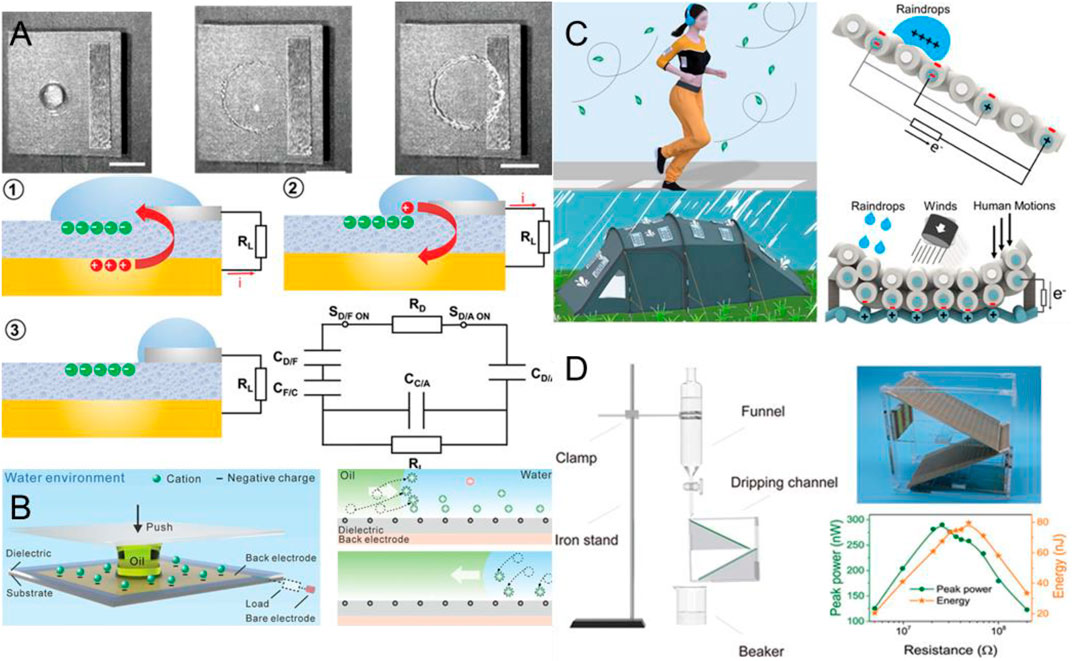
FIGURE 8. (A) A Single-Droplet Electricity Generator Achieves an Ultrahigh Output Over 100 V Without Pre-Charging [reproduced with permission from the work of Zhang et al. (2021), Copyright Wiley-VCH GmbH]. (B) Underwater Energy Harvesting and Sensing by Sweeping Out the Charges in an Electric Double Layer Using an Oil Droplet [reproduced with permission from the work of Qin et al. (2022), Copyright 2022 WILEY-VCH Verlag GmbH & Co. KGaA, Weinheim]. (C) Textile TENG for harvesting raindrop energy [reproduced with permission from the work of Gang et al. (2021), Copyright 2021 American Chemical Society]. (D) L-S TENG with folded structure and grating to harvest energy and sensor [reproduced with permission from the work of Zhong et al. (2020), Copyright 2020 American Chemical Society].
In terms of liquid–solid contact electrification based triboelectric nanogenerator for water drop energy and ocean energy collection, as shown in Figure 8C, Gang et al. (2021) prepared textile TENG which can collect raindrop energy and generate a voltage of 4.3 V and a current of 6 μA. Li et al. (2018) prepared a floating liquid–solid triboelectric nanogenerator for marine energy collection. The energy output of this TENG is 48.7 times that of the solid–solid triboelectric nanogenerator of the same volume. The TENG network can output 290 μA, 16725 nC, 300 V, and can light 100s of LED lamps. As shown in Figure 8D, Zhong et al. (2020) designed a liquid–solid contact electrification TENG with a single electrode and grating electrode. The TENG was designed with a folded structure that can effectively improve the energy density, and the droplet information can be recorded as a sensor when the droplet flows through the grating. Xu et al. (2019) fabricated a highly sensitive wave sensor based on the liquid–solid contact electrification by covering a Cu electrode on a microstructured PTFE surface. With a sensitivity of 23.5 mV/mm and the ability to monitor millimeter-level waves, the sensor can be used for ocean wave detection. Simultaneously, a liquid–solid contact electrification can be used to reduce the explosion of petrochemical products caused by the liquid–solid contact electrification during tanker transportation by coating antistatic coating or adding ionic liquids to petroleum products to reduce the liquid–solid contact electrification to ensure safety.
Liquid–Liquid Contact Electrification
Mechanism
As a special interface contact electrification, the liquid–liquid contact electrification differs from the solid–solid contact electrification and liquid–solid contact electrification. The liquid–liquid contact electrification is difficult to detect and study due to a lack of appropriate detection technology.
Recently, as shown in Figure 9A, Jiang et al. (2019) used a single-electrode-based TENG as a probe. The electrode was slowly inserted into the oil/water interface, and charges could be detected on the interface by measuring the current when the electrode was inserted. However, this work shows that TENG can be used as a probe for detection. Therefore, the size of TENG can be reduced to the tip shape and the scanning method can be used as much as possible. A new type of probe can be developed to study the charge behavior at the liquid–liquid interface.
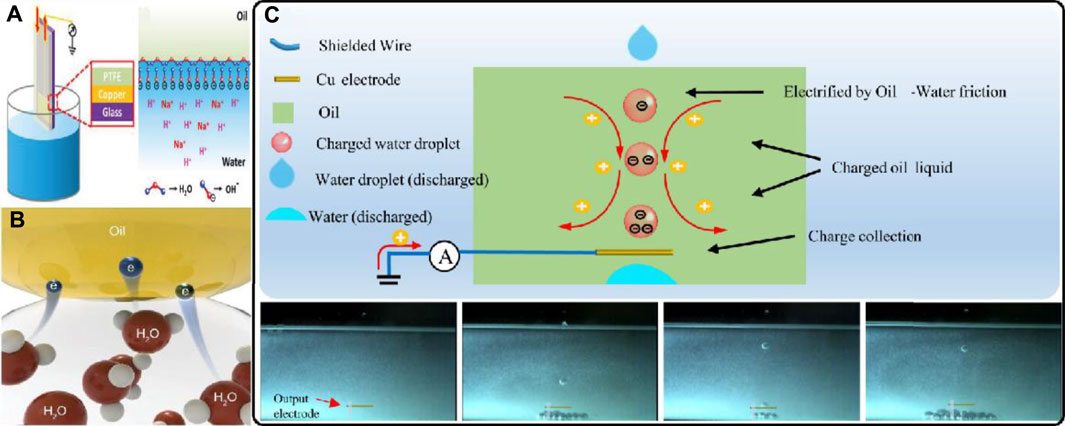
FIGURE 9. (A) Oil–water–solid multiphase interface TENG [reproduced with permission from the work of Jiang et al. (2019), Copyright 2019 WILEY-VCH Verlag GmbH & Co. KGaA, Weinheim]. (B) Quantifying charge transfer in the liquid–liquid contact electrification [reproduced with permission from the work of Tang et al. (2021), Copyright 2021 WILEY-VCH Verlag GmbH & Co. KGaA, Weinheim]. (C) The mechanism of contact electrification between oil droplets and water [reproduced with permission from the work of Zhao et al. (2021), Copyright 2020 Elsevier Ltd.].
Tang et al. (2021) cleverly designed a noncontact measurement method combining the electric field and sound field to quantify droplet charges, suspended droplets by field acoustic emitters, and used this method to study charge transfer behavior at the liquid–solid and liquid–liquid contact interfaces, as shown in Figure 9B. This method eliminates container interference, and for the liquid–solid interface, it is discovered that the amount of charge transfer between the solid and liquid decreases as ion concentration in the droplet increases, which is consistent with the previous reports. More importantly, at the liquid–liquid interface, the contact between the silicone oil droplets and different solutions such as DI water droplets, NaCl droplets, HCl droplets, and NaOH droplets is charged, with inconsistent positive and negative charge transfer behaviors. This asymmetric charge transfer phenomenon supports the existence of electron transfer at the liquid–liquid interface.
As shown in Figure 9C, Zhao et al. (2021) established a liquid–liquid TENG by dropping water into insoluble transformer oil. By using the effect of gravity and the difference in molecular mass, the droplet can “freely fall” without the influence of external force, and the L–L contact electrification energy collection system is realized. A droplet of 25 μl falling through a 40 mm thick organic liquid can produce a maximum output charge of—5.3pC. Water molecules have the ability to absorb electrons from oil molecules. The relative displacement distance can be altered by varying the initial velocity of the DI water drop, and the amount of transferred charge can then be altered. The transfer charge on the droplet surface gradually decreases and eventually reaches saturation as the droplet descends. To explain the effect of the liquid–liquid contact electrification, the “Wang transition” model is used. The transfer charge of the liquid–liquid interface decreased significantly as the concentration of H+ in the droplet increased, indicating that the presence of electron transfer may be the most important factor influencing the CE interface. In addition, with the increase in temperature, the cumulative transfer charge increases at the liquid–liquid interface, which is quite different from the case of solid electrification. The main reason for this phenomenon is the decrease in viscosity caused by high temperatures. As shown in Figure 9C, Zhao et al. (2021) proposed the liquid–liquid double electric layer model. When the water droplets contact transformer oil, the molecules and ions in the two liquid phases collide at the oil–water interface due to thermal motion and surrounding liquid pressure. In the process of impact, electron transfer and ion reaction occur simultaneously on the transformer oil surface. According to the pH test results, the electron transfer effect is much larger than the adsorption of H+ on the oil/water interface, and some anions in an aqueous solution are attracted to migrate to the interface due to electrostatic interaction, forming an EDL layer at the interface. Furthermore, electrons transferred from the oil molecules to water molecules cannot stay on the surface. Electrons combine with the water molecules to form water and electrons, and the unstable hydrated electrons will convert to OH− and H+, increasing the OH− concentration at the liquid–liquid interface. The abovementioned experimental results indicate that both electrons and ions are involved in the process of liquid–liquid contact electrification, in which electron transfer is dominant.
Applications
Contact electrification at the liquid–liquid interface can be applied to monitor chemical reactions between liquids and changes in the liquid composition. However, the liquid–liquid interface is easy to merge and difficult to separate when contacting, and the interface is complicated. There are few studies on the liquid–liquid interface in the TENGs. As shown in Figure 10, Nie et al. (2019) proposed to use contact separation between the liquid membrane and droplet to realize energy collection of TENGs at the liquid–liquid interface. When 40 μl droplets passed through the membrane, the laboratory grounded the liquid membrane, which collected the static charge on the surface of the droplets, and precharged the membrane, which generated a peak power of 137.4 nW. In addition, this TENG can also be used for mechanical energy collection in raindrops, irrigation streams, and microfluidics.
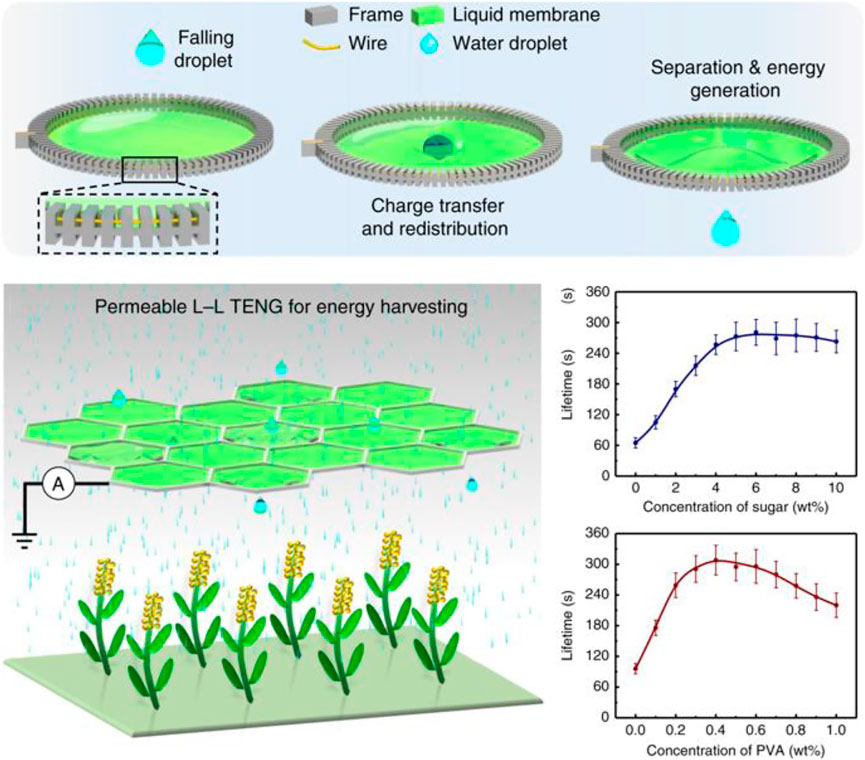
FIGURE 10. The application between the liquid membrane and water droplet [reproduced with permission from the work of Nie et al. (2019), Copyright 2019 Springer Nature].
Gas and Other Interface Contact Electrification
Mechanism
Contact electrification can also occur between gas and other interfaces, such as the gas–solid interface and gas–liquid interface. Because the materials for contact electrification are gas and solid, the charge transfer type is single for the gas–solid contact interfaces. The charge type transferred during the gas–solid contact electrification is the electron, according to the electron cloud overlap model. However, due to the complexity of liquid phase composition, low density of the gas, and low fluidity of a liquid, sufficient friction cannot be generated at the contact interface between the two, and thus few studies on the gas–liquid contact electrification have been conducted in the past. As shown in Figure 11, Wang et al. (2021) realized the controllable frictional motion between the droplets and air by using ultrasonic control of droplet suspension through a portable ultrasonic suspension device. When the water droplets are suspended in air and friction occurs, the droplet surface produces a positive charge. The volume of 20 drops of water floating in the air increases with time. When the suspension time is reduced to 30 s, the droplet surface charge amount reaches saturation. When the droplets are mixed and suspended with PTFE powder, nylon powder and BaTiO3 powder respectively, the droplets will take on different types of charges. When NaCl is dissolved in the droplet and the ion concentration of the droplet increases, the droplet suspension will inhibit the generation of friction charge on the surface of the droplet due to the ion shielding effect. Therefore, it can be seen that both ions and electrons are involved in the electrification of the gas–liquid contact, in which electrons play a leading role.
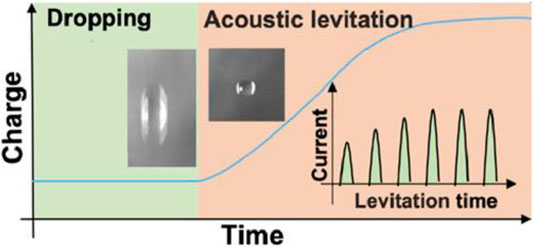
FIGURE 11. Study about the gas–liquid contact electrification [reproduced with permission from the work of Wang et al. (2021), Copyright 2021 American Chemical Society].
Applications
Contact electrification between gas and other interfaces has significant application value in our daily lives. Understanding the gas–liquid interface contact electrification mechanism can help us understand the gas–liquid interface charge accumulation and dissipation, which can help us collect electricity from the atmosphere, while also allowing us to study other related areas such as electrostatic hazard prevention, rain energy collection, friction power, surface engineering, and so on. Many studies have also been conducted on the use of gas–solid contact electrification.
As shown in Figure 12, Xiong et al. (2020) developed a gas–solid contact charged friction nanogenerator (GS-TENG) and prepared a soft porous self-healing and elastomer polysiloxane dimethyl acetaloxime polyurethane (PDPU). PDPU has a controllable seal gap to capture the gas, and PDPU interacts with the captured gas to produce electron transfer. Through periodic compression, electrons can be transferred from gas to polymer, and GS-TENG can perform various mechanical movements. It can be used as wearable power for textiles, shoes, mice, and other devices, as well as a self-powered skin sensor for monitoring the finger movement and walking posture analysis. Xue et al. (2017) pointed out that water evaporation can harvest energy from the surroundings, when the water vapor and carbon materials on the surface of the nanostructures contact can generate electricity. cm size of carbon black can produce when in contact with water vapor 1 V voltage output, experimental proof cm thick millimeter size carbon black can produce when in contact with water vapor and standard AA batteries are voltage output, It can be used for sterilization, water purification, water desalination, and large-scale application in warm areas.
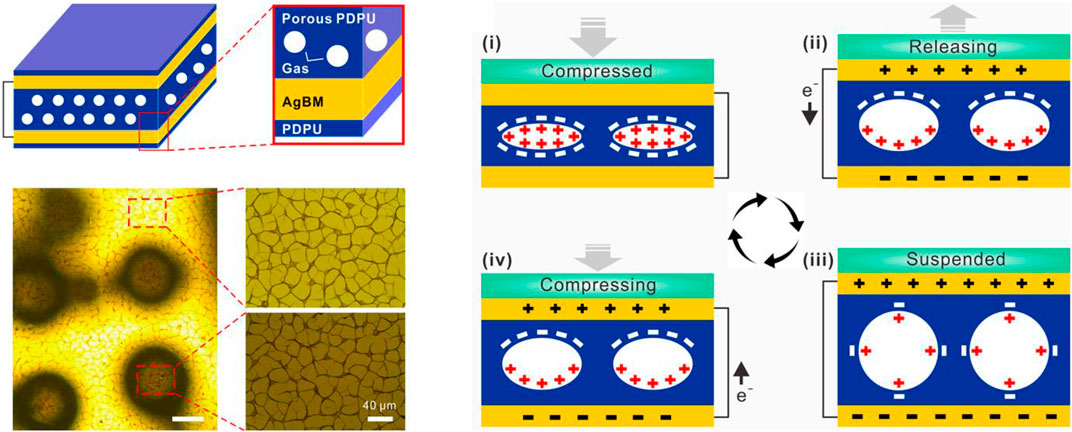
FIGURE 12. Contact electrification between PDPU and gas [reproduced with permission from the work of Xiong et al. (2020), Copyright 2020 American Association for the Advancement of Science].
Summary and Perspective
In summary, we have presented the contact electrification phenomena that occur at special interfaces, summarizing and generalizing the electrification mechanisms and applications of contact electrification at the solid–solid, liquid–solid, liquid–liquid, and gas-participating interfaces, respectively. In this work, several conclusions have been obtained as follows:
(1) In the solid–solid contact electrification, the electrification is due to the transfer of electrons between two materials in contact with each other.
(2) In the liquid–solid contact electrification, both electron transfer and ion transfer are involved, with electron transfer playing a dominant role. The hybrid EDL theory, which differs from the traditional EDL theory, is proposed to explain the liquid–solid contact electrification theory.
(3) In the liquid–liquid contact electrification, both ions and electrons are involved in contact electrification, and electron transfer is the main cause of the liquid–liquid contact electrification.
(4) In the contact electrification of gas and other interfaces, where the gas–solid contact electrification is caused by electron transfer, and the gas–liquid contact electrification is caused by both electrons transfer and ions transfer, where electron transfer plays a major role.
Although there has been some progress in understanding the contact electrification between special interfaces, there are still significant gaps. The phenomenon of contact electrification between different interfaces pervades our lives, and there are still many possibilities for future applications. This work can help us to understand the mechanism of contact electrification and the progress we have made. Understanding these can broaden the application of TENG at these interfaces to improve energy utilization and can be used in fields such as micro and nano energy, self-driven sensing, electrostatic hazards elimination, surface engineering, and micro and nano fluids.
Data Availability Statement
The original contributions presented in the study are included in the article/Supplementary Material. Further inquiries can be directed to the corresponding author.
Author Contributions
WZ wrote the manuscript. WZ and YS contributed equally to this work. All authors contributed to the choice of the review topic, to the general outline of the manuscript, and to the revision and approval of the manuscript to be submitted.
Funding
This work was supported by the National Natural Science Foundation of China (No. 61774084), the Fundamental Research Funds for the Central Universities (No. NG2019003), the special fund of Jiangsu Province for the transformation of scientific and technological achievements (No. BA2017032), the Postgraduate Research & Practice Innovation Program of Jiangsu Province (No. KYCX18_0273), and a Project Funded by the Priority Academic Program Development of Jiangsu Higher Education Institutions.
Conflict of Interest
The authors declare that the research was conducted in the absence of any commercial or financial relationships that could be construed as a potential conflict of interest.
Publisher’s Note
All claims expressed in this article are solely those of the authors and do not necessarily represent those of their affiliated organizations, or those of the publisher, the editors, and the reviewers. Any product that may be evaluated in this article, or claim that may be made by its manufacturer, is not guaranteed or endorsed by the publisher.
References
Atzori, L., Iera, A., and Morabito, G. (2010). The Internet of Things: A Survey. Comput. Netw. 54 (15), 2787–2805. doi:10.1016/j.comnet.2010.05.010
Byun, K.-E., Cho, Y., Seol, M., Kim, S., Kim, S.-W., Shin, H.-J., et al. (2016). Control of Triboelectrification by Engineering Surface Dipole and Surface Electronic State. ACS Appl. Mat. Interfaces 8 (28), 18519–18525. doi:10.1021/acsami.6b02802
Chen, C., Wen, Z., Shi, J., Jian, X., Li, P., Yeow, J. T. W., et al. (2020). Micro Triboelectric Ultrasonic Device for Acoustic Energy Transfer and Signal Communication. Nat. Commun. 11 (1), 4143. doi:10.1038/s41467-020-17842-w
Chen, X., Jiang, T., Yao, Y., Xu, L., Zhao, Z., and Wang, Z. L. (2016). Stimulating Acrylic Elastomers by a Triboelectric Nanogenerator - toward Self-Powered Electronic Skin and Artificial Muscle. Adv. Funct. Mat. 26 (27), 4906–4913. doi:10.1002/adfm.201600624
Chen, Y., Gao, Z., Zhang, F., Wen, Z., and Sun, X. (2022). Recent Progress in Self‐powered Multifunctional E‐skin for Advanced Applications. Exploration 2 (1), 20210112. doi:10.1002/exp.20210112
Diaz, A. F. (1998). Contact Electrification of Materials: The Chemistry of Ions on Polymer Surfaces. J. Adhesion 67 (1-4), 111–122. doi:10.1080/00218469808011102
El-Kazzaz, A., and Rose-Innes, A. C. (1985). Contact Charging of Insulators by Liquid Metals. J. Electrost. 16 (2), 157–163. doi:10.1016/0304-3886(85)90039-7
Fan, F.-R., Tian, Z.-Q., and Lin Wang, Z. (2012). Flexible Triboelectric Generator. Nano Energy 1 (2), 328–334. doi:10.1016/j.nanoen.2012.01.004
Favaro, M., Jeong, B., Ross, P. N., Yano, J., Hussain, Z., Liu, Z., et al. (2016). Unravelling the Electrochemical Double Layer by Direct Probing of the Solid/liquid Interface. Nat. Commun. 7, 12695. doi:10.1038/ncomms12695
Gang, X., Guo, Z. H., Cong, Z., Wang, J., Chang, C., Pan, C., et al. (2021). Textile Triboelectric Nanogenerators Simultaneously Harvesting Multiple "High-Entropy" Kinetic Energies. ACS Appl. Mat. Interfaces 13 (17), 20145–20152. doi:10.1021/acsami.1c03250
Hierrezuelo, J., Sadeghpour, A., Szilagyi, I., Vaccaro, A., and Borkovec, M. (2010). Electrostatic Stabilization of Charged Colloidal Particles with Adsorbed Polyelectrolytes of Opposite Charge. Langmuir 26 (19), 15109–15111. doi:10.1021/la102912u
Iqbal, A., Hossain, M. S., and Bevan, K. H. (2016). The Role of Relative Rate Constants in Determining Surface State Phenomena at Semiconductor-Liquid Interfaces. Phys. Chem. Chem. Phys. 18 (42), 29466–29477. doi:10.1039/c6cp04952d
Jiang, P., Zhang, L., Guo, H., Chen, C., Wu, C., Zhang, S., et al. (2019). Signal Output of Triboelectric Nanogenerator at Oil-Water-Solid Multiphase Interfaces and its Application for Dual‐Signal Chemical Sensing. Adv. Mat. 31 (39), 1902793. doi:10.1002/adma.201902793
Jiang, T., Chen, X., Han, C. B., Tang, W., and Wang, Z. L. (2015). Theoretical Study of Rotary Freestanding Triboelectric Nanogenerators. Adv. Funct. Mat. 25 (19), 2928–2938. doi:10.1002/adfm.201500447
Kolmakov, A., and Moskovits, M. (2004). Chemical Sensing and Catalysis by One-Dimensional Metal-Oxide Nanostructures. Annu. Rev. Mat. Res. 34, 151–180. doi:10.1146/annurev.matsci.34.040203.112141
Lei, H., Chen, Y., Gao, Z., Wen, Z., and Sun, X. (2021a). Advances in Self-Powered Triboelectric Pressure Sensors. J. Mat. Chem. A 9 (36), 20100–20130. doi:10.1039/d1ta03505c
Lei, R., Shi, Y., Ding, Y., Nie, J., Li, S., Wang, F., et al. (2020). Sustainable High-Voltage Source Based on Triboelectric Nanogenerator with a Charge Accumulation Strategy. Energy Environ. Sci. 13 (7), 2178–2190. doi:10.1039/d0ee01236j
Lei, R., Shi, Y., Wang, X., Tao, X., Zhai, H., and Chen, X. (2021b). Water Purification System Based on Self-Powered Ozone Production. Nano Energy 88, 106230. doi:10.1016/j.nanoen.2021.106230
Lei, R., Zhai, H., Nie, J., Zhong, W., Bai, Y., Liang, X., et al. (2019). Butterfly-inspired Triboelectric Nanogenerators with Spring-Assisted Linkage Structure for Water Wave Energy Harvesting. Adv. Mat. Technol. 4 (3), 1800514. doi:10.1002/admt.201800514
Li, C., Liu, X., Yang, D., and Liu, Z. (2022). Triboelectric Nanogenerator Based on a Moving Bubble in Liquid for Mechanical Energy Harvesting and Water Level Monitoring. Nano Energy 95, 106998. doi:10.1016/j.nanoen.2022.106998
Li, D., Xu, C., Liao, Y., Cai, W., Zhu, Y., and Wang, Z. L. (2021). Interface Inter-atomic Electron-Transition Induced Photon Emission in Contact-Electrification. Sci. Adv. 7(39), eabj0349. doi:10.1126/sciadv.abj0349
Li, S., Nie, J., Shi, Y., Tao, X., Wang, F., Tian, J., et al. (2020). Contributions of Different Functional Groups to Contact Electrification of Polymers. Adv. Mat. 32 (25), 2001307. doi:10.1002/adma.202001307
Li, X., Lau, T. H., Guan, D., and Zi, Y. (2019a). A Universal Method for Quantitative Analysis of Triboelectric Nanogenerators. J. Mat. Chem. A 7 (33), 19485–19494. doi:10.1039/c9ta06525c
Li, X., Tao, J., Wang, X., Zhu, J., Pan, C., and Wang, Z. L. (2018). Networks of High Performance Triboelectric Nanogenerators Based on Liquid-Solid Interface Contact Electrification for Harvesting Low-Frequency Blue Energy. Adv. Energy Mat. 8 (21), 1800705. doi:10.1002/aenm.201800705
Li, X., Tian, H., Shao, J., Ding, Y., Chen, X., Wang, L., et al. (2016). Decreasing the Saturated Contact Angle in Electrowetting-On-Dielectrics by Controlling the Charge Trapping at Liquid-Solid Interfaces. Adv. Funct. Mat. 26 (18), 2994–3002. doi:10.1002/adfm.201504705
Li, X., Xu, G., Xia, X., Fu, J., Huang, L., and Zi, Y. (2019b). Standardization of Triboelectric Nanogenerators: Progress and Perspectives. Nano Energy 56, 40–55. doi:10.1016/j.nanoen.2018.11.029
Lin, S., Chen, X., and Wang, Z. L. (2022). Contact Electrification at the Liquid-Solid Interface. Chem. Rev. 122 (5), 5209–5232. doi:10.1021/acs.chemrev.1c00176
Lin, S., Chen, X., and Wang, Z. L. (2020a). The Tribovoltaic Effect and Electron Transfer at a Liquid-Semiconductor Interface. Nano Energy 76, 105070. doi:10.1016/j.nanoen.2020.105070
Lin, S., Xu, L., Chi Wang, A., and Wang, Z. L. (2020b). Quantifying Electron-Transfer in Liquid-Solid Contact Electrification and the Formation of Electric Double-Layer. Nat. Commun. 11 (1), 399. doi:10.1038/s41467-019-14278-9
Lin, S., Xu, L., Xu, C., Chen, X., Wang, A. C., Zhang, B., et al. (2019). Electron Transfer in Nanoscale Contact Electrification: Effect of Temperature in the Metal-Dielectric Case. Adv. Mat. 31 (17), 1808197. doi:10.1002/adma.201808197
Lin, Z.-H., Cheng, G., Lin, L., Lee, S., and Wang, Z. L. (2013). Water-solid Surface Contact Electrification and its Use for Harvesting Liquid-Wave Energy. Angew. Chem. Int. Ed. 52 (48), 12545–12549. doi:10.1002/anie.201307249
Liu, J., Wen, Z., Lei, H., Gao, Z., and Sun, X. (2022). A Liquid-Solid Interface-Based Triboelectric Tactile Sensor with Ultrahigh Sensitivity of 21.48 kPa−1. Nano-Micro Lett. 14 (1), 88. doi:10.1007/s40820-022-00831-7
Liufu, S., Xiao, H., and Li, Y. (2005). Adsorption of Poly(acrylic Acid) onto the Surface of Titanium Dioxide and the Colloidal Stability of Aqueous Suspension. J. Colloid Interface Sci. 281 (1), 155–163. doi:10.1016/j.jcis.2004.08.075
Lowell, J. (1977). Surface States and the Contact Electrification of Polymers. J. Phys. D. Appl. Phys. 10 (1), 65–71. doi:10.1088/0022-3727/10/1/008
Luo, J., and Wang, Z. L. (2020). Recent Progress of Triboelectric Nanogenerators: From Fundamental Theory to Practical Applications. EcoMat 2 (4). doi:10.1002/eom2.12059
Magnussen, O. M., and Gross, A. (2019). Toward an Atomic-Scale Understanding of Electrochemical Interface Structure and Dynamics. J. Am. Chem. Soc. 141 (12), 4777–4790. doi:10.1021/jacs.8b13188
Matsui, M., Murasaki, N., Fujibayashi, K., Peng You Bao, B., and Kishimoto, Y. (1993). Electrification of Pure Water Flowing Down a Trough Set up with a Resin Sheet. J. Electrost. 31 (1), 1–10. doi:10.1016/0304-3886(93)90043-7
McCarty, L. S., and Whitesides, G. M. (2008). Electrostatic Charging Due to Separation of Ions at Interfaces: Contact Electrification of Ionic Electrets. Angew. Chem. Int. Ed. 47 (12), 2188–2207. doi:10.1002/anie.200701812
Nie, J., Ren, Z., Xu, L., Lin, S., Zhan, F., Chen, X., et al. (2020). Probing Contact‐Electrification‐Induced Electron and Ion Transfers at a Liquid-Solid Interface. Adv. Mat. 32 (2), 1905696. doi:10.1002/adma.201905696
Nie, J., Wang, Z., Ren, Z., Li, S., Chen, X., and Lin Wang, Z. (2019). Power Generation from the Interaction of a Liquid Droplet and a Liquid Membrane. Nat. Commun. 10 (1), 2264. doi:10.1038/s41467-019-10232-x
Oja, S. M., Wood, M., and Zhang, B. (2012). Nanoscale Electrochemistry. Anal. Chem. 85 (2), 473–486. doi:10.1021/ac3031702
Qin, H., Xu, L., Lin, S., Zhan, F., Dong, K., Han, K., et al. (2022). Underwater Energy Harvesting and Sensing by Sweeping Out the Charges in an Electric Double Layer Using an Oil Droplet. Adv. Funct. Mater. 32, 2111662. doi:10.1002/adfm.202111662
Shi, Y., Lei, R., Li, F., Li, S., Tao, X., Yang, P., et al. (2021a). Self-powered Persistent Phosphorescence for Reliable Optical Display. ACS Energy Lett. 6 (9), 3132–3140. doi:10.1021/acsenergylett.1c01508
Shi, Y., Wang, F., Tian, J., Li, S., Fu, E., Nie, J., et al. (2021b). Self-powered Electro-Tactile System for Virtual Tactile Experiences. Sci. Adv. 7(6), eabe2943. doi:10.1126/sciadv.abe2943
Strasser, P., Gliech, M., Kuehl, S., and Moeller, T. (2018). Electrochemical Processes on Solid Shaped Nanoparticles with Defined Facets. Chem. Soc. Rev. 47 (3), 715–735. doi:10.1039/c7cs00759k
Sun, Z., Zhu, M., and Lee, C. (2021). Progress in the Triboelectric Human-Machine Interfaces (HMIs)-Moving from Smart Gloves to AI/Haptic Enabled HMI in the 5G/IoT Era. Nanoenergy Adv. 1 (1), 81–121. doi:10.3390/nanoenergyadv1010005
Tang, Z., Lin, S., and Wang, Z. L. (2021). Quantifying Contact‐Electrification Induced Charge Transfer on a Liquid Droplet after Contacting with a Liquid or Solid. Adv. Mater. 33 (42), 2102886. doi:10.1002/adma.202102886
Tao, F., and Crozier, P. A. (2016). Atomic-scale Observations of Catalyst Structures under Reaction Conditions and during Catalysis. Chem. Rev. 116 (6), 3487–3539. doi:10.1021/cr5002657
Tao, X., Li, S., Shi, Y., Wang, X., Tian, J., Liu, Z., et al. (2021a). Triboelectric Polymer with High Thermal Charge Stability for Harvesting Energy from 200 °C Flowing Air. Adv. Funct. Mat. 31 (49), 2106082. doi:10.1002/adfm.202106082
Tao, X., Nie, J., Li, S., Shi, Y., Lin, S., Chen, X., et al. (2021b). Effect of Photo-Excitation on Contact Electrification at Liquid-Solid Interface. ACS Nano 15 (6), 10609–10617. doi:10.1021/acsnano.1c03358
Tian, J., Chen, X., and Wang, Z. L. (2020). Environmental Energy Harvesting Based on Triboelectric Nanogenerators. Nanotechnology 31 (24), 242001. doi:10.1088/1361-6528/ab793e
Tian, J., Wang, F., Ding, Y., Lei, R., Shi, Y., Tao, X., et al. (2021). Self-powered Room-Temperature Ethanol Sensor Based on Brush-Shaped Triboelectric Nanogenerator. Research 2021, 1–11. doi:10.34133/2021/8564780
Wang, F., Ren, Z., Nie, J., Tian, J., Ding, Y., and Chen, X. (2019). Self‐Powered Sensor Based on Bionic Antennae Arrays and Triboelectric Nanogenerator for Identifying Noncontact Motions. Adv. Mat. Technol. 5 (1), 1900789. doi:10.1002/admt.201900789
Wang, F., Yang, P., Tao, X., Shi, Y., Li, S., Liu, Z., et al. (2021). Study of Contact Electrification at Liquid-Gas Interface. ACS Nano 15, 18206–18213. doi:10.1021/acsnano.1c07158
Wang, S., Lin, L., and Wang, Z. L. (2012). Nanoscale Triboelectric-Effect-Enabled Energy Conversion for Sustainably Powering Portable Electronics. Nano Lett. 12 (12), 6339–6346. doi:10.1021/nl303573d
Wang, S., Lin, L., Xie, Y., Jing, Q., Niu, S., and Wang, Z. L. (2013). Sliding-triboelectric Nanogenerators Based on In-Plane Charge-Separation Mechanism. Nano Lett. 13 (5), 2226–2233. doi:10.1021/nl400738p
Wang, X., Shi, Y., Yang, P., Tao, X., Li, S., Lei, R., et al. (2022a). Fish‐Wearable Data Snooping Platform for Underwater Energy Harvesting and Fish Behavior Monitoring. Small 18 (10), 2107232. doi:10.1002/smll.202107232
Wang, Z., Berbille, A., Feng, Y., Li, S., Zhu, L., Tang, W., et al. (2022b). Contact-electro-catalysis for the Degradation of Organic Pollutants Using Pristine Dielectric Powders. Nat. Commun. 13 (1), 130. doi:10.1038/s41467-021-27789-1
Wang, Z. L., Jiang, T., and Xu, L. (2017). Toward the Blue Energy Dream by Triboelectric Nanogenerator Networks. Nano Energy 39, 9–23. doi:10.1016/j.nanoen.2017.06.035
Wang, Z. L. (2017). On Maxwell's Displacement Current for Energy and Sensors: the Origin of Nanogenerators. Mater. Today 20 (2), 74–82. doi:10.1016/j.mattod.2016.12.001
Wang, Z. L. (2020). On the First Principle Theory of Nanogenerators from Maxwell's Equations. Nano Energy 68, 104272. doi:10.1016/j.nanoen.2019.104272
Wiles, J. A., Fialkowski, M., Radowski, M. R., Whitesides, G. M., and Grzybowski, B. A. (2004). Effects of Surface Modification and Moisture on the Rates of Charge Transfer between Metals and Organic Materials. J. Phys. Chem. B 108 (52), 20296–20302. doi:10.1021/jp0457904
Xie, L., Zhai, N., Liu, Y., Wen, Z., and Sun, X. (2021a). Hybrid Triboelectric Nanogenerators: From Energy Complementation to Integration. Research 2021, 1–23. doi:10.34133/2021/9143762
Xie, X., Chen, X., Zhao, C., Liu, Y., Sun, X., Zhao, C., et al. (2021b). Intermediate Layer for Enhanced Triboelectric Nanogenerator. Nano Energy 79, 105439. doi:10.1016/j.nanoen.2020.105439
Xiong, J., Thangavel, G., Wang, J., Zhou, X., and Lee, P. S. (2020). Self-healable Sticky Porous Elastomer for Gas-Solid Interacted Power Generation. Sci. Adv. 6(29), eabb4246. doi:10.1126/sciadv.abb4246
Xu, C., Zi, Y., Wang, A. C., Zou, H., Dai, Y., He, X., et al. (2018). On the Electron-Transfer Mechanism in the Contact-Electrification Effect. Adv. Mat. 30 (15), 1706790. doi:10.1002/adma.201706790
Xu, M., Wang, S., Zhang, S. L., Ding, W., Kien, P. T., Wang, C., et al. (2019). A Highly-Sensitive Wave Sensor Based on Liquid-Solid Interfacing Triboelectric Nanogenerator for Smart Marine Equipment. Nano Energy 57, 574–580. doi:10.1016/j.nanoen.2018.12.041
Xu, W., Zheng, H., Liu, Y., Zhou, X., Zhang, C., Song, Y., et al. (2020). A Droplet-Based Electricity Generator with High Instantaneous Power Density. Nature 578 (7795), 392–396. doi:10.1038/s41586-020-1985-6
Xue, G., Xu, Y., Ding, T., Li, J., Yin, J., Fei, W., et al. (2017). Water-evaporation-induced Electricity with Nanostructured Carbon Materials. Nat. Nanotech 12 (4), 317–321. doi:10.1038/nnano.2016.300
Yang, P., Shi, Y., Li, S., Tao, X., Liu, Z., Wang, X., et al. (2022). Monitoring the Degree of Comfort of Shoes In-Motion Using Triboelectric Pressure Sensors with an Ultrawide Detection Range. ACS Nano 16 (3), 4654–4665. doi:10.1021/acsnano.1c11321
Yang, Y., Zhang, H., Liu, Y., Lin, Z.-H., Lee, S., Lin, Z., et al. (2013a). Silicon-based Hybrid Energy Cell for Self-Powered Electrodegradation and Personal Electronics. Acs Nano 7 (3), 2808–2813. doi:10.1021/nn400361p
Yang, Y., Zhou, Y. S., Zhang, H., Liu, Y., Lee, S., and Wang, Z. L. (2013b). A Single-Electrode Based Triboelectric Nanogenerator as Self-Powered Tracking System. Adv. Mat. 25 (45), 6594–6601. doi:10.1002/adma.201302453
Yatsuzuka, K., Higashiyama, Y., and Asano, K. (1996). Electrification of Polymer Surface Caused by Sliding Ultrapure Water. IEEE Trans. Ind. Appl. 32 (4), 825–831. doi:10.1109/28.511638
Yatsuzuka, K., Mizuno, Y., and Asano, K. (1994). Electrification Phenomena of Pure Water Droplets Dripping and Sliding on a Polymer Surface. J. Electrost. 32 (2), 157–171. doi:10.1016/0304-3886(94)90005-1
Zhan, F., Wang, A. C., Xu, L., Lin, S., Shao, J., Chen, X., et al. (2020). Electron Transfer as a Liquid Droplet Contacting a Polymer Surface. ACS Nano 14, 17565–17573. doi:10.1021/acsnano.0c08332
Zhang, Q., Li, Y., Cai, H., Yao, M., Zhang, H., Guo, L., et al. (2021). A Single‐Droplet Electricity Generator Achieves an Ultrahigh Output over 100 V without Pre‐Charging. Adv. Mater. 33 (51), 2105761. doi:10.1002/adma.202105761
Zhang, T., Wen, Z., Liu, Y., Zhang, Z., Xie, Y., and Sun, X. (2020). Hybridized Nanogenerators for Multifunctional Self-Powered Sensing: Principles, Prototypes, and Perspectives. iScience 23 (12), 101813. doi:10.1016/j.isci.2020.101813
Zhang, Y., Pähtz, T., Liu, Y., Wang, X., Zhang, R., Shen, Y., et al. (2015). Electric Field and Humidity Trigger Contact Electrification. Phys. Rev. X 5 (1), 011002. doi:10.1103/PhysRevX.5.011002
Zhao, X., Lu, X., Zheng, Q., Fang, L., Zheng, L., Chen, X., et al. (2021). Studying of Contact Electrification and Electron Transfer at Liquid-Liquid Interface. Nano Energy 87, 106191. doi:10.1016/j.nanoen.2021.106191
Zheng, M., Lin, S., Zhu, L., Tang, Z., and Wang, Z. L. (2021). Effects of Temperature on the Tribovoltaic Effect at Liquid‐Solid Interfaces. Adv. Mater. Inter 9 (3), 2101757. doi:10.1002/admi.202101757
Zhong, W., Xu, L., Zhan, F., Wang, H., Wang, F., and Wang, Z. L. (2020). Dripping Channel Based Liquid Triboelectric Nanogenerators for Energy Harvesting and Sensing. ACS Nano 14 (8), 10510–10517. doi:10.1021/acsnano.0c04413
Zhong, Y., Zhao, H., Guo, Y., Rui, P., Shi, S., Zhang, W., et al. (2019). An Easily Assembled Electromagnetic‐Triboelectric Hybrid Nanogenerator Driven by Magnetic Coupling for Fluid Energy Harvesting and Self‐Powered Flow Monitoring in a Smart Home/City. Adv. Mat. Technol. 4 (12), 1900741. doi:10.1002/admt.201900741
Zhu, G., Lin, Z.-H., Jing, Q., Bai, P., Pan, C., Yang, Y., et al. (2013). Toward Large-Scale Energy Harvesting by a Nanoparticle-Enhanced Triboelectric Nanogenerator. Nano Lett. 13 (2), 847–853. doi:10.1021/nl4001053
Keywords: triboelectric nanogenerator, charge transfer, contact electrification, liquid–solid interfaces, liquid–liquid interfaces, gas and other interfaces
Citation: Zhang W, Shi Y, Li Y, Chen X and Shen H (2022) A Review: Contact Electrification on Special Interfaces. Front. Mater. 9:909746. doi: 10.3389/fmats.2022.909746
Received: 31 March 2022; Accepted: 09 May 2022;
Published: 23 June 2022.
Edited by:
Sihong Wang, The University of Chicago, United StatesReviewed by:
Minyi Xu, Dalian Maritime University, ChinaXuhui Sun, Soochow University, China
Xiaoyi Li, Ocean University of China, China
Copyright © 2022 Zhang, Shi, Li, Chen and Shen. This is an open-access article distributed under the terms of the Creative Commons Attribution License (CC BY). The use, distribution or reproduction in other forums is permitted, provided the original author(s) and the copyright owner(s) are credited and that the original publication in this journal is cited, in accordance with accepted academic practice. No use, distribution or reproduction is permitted which does not comply with these terms.
*Correspondence: Yufang Li, bHlmX21zY0BudWFhLmVkdS5jbg==; Xiangyu Chen, Y2hlbnhpYW5neXVAYmlubi5jYXMuY24=