Heterogeneous Interfaces of Magnetic CIP and Binary Dielectric Carbon-Based Material Toward High-Efficiency Microwave Attenuation
- 1304 Department, Xi’an Research Institute of High-Tech, Xi’an, China
- 2College of Weapon Science and Technology, Xi’an Technological University, Xi’an, China
Carbon-based materials, as lightweight absorbers, have been widely applied in varied fields to counteract the adverse effects of electromagnetic waves. Nevertheless, there remain challenges in coping with the impairment of microwave absorption performance caused by the excessively high permittivity of carbon materials. Herein, carbonyl iron powder (CIP) was added to graphene oxide/carbon black (RGO-CB) composites to adjust the permittivity improving impedance matching and electromagnetic absorption properties. In this work, the RGO provides a three-dimensional structure net, which introduces circularly and variously electromagnetic loss interfaces within the RGO-CB/CIP composites. Owing to the unique architecture and synergy of the three components, the as-prepared absorber exhibits good impedance matching and gratifying microwave absorption properties. It was found that the 1:1 mixture of RGO-CB and CIP in the composite had superb impedance matching at the thicknesses of 2.6 and 2.7 mm to achieve complete X-band microwave absorption. Such excellent performance benefits from the multiple polarization provided by the heterogeneous interfaces and the ideal impedance match attributed to the tunable permeability and permittivity of this work.
Introduction
Up to now, the range of X-band of electromagnetic waves is still the most critical frequency of electromagnetic waves in civil and military aerospace and space technology (Micheli et al., 2010; Vinayasree et al., 2013; Silva and Rezende, 2021). Though with increasing convenience to human life, the ensuing electromagnetic pollution can greatly impact human health and sophisticated electronic devices. Thus, microwave absorbing materials have drawn the attention of researchers worldwide. A microwave absorbing material with lightweight, strong absorption, small thickness, and wide absorption bandwidth is considered ideal for attenuating electromagnetic energies (He et al., 2020; Liang et al., 2020; Wang et al., 2020).
As one of the essential absorbing properties, impedance matching determines whether electromagnetic waves can enter from free space into the absorber, which is an essential factor that must be considered when designing an absorber. The current single absorbing materials, including magnetic and electric loss absorbing material, can not achieve good impedance matching conditions due to the large gap between complex permittivity and permeability (Li et al., 2018). Apart from that, the attenuation constant is another factor that must be considered for designing a microwave absorbing material. When electromagnetic waves pass through the surface of the absorber and enter the interior, it is vital that incident waves can be attenuated rapidly to achieve effective absorption (Li et al., 2022). The perfect combination of attenuation constants and impedance matching will be a top priority for scholars designing ideal absorbers (Shen et al., 2021).
Typical nanostructured carbon, including graphite, CB and carbon nanotubes (CNTs) materials, are usually applied as microwave absorber given their excellent dielectric properties, low density and other unique physical and chemical properties (Li et al., 2018). In recent years, graphene, as a new type of nanostructured carbon material, has become a focus of attention for its excellent electrical, thermal, mechanical properties and high specific surface area (Song et al., 2021). Pure graphene absorbers mainly rely on conduction losses to attenuate electromagnetic wave energies (Zhang et al., 2019). However, good electrical conductivity hampers impedance matching, leading to the inability of electromagnetic waves to cross the surface of the absorber. This means that the best way to take advantage of graphene’s superb dielectric loss is to improve impedance matching. As another member of the carbon family, carbon black possesses both excellent dielectric properties and low density. One of the benefits of using carbon black as a filler between graphene sheets is that it preserves graphene’s dielectric properties while also reducing its conductivity, thus improving the wave absorption properties of graphene.
As of now, the most widely studied magnetic microwave-absorbing materials include Fe3O4, cobalt-zinc ferrite, magnetite nanoparticles, Ni, and carbonyl iron powders (CIP) (He et al., 2017; Li et al., 2017; Liao et al., 2018; Xu et al., 2018; Wang et al., 2019; Wang et al., 2020; Reyes-Ortega et al., 2021; Wang et al., 2021). Carbonyl iron powders (CIP) are commonly used in composites because of their high saturation magnetization strength, low coercivity, and good magnetic properties (Sista et al., 2021). However, the high density and filling ratio of CIP prevent it from being widely used. In general, it is hard to achieve the desired electromagnetic wave absorption properties solely by filling absorbers with dielectric or magnetic media. Thus, researchers attempt to combine magnetic and carbon materials in composite or use hybridized approaches to improve the impedance matching as well as its microwave loss performance (Yang et al., 2020; Han et al., 2021; Jang et al., 2021). Recently, Liu et al. (Liu et al., 2010) investigated the microwave absorption performance of monolayer coatings consisting of CIP and CB in the range of 2–18 GHz. They found that at a sample thickness of 1.5 mm, the absorption bandwidth broadened with increasing CB content, especially when the ratio of CIP and CB was 25 wt.% respectively, and the absorption bandwidth (RL < −4 dB) reached 10.1 GHz (7.9–18 GHz). Lopes et al. (Lopes et al., 2020) prepared hybrid composites with 30 wt.% MnZn ferrites, 50 wt.% CIP and 1 wt.% CNTs and investigated their electromagnetic absorption properties on silicone rubber in the X-band (8.2–12.4 GHz). The RLmax was found at 2.3 mm thickness, with a value of −21 dB. Xu et al. (Xu et al., 2015)evaluated the microwave absorption performance of the graphene doped with CIP and found that the CIP/GO composites filled with 5 vol% had excellent wave absorption performance at 2–18 GHz, with the RLmax of - 25.14 dB at 6 mm and -26.52 dB at 8 mm. The above studies showed that many scholars have tried to combine the properties of CIP and carbon-based materials to prepare an ideal microwave absorbing material and have indeed achieved some progress. However, there still exist deficiencies such as the lack of absorbing bandwidth, the low absorbing intensity, and the excessive thickness.
In order to overcome these deficiencies, the present RGO-CB/CIP composite was synthesized via a three-step method. GO was prepared based on the Hummers method in the first step and followed by the one-pot method to attach the CB with RGO during the reduction of GO to GRO. Finally, the group on RGO-CB was activated by acidification treatment while roughening the CIP surface to facilitate the binding of CIP to RGO-CB. This work concentrates on the electromagnetic and microwave absorption properties of RGO-CB/CIP composites in 8–12.4 GHz. The effects of CIP loading and absorber thickness on the microwave absorption properties of the composites were discussed, and the microwave absorption mechanism was analyzed in depth.
Experiments
Preparation of Aqueous GO Solutions
Based on the modified Hummers method, graphene oxide solutions were prepared. Graphite powder (6 g) and NaNO3 were added to concentrated H2SO4 (360 ml) stirred under an ice water bath for 1 h, and then 18 g of KMnO4 powder was added slowly. A slow addition rate and a temperature of lower than 5°C were stressed to the safety of this method. After 2 h of stirring, the mixture turned to dark green-black colour. The solution was then heated to 30°C and stirred for another 2 h. Deionized water (600 ml) was added to the solution and stirred for 0.5 h H2O2 (150 ml 5%) was then added and stirred for 0.5 h. After 12 h of settling, the solution was poured off its top layer, and the precipitate was washed with hydrochloric acid solution and deionized water. After six times centrifugation and washed in total, the pure precipitate was obtained. In the end, 500 ml GO solution (GOs) was prepared by dissolving the precipitate in deionized water and stirring. The schematic is shown in Figure 1.
Preparation of RGO-CB Composites
RGO-CB was prepared by the one-pot method. Firstly, 500 ml of deionized water was mixed with 2.4 g of CB powder and 1.2 g of CTAB (hexadecyl trimethyl ammonium bromide), and the mixture was stirred for 15 min before being sonicated for 1 h. At the same time, 100 ml of GOs was taken and sonicated for 1 h. Once the CB dispersion solution was sonicated, GOs was added and sonicated for an additional hour. After the correct amount of p-diphenol was added, the beaker was sealed with cling film. The obtained mixed solution was transferred to a drying oven and heated at 100°C for 12 h, and then the precipitate was washed with deionized water and cycled twice. After vacuum freeze-drying treatment, CB/RGO composites were obtained.
Preparation of RGO-CB/CIP Composites
RGO-CB/CIP composite was synthesized by a simple acidification process. The CB/RGO solution was generated by dispersing 1.2 g of CB/RGO powder in 100 ml of deionized water and sonicating for 2 h. To get a well-mixed solution, 200 ml of HCl (35%) was added to the CB/RGO solution and constantly stirred at room temperature. An appropriate amount of CIP was then added to 100 ml of ethanol and stirred vigorously to form a homogeneous dispersion before being added to the CB/RGO solution at room temperature. To flocculate, the mixture was sonicated for 1 h and then held. Finally, the flocs were removed by filtration, washed several times with deionized water and ethanol, and dried in a vacuum oven at 70°C for 12 h. Four sample materials were made according to the proportion of CIP and CB/RGO (1:2, 1:1, 2:1, 3:1, S1, S2, S3 and S4, respectively).
Characterization
These composites were characterized using a variety of methods. The microstructure and morphology of the samples were observed by a ZEISS GeminiSEM 300 scanning electron microscope (SEM) and an F200X transmission electron microscope (TEM) from Germany. Coaxial line measurements of electromagnetic parameters were performed with a KEYSIGHT E5071C vector network analyzer. The specimens were prepared by homogeneously mixing paraffin wax with the sample at a mass ratio of 3:7 and then pressing the mixture into an annular ring with an external diameter of 7.00 mm and an internal diameter of 3.04 mm.
Results and Discussion
RGO-CB/CIP composite’s XRD pattern was depicted in Figure 2. It showed the presence of four phases of carbon in the RGO-CB/CIP composite by comparing the test data with the PDF#89-8487 standard card, with 2θ values equal to 26.55°, 42.37°, 50.71°, and 59.91°, which can be attributed to (002), (100), (102) and (103) crystal planes of RGO or CB, respectively. Compared with the standard card PDF#72-1081, there were majorly four CIP crystal planes in the RGO-CB/CIP composite, (−111), (002), (−112) and (130), corresponding to the 2θ values of 16.88°, 20.06°, 23.26°, and 40.57°, respectively. The characteristic diffraction peaks show that the CIP successfully grew in the composite.
Figure 3 shows the RGO-CB/CIP composite’s SEM image. Obviously, there are abundant crumples on the surface of RGO, and multiple heterogeneous interfaces are formed through the contact between individual folds or the connection of CB and CIP, which would lead to multiple interface polarization. Meanwhile, CB, CIP and RGO were superimposed in sequence, forming a multi-stage structure. The interface polarization and multiple scattering characteristics of the multi-stage structure might effectively improve the absorbing properties (Weng et al., 2022). It was evident that the nanospheres were tightly adhered to the RGO surface, especially between the individual folds. Therefore, the two-dimensional graphene nanosheets are developed into three-dimensional structural composite materials.
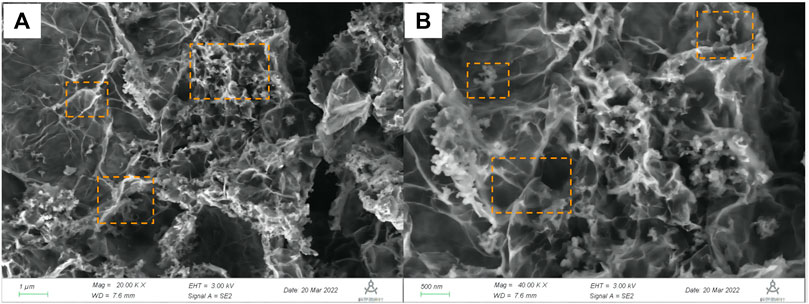
FIGURE 3. SEM image of the RGO-CB/CIP composite. (A) The interface formed by the folding of RGO itself; (B) CIP and CB are attached to the RGO surface to form a heterogeneous interface.
Figure 4 shows the morphology of the RGO-CB/CIP composite taken by the transmission electron microscope. From Figures 4A,B, it can be intuitively observed that the soft RGO film is like a giant net covering CB and CIP, forming a cladding structure. This structure would easily lead to the confinement effect (Hao et al., 2016) and could significantly improve the electromagnetic wave attenuation capability of the RGO-CB/CIP composite. By performing a high-resolution TEM (Figure 4D) of the interface shown in Figure 4C, it can be confirmed that CIP and CB were tangled with each other by the lattice fringe spacing of ∼0.52 and ∼0.33 nm corresponding to the d-spacing of CIP(−111) and carbon (002), respectively. It provides visual structural evidence for the existence of interface polarization to attenuate microwaves in the composite.
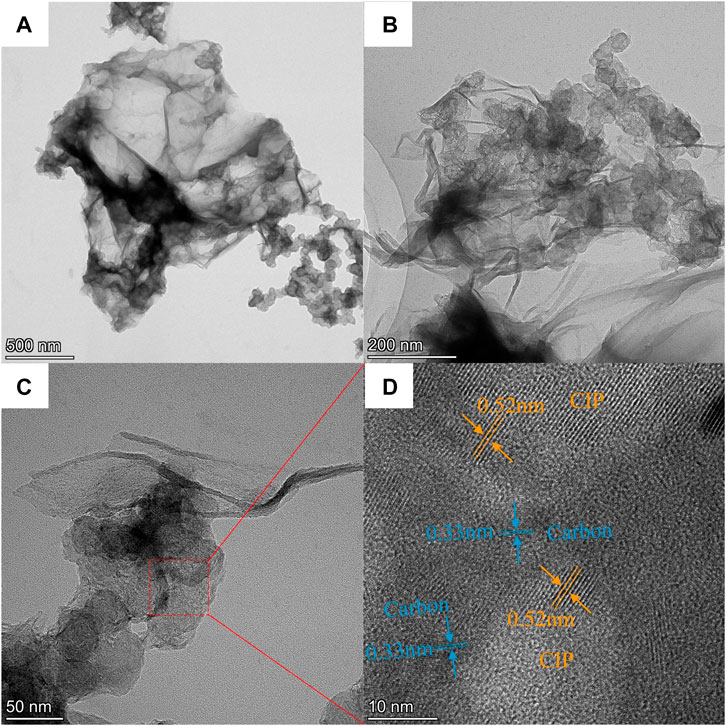
FIGURE 4. Transmission electron micrograph of RGO-CB/CIP composite. (A,B) CB and CIP particles wrapped with RGO film; (C) interface between CB and CIP; (D) high resolution TEM image of the selected part of (C), showing lattice fringe spacing of CIP and CB.
The as-prepared samples were applied to the test of magnetic properties at room temperature and the magnetic field was 6,000 Oe. The hysteresis loops of the samples are shown in Figure 5. It is evident that the growth of CB has limited influence on the magnetic properties of RGO, while the saturation magnetization of the RGO-CB/CIP is much higher than that of RGO and RGO-CB samples since the CIP is a typical magnetic material, which shows that the CIP is successfully growing with the RGO-CB.
RL (reflection loss) was calculated from the relative complex permittivity
Where Zin is the normalized input impedance, and d is the thickness of the material. Effective microwave absorption is defined as less than 10% reflectance, i.e., RL < −10 dB, and the corresponding frequency range is the effective absorption bandwidth (EAB). Absorption and reflection losses are shown in Figure 6 for the various thicknesses of samples, and all samples have good microwave absorption in X-band. Based on transmission line theory and calculated S1’s electromagnetic parameters, it is found that the EAB of S1 is equal to 4.2 GHz, which covers the X-band (8.2–12.4 GHz) with a maximum of RLmax of −16.88 dB at 2.6 mm (Figure 6A). The RLmax of −18.59 dB and −17.28 dB are achieved by S2 at 2.6 and 2.7 mm thicknesses, respectively, with full EAB coverage of the X-band (Figure 6B). RLmax of −19.78 dB and -20.92 dB for S3 and S4 were achieved (Figures 6C,D). Meanwhile, the EAB of both S3 and S4 covered the X-band at 2.7 mm. Aside from this, it is clear from the graph that the increase of CIP content is positively associated with the maximum intensity of RLmax for the four samples in the 2.0–3.0 mm range, with RLmax values of −19.00 dB, −21.14 dB, −22.59 dB, and −29.17 dB, corresponding the thickness of 2.4 mm (S1), 2.4 mm (S2), 2.4 mm (S3) and 2.5 mm (S4,) respectively.
The absorption properties of electromagnetic waves are closely related to the complex permittivity and complex permeability. In general, the real part of the complex permittivity
Based on the conductive network manner proposed by Cao and his coworker, the electron hopping between CB-RGO layers significantly affects the conductivity of the composite (Wang et al., 2018). When introduced, CIP exhibits resistive properties that hinder inter-layer electron hopping and intra-layer electron migration, reducing conductive network connections and lowering the conductivity of the composites.
According to the Debye theory, the
Based on the two equations above, the relationship between
In the above equations,
As can be seen in Figure 8A, the mean values of
Figure 9 shows the dielectric loss tangent and the magnetic loss tangent of the RGO-CB/CIP composite from 8 to 12.4 GHz. The dielectric loss tangent is much larger than the magnetic loss tangent, indicating that the dielectric loss is dominant among the two loss manners. As CIP is a kind of magnetically lossy absorbing material, the average value of the magnetic loss tangent increases gradually as the CIP content. However, something is unexpectable that the content of CIP increasing makes the dielectric loss tangent of S2 larger than that of S1. These results suggested that adding an appropriate amount of CIP could increase the number of heterogeneous interfaces in the composite, enhancing the interfacial polarization of the composite and improving its dielectric loss strength. However, with the continuing increase of CIP, the dielectric loss tangent value of S3 decreases abruptly and reaches its lowest value at S4. This result also corroborates the previous finding of the decrease in S3 and S4 Debay rings. In short, the excessive addition of CIP weakens the connection points between graphene layers, and widens their distance, leading to the difficulty of electron hopping between the layers, thus hindering the interfacial polarization. As the CIP is in a resistive state on graphene, excessive concentration also inevitably leads to a less efficient conductive network and makes it difficult for conductive losses to complete for electromagnetic waves attenuation.
Where Re [X] is taken as the real part of the complex number X,
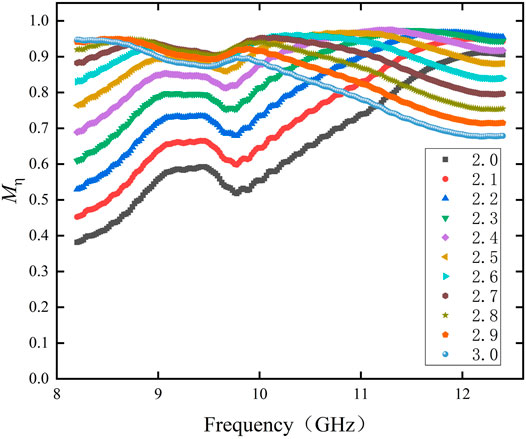
FIGURE 10. Impedance matching coefficient graph of samples S2 for 2.0–3.0 mm. The graph shows the wave impedance matching factor
RGO-CB/CIP composite absorption of electromagnetic waves was summarized in Figure 11. Impedance matching was critical to ensure the vast majority of electromagnetic waves penetrate the composite’s interior through the air. The energy of the incident electromagnetic waves was then converted into heat dissipation inside the absorber by various absorption mechanisms, as elaborated below.
1 Conductive losses. In the composite material, graphene, carbon black and CIP formed a three-dimension conductive network of limited resistance. When electromagnetic waves passed through the composite material, induced currents were formed within the material and converted to thermal energy as the induced currents passed through the resistive conductive network.
2 Polarization loss. As a ternary composite, the RGO-CB/CIP composite had multiple forms of phase boundaries. The different phase boundaries in RGO-CB/CIP can accumulate charge and lead to interfacial polarization. Also, in reduced graphene oxide, there is a large number of functional groups and defects, which can act as dipole centres under the influence of a magnetic field and cause dipole polarization.
3 Magnetic losses. Due to the presence of CIP in the RGO-CB/CIP complex, the RGO-CB/CIP complex is subjected to not only dielectric losses but also magnetic losses. The leading cause of magnetic loss was natural resonance.
Conclusion
In summary, RGO-CB/CIP composite was prepared by hummers method, one-pot method, and acidified mixing method. GO was grafted with CB during the reduction process of GO to RGO, forming an RGO-CB composite, which would be tightly CIP-coated. By combining the magnetic loss material with the dielectric loss material, the RGO-CB/CIP composite achieved better impedance matching performance. Most of the electromagnetic waves of the X-band can enter the material. The redox process of graphene led to the formation of many defects on the surface of graphene, which became active centres for dipole polarization. In addition, the heterogeneous interface formed between RGO, CB, and CIP provided conditions for the accumulation of charges, and the multi-heterogeneous interface dramatically enhanced the interface polarization. The results demonstrated that the sample, whose proportion of CIP and RGO-CB is 1:1, had an absorption bandwidth covering the X-band at 2.6 and 2.7 mm, and had microwave absorption peaks at −18.59 dB and -17.28 dB, respectively. This research provided a method for making X-band-targeted absorbing materials, as well as an in-depth analysis of the absorption mechanism, in the hopes of serving as a guide for future researchers.
Data Availability Statement
The raw data supporting the conclusion of this article will be made available by the authors, without undue reservation.
Author Contributions
The thesis was completed by YS under the supervision of SB and GH for the experiments and thesis writing. The protocol design of the experiments was done by HL, and ZL was responsible for the theoretical study and image processing.
Funding
This work was supported by the National Natural Science Foundation of China (No. 62175010).
Conflict of Interest
The authors declare that the research was conducted in the absence of any commercial or financial relationships that could be construed as a potential conflict of interest.
Publisher’s Note
All claims expressed in this article are solely those of the authors and do not necessarily represent those of their affiliated organizations, or those of the publisher, the editors and the reviewers. Any product that may be evaluated in this article, or claim that may be made by its manufacturer, is not guaranteed or endorsed by the publisher.
References
Deng, B., Xiang, Z., Xiong, J., Liu, Z., Yu, L., and Lu, W. (2020). Sandwich-like Fe&TiO2@C Nanocomposites Derived from MXene/Fe-MOFs Hybrids for Electromagnetic Absorption. Nano-Micro Lett. 12, 1–16. doi:10.1007/s40820-020-0398-2
Han, C., Zhang, M., Cao, W.-Q., and Cao, M.-S. (2021). Electrospinning and In-Situ Hierarchical Thermal Treatment to Tailor C-NiCo2O4 Nanofibers for Tunable Microwave Absorption. Carbon 171, 953–962. doi:10.1016/j.carbon.2020.09.067
Hao, X.-Q., Yang, H., Jin, Z.-L., Xu, J., Min, S.-X., and Lü, G.-X. (2016). Quantum Confinement Effect of Graphene-like C3N4 Nanosheets for Efficient Photocatalytic Hydrogen Production fromWater Splitting. Acta Physico-Chimica Sin. 32, 2581–2592. doi:10.3866/PKU.WHXB201606226
He, G., Duan, Y., and Pang, H. (2020). Microwave Absorption of Crystalline Fe/MnO@C Nanocapsules Embedded in Amorphous Carbon. Nano-Micro Lett. 12, 1–16. doi:10.1007/s40820-020-0388-4
He, P., Hou, Z.-L., Zhang, K.-L., Li, J., Yin, K., Feng, S., et al. (2017). Lightweight Ferroferric Oxide Nanotubes with Natural Resonance Property and Design for Broadband Microwave Absorption. J. Mater Sci. 52, 8258–8267. doi:10.1007/s10853-017-1041-6
Jang, W., Mallesh, S., Gu, M., and Kim, K. H. (2021). Edge Selectively Oxidized Graphene on Carbonyl Iron Composites for Microwave Absorption and Radar Cross-Section Performance at X- and Ku-Band. J. Alloys Compd. 886, 161230. doi:10.1016/j.jallcom.2021.161230
Li, J., Bi, S., Mei, B., Shi, F., Cheng, W., Su, X., et al. (2017). Effects of Three-Dimensional Reduced Graphene Oxide Coupled with Nickel Nanoparticles on the Microwave Absorption of Carbon Fiber-Based Composites. J. Alloys Compd. 717, 205–213. doi:10.1016/j.jallcom.2017.03.098
Li, Y., Sun, N., Liu, J., Hao, X., Du, J., Yang, H., et al. (2018). Multifunctional BiFeO3 Composites: Absorption Attenuation Dominated Effective Electromagnetic Interference Shielding and Electromagnetic Absorption Induced by Multiple Dielectric and Magnetic Relaxations. Compos. Sci. Technol. 159, 240–250. doi:10.1016/j.compscitech.2018.02.014
Li, Y., Wang, G., Gong, A., Zhang, S., Liu, J., Sun, N., et al. (2022). High‐Performance Ferroelectric Electromagnetic Attenuation Materials with Multiple Polar Units Based on Nanodomain Engineering. Small (Weinheim der Bergstrasse, Ger. 18, e2106302–n/a. doi:10.1002/smll.202106302
Liang, X., Man, Z., Quan, B., Zheng, J., Gu, W., Zhang, Z., et al. (2020). Environment-Stable CoxNiy Encapsulation in Stacked Porous Carbon Nanosheets for Enhanced Microwave Absorption. Nano-Micro Lett. 12, 1–12. doi:10.1007/s40820-020-00432-2
Liao, Q., He, M., Zhou, Y., Nie, S., Wang, Y., Hu, S., et al. (2018). Highly Cuboid-Shaped Heterobimetallic Metal-Organic Frameworks Derived from Porous Co/ZnO/C Microrods with Improved Electromagnetic Wave Absorption Capabilities. ACS Appl. Mat. Interfaces 10, 29136–29144. doi:10.1021/acsami.8b09093
Liu, L., Duan, Y., Ma, L., Liu, S., and Yu, Z. (2010). Microwave Absorption Properties of a Wave-Absorbing Coating Employing Carbonyl-Iron Powder and Carbon Black. Appl. Surf. Sci. 257, 842–846. doi:10.1016/j.apsusc.2010.07.078
Lopes, B. H. K., Portes, R. C., Amaral Junior, M. A. D., Florez-Vergara, D. E., Gama, A. M., Silva, V. A., et al. (2020). X Band Electromagnetic Property Influence of Multi-Walled Carbon Nanotube in Hybrid MnZn Ferrite and Carbonyl Iron Composites. J. Mater. Res. Technol. 9, 2369–2375. doi:10.1016/j.jmrt.2019.12.068
Micheli, D., Apollo, C., Pastore, R., and Marchetti, M. (2010). X-band Microwave Characterization of Carbon-Based Nanocomposite Material, Absorption Capability Comparison and RAS Design Simulation. Compos. Sci. Technol. 70, 400–409. doi:10.1016/j.compscitech.2009.11.015
Reyes-Ortega, F., Delgado, Á., and Iglesias, G. (2021). Modulation of the Magnetic Hyperthermia Response Using Different Superparamagnetic Iron Oxide Nanoparticle Morphologies. Nanomaterials 11, 627. doi:10.3390/nano11030627
Shen, J., Zhang, D., Han, C., Wang, Y., Zeng, G., and Zhang, H. (2021). Three-dimensional Flower-like FeCoNi/reduced Graphene Oxide Nanosheets with Enhanced Impedance Matching for High-Performance Electromagnetic Wave Absorption. J. Alloys Compd. 883, 160877. doi:10.1016/j.jallcom.2021.160877
Silva, V. A., and Rezende, M. C. (2021). S‐parameters, Electrical Permittivity, and Absorbing Energy Measurements of Carbon Nanotubes‐based Composites in X‐band. J. Appl. Polym. Sci. 138, 49843–n/a. doi:10.1002/app.49843
Sista, K. S., Dwarapudi, S., Kumar, D., Sinha, G. R., and Moon, A. P. (2021). Carbonyl Iron Powders as Absorption Material for Microwave Interference Shielding: A Review. J. Alloys Compd. 853, 157251. doi:10.1016/j.jallcom.2020.157251
Song, P., Liu, B., Liang, C., Ruan, K., Qiu, H., Ma, Z., et al. (2021). Lightweight, Flexible Cellulose-Derived Carbon Aerogel@Reduced Graphene Oxide/PDMS Composites with Outstanding EMI Shielding Performances and Excellent Thermal Conductivities. Nano-Micro Lett. 13, 1–17. doi:10.1007/s40820-021-00624-4
Vinayasree, S., Soloman, M., Sunny, V., Mohanan, P., Kurian, P., and Anantharaman, M. (2013). A Microwave Absorber Based on Strontium Ferrite-Carbon Black-Nitrile Rubber for S and X-Band Applications. Compos. Sci. Technol. 82, 69–75. doi:10.1016/j.compscitech.2013.04.010
Wang, D.-J., Zhang, J.-Y., He, P., and Hou, Z.-L. (2019). Size-modulated Electromagnetic Properties and Highly Efficient Microwave Absorption of Magnetic Iron Oxide Ceramic Opened-Hollow Microspheres. Ceram. Int. 45, 23043–23049. doi:10.1016/j.ceramint.2019.07.352
Wang, J., Jia, Z., Liu, X., Dou, J., Xu, B., Wang, B., et al. (2021). Construction of 1D Heterostructure NiCo@C/ZnO Nanorod with Enhanced Microwave Absorption. Nano-Micro Lett. 13, 1–16. doi:10.1007/s40820-021-00704-5
Wang, L., Yu, X., Li, X., Zhang, J., Wang, M., and Che, R. (2020). MOF-derived Yolk-Shell Ni@C@ZnO Schottky Contact Structure for Enhanced Microwave Absorption. Chem. Eng. J. 383, 123099. doi:10.1016/j.cej.2019.123099
Wang, X.-X., Ma, T., Shu, J.-C., and Cao, M.-S. (2018). Confinedly Tailoring Fe3O4 Clusters-NG to Tune Electromagnetic Parameters and Microwave Absorption with Broadened Bandwidth. Chem. Eng. J. 332, 321–330. doi:10.1016/j.cej.2017.09.101
Weng, L., Lei, X., Liu, J., Hu, H., Wang, J., and Zhang, Z. (2022). Three-dimensional Network FeNi/C Composites with Excellent Microwave-Absorbing Properties. J. Alloys Compd. 906, 164301. doi:10.1016/j.jallcom.2022.164301
Xu, W., Pan, Y.-F., Wei, W., Wang, G.-S., and Qu, P. (2018). Microwave Absorption Enhancement and Dual-Nonlinear Magnetic Resonance of Ultra Small Nickel with Quasi-One-Dimensional Nanostructure. Appl. Surf. Sci. 428, 54–60. doi:10.1016/j.apsusc.2017.09.052
Xu, Y., Yan, Z., and Zhang, D. (2015). Microwave Absorbing Property of a Hybrid Absorbent with Carbonyl Irons Coating on the Graphite. Appl. Surf. Sci. 356, 1032–1038. doi:10.1016/j.apsusc.2015.08.162
Yang, M., Yuan, Y., Li, Y., Sun, X., Wang, S., Liang, L., et al. (2020). Dramatically Enhanced Electromagnetic Wave Absorption of Hierarchical CNT/Co/C Fiber Derived from Cotton and Metal-Organic-Framework. Carbon 161, 517–527. doi:10.1016/j.carbon.2020.01.073
Zhang, K.-L., Zhang, J.-Y., Hou, Z.-L., Bi, S., and Zhao, Q.-L. (2019). Multifunctional Broadband Microwave Absorption of Flexible Graphene Composites. Carbon 141, 608–617. doi:10.1016/j.carbon.2018.10.024
Zhao, H., Cheng, Y., Lv, H., Ji, G., and Du, Y. (2019). A Novel Hierarchically Porous Magnetic Carbon Derived from Biomass for Strong Lightweight Microwave Absorption. Carbon 142, 245–253. doi:10.1016/j.carbon.2018.10.027
Keywords: RGO, CIP, CB, X-band, microwave absorption, reflection loss
Citation: Song Y, Bi S, Hou G, Li H and Liu Z (2022) Heterogeneous Interfaces of Magnetic CIP and Binary Dielectric Carbon-Based Material Toward High-Efficiency Microwave Attenuation. Front. Mater. 9:950399. doi: 10.3389/fmats.2022.950399
Received: 22 May 2022; Accepted: 30 May 2022;
Published: 04 July 2022.
Edited by:
Dawei Wang, Shenzhen Institutes of Advanced Technology (CAS), ChinaReviewed by:
Peng He, Anhui Polytechnic University, ChinaYong Li, Inner Mongolia University of Science and Technology, China
Bo Wen, Shenzhen University, China
Copyright © 2022 Song, Bi, Hou, Li and Liu. This is an open-access article distributed under the terms of the Creative Commons Attribution License (CC BY). The use, distribution or reproduction in other forums is permitted, provided the original author(s) and the copyright owner(s) are credited and that the original publication in this journal is cited, in accordance with accepted academic practice. No use, distribution or reproduction is permitted which does not comply with these terms.
*Correspondence: Song Bi, xiaozhu-youyou@163.com; Zhaohui Liu, lzh5011@163.com