- 1School of Chemical and Materials Engineering (SCME), School of Interdisciplinary Engineering and Sciences (SINES), National University of Sciences and Technology (NUST), Islamabad, Pakistan
- 2School of Chemistry, South China Normal University, Guangzhou, China
Aimed at commercializing the technology of sodium-ion batteries (SIBs), researchers have been trying to produce electrode materials with optimally high charge storage capacity, superior rate capability, extended life, and cost-effective components. Herein, we synthesized an electrode of cobalt selenides loaded in carbon spheres and anchored on reduced graphene (CSSs@rGO) for high-performance SIBs. This improved structure of CSSs@rGO permits the pseudocapacitive storage of charge, thus enhancing the electrical characteristics. It was discovered that the diameter of the carbon sphere had a significant impact on the charge storage capacities of the developed electrode materials, suggesting the probable depth of sodium-ion (Na-ion) movement in the electrode materials during charge and discharge. For instance, CSSs@rGO with an average diameter of ∼70 nm presented the best electrochemical performance as an anode of SIBs. The nano-architecture CSSs@rGO exhibits excellent ion storage capability with a reversible capacity of 600 mA h g-1 at a discharge rate of 100 mA g−1 after 50 cycles. However, at a higher discharge rate (e.g., 1,000 mA g−1), a storage capacity as high as 380 mA h g−1 was achieved. In addition to higher charge storage capability and efficient charge storage at higher discharge rates, the developed CSSs@rGO exhibited stable cycling performance for over 3,000 cycles, which clearly shows the feasibility of our products. This work will open new approaches for developing advanced electrode materials for high-performance sodium-ion batteries.
Introduction
The ubiquitous distribution of sodium metal resources around the world and the low prices of its salts have urged scientists across the globe to consider sodium-ion batteries (SIBs) as a promising alternative to lithium-ion batteries (LIBs) (Slater et al., 2013; Kim et al., 2016; Ali et al., 2020; Rashad et al., 2020; Zhou et al., 2021; Asif et al., 2022). Although the recent studies of SIBs have reported cathode performances comparable to the cathodes of LIBs (Barpanda et al., 2014; Lee et al., 2014; Xiang et al., 2015; Ahsan et al., 2022), the development of an anode with adequately high-energy capacity, sufficiently long cycling life, and applicably low redox potential is still a big challenge for the scientific community. Moreover, the deployment of the already developed LIB anode materials in SIBs has been unsuccessful owing to the greater ionic size and higher molar mass of sodium compared to lithium (Yousaf et al., 2021). Graphite, silicon, and titanium oxide-based materials are effective and mostly used as anodes in LIBs. However, when employed in SIBs, these materials show significant issues (Wang et al., 2013; Wen et al., 2014; Chen et al., 2015; Kim et al., 2015; Asif et al., 2020c). Even an expanded graphite structure can only produce a reversible capacity of 184 mA h g−1 at 100 mA g−1 (Wen et al., 2014). “P2-Na0.66 [Li0.22Ti0.78] O2,” a layered material, was recently introduced, exhibiting only ∼0.77% volume change during sodium insertion/extraction (Wang et al., 2013; Ali et al., 2020). Although it can exhibit an average storage voltage of 0.75 V, it can provide a reversible capacity of only 116 mA h g−1. Silicon can concoct a fully sodiated phase NaSi, but the required activation energy is calculated to be higher than 1 eV, indicating limited uptake of sodium-ion in pristine silicon. A recent report demonstrated that silicon nano-particles can store sodium ions reversibly and deliver a capacity of 279 mA h g−1 at a very slow scan rate of 10 mA g−1 (Xu et al., 2016).
Charge storage mechanisms at the anode could be intercalation, alloying, or conversion. Until now, intercalation-type anode materials such as graphite and Na3V2(PO4)3 have been mostly used (Cai et al., 2018; Dong et al., 2018; Gu et al., 2018; Yousaf et al., 2021; Zhou et al., 2021). This type presents sufficient cyclic performance and rate capabilities but suffers from low energy capacities (i.e., less than 300 mA h g−1) (Yousaf et al., 2021; Zhou et al., 2021). Materials that store charge by making alloys (e.g., P, Sb, and Ge) or conversion (e.g., SnO2, MoS2, and NiSe2) mechanisms usually possess higher energy capacities but lack longer cycling life due to severe structural deteriorations caused by large volume changes (Dahbi et al., 2016; Jiang et al., 2016; Lu et al., 2017; Luo et al., 2017; Asif et al., 2018; Ahsan et al., 2020). To rectify this problem of structural instability, many authors have successfully presented the incorporation of carbon-based additives to make composite or utilization of 2D structures to compensate for volumetric change materials (Huang et al., 2019; Mahmood et al., 2019; Wang et al., 2019). Transition metal chalcogenides (TMCs) are among these conversion mechanism-based anode materials (Ali et al., 2018a; Ali et al., 2018b; Ali et al., 2019; Riaz et al., 2019; Asif et al., 2020b; Mahmood et al., 2020; Rashad et al., 2020; Sajjad et al., 2021; Usman et al., 2022), which have attracted pronounced attraction as anodes of SIBs because of their high theoretical energy capacity, low cost, robust structure, and facile synthesis techniques (Li et al., 2019; Riaz et al., 2019; Ali et al., 2020; Rashad et al., 2020; Wang et al., 2020; Usman et al., 2022). To the best of our knowledge, a spherical structure composed of cobalt selenides in the spherical form embedded on rGO has not been reported yet.
Herein, we present an anode composed of uniquely structured cobalt selenide spheres (CSSs), which are uniformly anchored on reduced graphene oxide (rGO) sheets. These CSSs consist of small crystallites (2–4 nm) of cobalt selenide embedded and dispersed in an amorphous carbon matrix. The carbon matrix not only enhances the electronic properties but also provides the buffers for the volumetric changes during the charge–discharge cycling. CSSs of various diameters (specifically 70, 150, and 300 nm) were produced in this study by controlling the reactant concentrations in a one-step hydrothermal method. Due to their unique structure, CSSs@rGO (with 70 nm diameter spheres) presented extraordinary electrochemical performance as an anode of SIBs. The tapping density of the electrode materials is a big question for the graphene-containing composite electrodes. In our study, tapping density was sufficiently high (1.5 mg cm−2) as we utilized very small concentrations (2–3wt%) of graphene oxide as the precursor. The composite showed high initial discharge capacity (770 mA h g−1 at 100 mA g−1), improved rate capability (770 mA h g−1 at 100 mA g−1 and 200 mA h g−1 at 5,000 mA g−1), and ultra-long cycle life (380 mA h g−1 at 1,000 mA g−1) after 3,000 cycles.
Results and discussion
Cobalt selenide spheres were produced by a facile method (for details, see Experiments and methods) where precursors were dissolved in isopropanol in the presence of glycerol and treated hydrothermally. As the CSSs were formed by the well-renowned crystallization and Ostwald growth mechanism, where growth can be controlled by solution viscosity (Meinders and van Vliet, 2004), we utilized varying amounts of glycerol to tailor viscosity. Hence, CSSs with different diameters were produced, and composites with sphere diameters of 70, 150, and 300 nm were termed CSSs70@rGO, CSSs150@rGO, and CSSs300@rGO, respectively. Although the as-obtained spheres were amorphous, crystalline spheres of cobalt selenide were obtained by annealing at 400°C for 3 h. These spheres consist of nano-crystallites embedded in a carbon matrix within the spheres, as illustrated by the schematic in Figure 1.
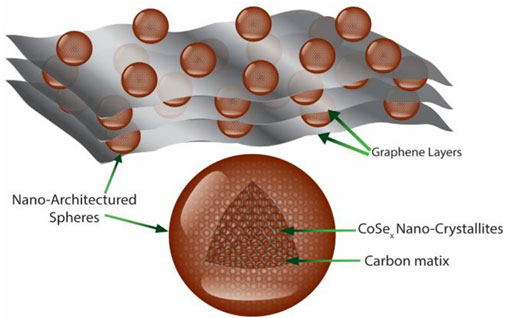
FIGURE 1. Schematic representation of the cobalt selenide spheres embedded on rGO sheets (CSSs@rGO).
X-ray diffraction (XRD) analysis was used to investigate the crystalline phases and compositions. Figure 2 shows XRD patterns in a 2θ range of 5°–80° of the as-obtained, annealed, and anchored on rGO CSSs. The XRD pattern of the as-obtained CSSs does not show the presence of any crystalline phases. However, annealing under optimal conditions (i.e., above recrystallization temperature for a sufficient duration) causes the formation of various crystals. All peaks of the XRD pattern can be attributed to the JCPDS No. 89-2004, indicating the hexagonal symmetry of the CSSs with a space group of P63/mmc. The absence of any other peaks shows good phase purity. Supplementary Figure S1 presents the optimization of annealing conditions for different temperatures and varying durations. XRD peaks become clearer as the annealing temperature is increased from 300 °C to 400°C or 500°C. However, the crystalline nature of the CSSs is not affected prominently by longer than 3 h (i.e., 4 or 5 h) annealing durations.
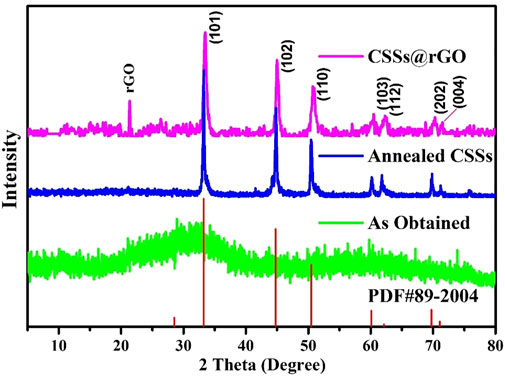
FIGURE 2. X-ray diffraction analysis of the cobalt as obtained cobalt selenide spheres, annealed cobalt selenide spheres (CSS), and composited with rGO cobalt selenide spheres (CSS@rGO).
In order to investigate the unique morphologies of the CSSs@rGO composites, scanning electron microscopy (SEM), transmission electron microscopy (TEM), and high-resolution TEM (HRTEM) were employed. The SEM and TEM analyses confirm the uniform distribution and anchoring of CSS over nanosheets of rGO (Figure 3A–D). Moreover, the high-resolution TEM analysis (Figure 3E) of an individual sphere reveals that the cobalt selenide particles are embedded in an amorphous carbon matrix. Furthermore, lattice fringes with a spacing of 0.26 nm agree well with the (101) plane of the hexagonal cobalt selenide (Figure 3E, inset). CSSs of different diameters obtained by varying the glycerol amount are presented in the TEM image (Supplementary Figures S2A–F). CSSs of nearly 700 nm diameters were produced with ∼5wt% glycerol, and the diameters decreased by increasing the glycerol amount. To produce CSSs in a nanometer range (<100 nm), the glycerol amount used was 25wt%. A further increase in glycerol amount inhibits the formation of the spherical shape of the particles (Supplementary Figure S2I). SEM images of cobalt selenide spheres prepared with and without rGO solution are presented in Supplementary Figures S3A–C, whereas the CSSs produced without adding rGO are presented in Supplementary Figures S3D–F. Monodispersed sphere-like morphologies are evidenced by the TEM and SEM analyses presented in Supplementary Figures S2G,H, and Supplementary Figure S3, respectively. However, all the CSS spheres seem to be firmly anchored over the rGO sheets.
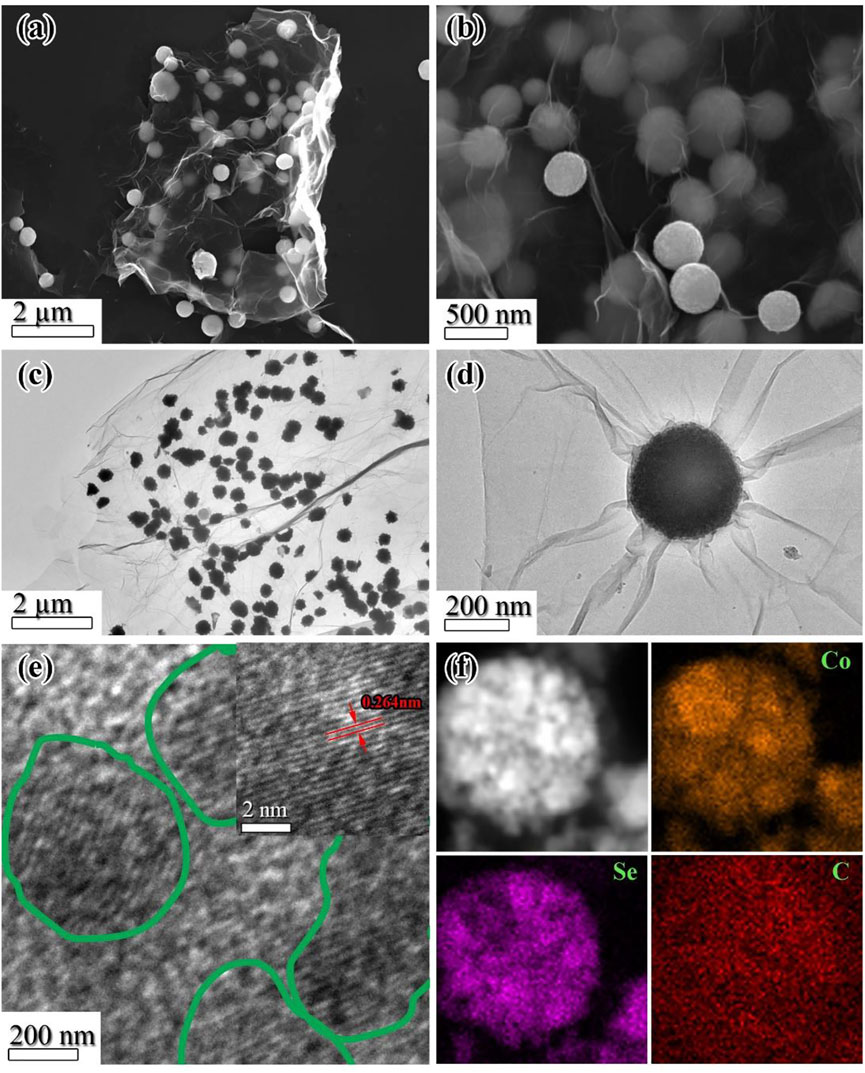
FIGURE 3. (A,B) SEM images. (C,D) TEM images. (E) High-resolution TEM images. (F) EDS elemental mapping of CSSs@rGO.
Elemental mapping of a graphene-embedded cobalt selenide sphere was done using energy dispersive spectroscopy (EDS). Figure 3F confirms the uniform distribution of cobalt, selenium, and carbon across the sphere. Due to the presence of graphene, the carbon signal around the sphere is also high. Hence, composites with cobalt selenide spheres anchored on reduced graphene oxide are ascertained. Such composites are expected to perform extraordinarily as an anode of sodium-ion batteries owing to the enhanced surface area, intimate contact with rGO, and monodispersed nature of chalcogenide species in carbon matrices (Asif et al., 2020a; Maqbool et al., 2020).
The electrochemical performance of the CSSs@rGO was examined by fabricating 2032-type coin cells with sodium metal foil as reference and counter electrode. The literature reveals that ether-based electrolytes yield the best results for TMC-based anodes (Ganesan et al., 2015; Zhang et al., 2015; Zhang et al., 2016a; Zhang et al., 2016b; Ko et al., 2016; Luo et al., 2016; Peng et al., 2016; Su et al., 2016; Zhu et al., 2017). Therefore, an electrolyte containing 1.0 M NaCF3SO3 in an ether-based solvent [diethylene glycol (DEG) and dimethyl ether (DME) in a 1:1 volume ratio] was used in this study. For good cycle stability, the scan voltage window is critical (Xu et al., 2013; Hu et al., 2015; Kim et al., 2015). Thus, varying voltage windows were used in previous reports to optimize the cycling performance. Our initial CV tests revealed that no peaks are present in the voltage range of 0.01–0.5 and 2.5–3.0 V. Hence, learning from CV tests and previous studies, we have compared the cyclic performance of only two voltage ranges (i.e., 0.1–2.8 and 0.5–2.8 V) at a scan rate of 2000 mAg−1. In order to observe a charge–discharge behavior in detail, CV tests were performed for all composites of CSSs@rGO, showing similar behavior to that presented in Figure 4A. In the first cycle, the cathodic peak at 0.95 V can be associated with the solid electrolyte layer (SEI) formation. However, the anodic peaks of 1.75 and 1.81 V can be related to the formation of NaxCoSe2 and cobalt selenide successively (Tang et al., 2017). From the second cycle onward, the strong cathodic peak at 1.14 V evidences the formation of CoSe2 and Na2Se together, whereas a shallow peak around 0.65 V confirms the formation of Co metal and Na2Se (Cui et al., 2018). Meanwhile, the anodic peak of 1.81 V confirms the reformation of cobalt selenide from Na2Se. The charge–discharge behavior for the 1st and 10th cycles (Figure 4C) is consistent with the CV data, proving the validity of these findings. Reversible Na-ion storage causes changes in the morphology and electrochemical activity of electrode materials, increasing the charge storage capacity (Zhang et al., 2015; Zhang et al., 2016a; Liu et al., 2016). However, the formation of unstable SEI or phase changes (activation) of material structure may reduce the charge storage capacity (Ge et al., 2018). Reports on cobalt selenide have described both the descent and increase in capacity as a result of these competing mechanisms (Zhang et al., 2015; Zhang et al., 2016a; Liu et al., 2016; Ge et al., 2018). We observed a steady drop in the charge storage capacity of CSSs@rGO throughout the first 70 cycles until it reached a steady level. During these cycles, a discharge plateau at 1.14 V and a charge plateau at 1.82 V are shown in charge–discharge curves (Figure 4C), consistent with the CV results of Figure 4A. The charge–discharge patterns undergo a significant reorganization throughout the subsequent cycles. After the activation (rearrangement), the (dis)charge patterns become stable. Subsequently, the material shows extraordinary stability even after 3,000 cycles. Similar activation behavior was reported and described in the literature because of the morphological or phase changes in electrode materials (Zhang et al., 2016a; Ali et al., 2018b; Ge et al., 2018). After the activation process, CV tests were conducted at 1,000, 2,000, and 3,000 cycles (Figure 4B), showing overlapping patterns indicating the materials’ persistent electrochemical activity. These CV scans contain four cathodic peaks: 1.83 V for Na-ion adsorption, 1.69 V for the formation of NaxCoSe2 intermediate phase, 1.14 V for the generation reaction of CoSe2 and Na2Se, and around 0.65 V confirming the formation of Co metal and Na2Se. Each of these CV scans depicted two anodic peaks (centered at 1.5 and 1.9 V) corresponding to the regeneration of Na ions from the NaxCoSe2 intermediate phase and Na2Se, respectively (Tang et al., 2017; Cui et al., 2018). These CV curves were consistent with discharge–charge curves shown in Figure 4C.
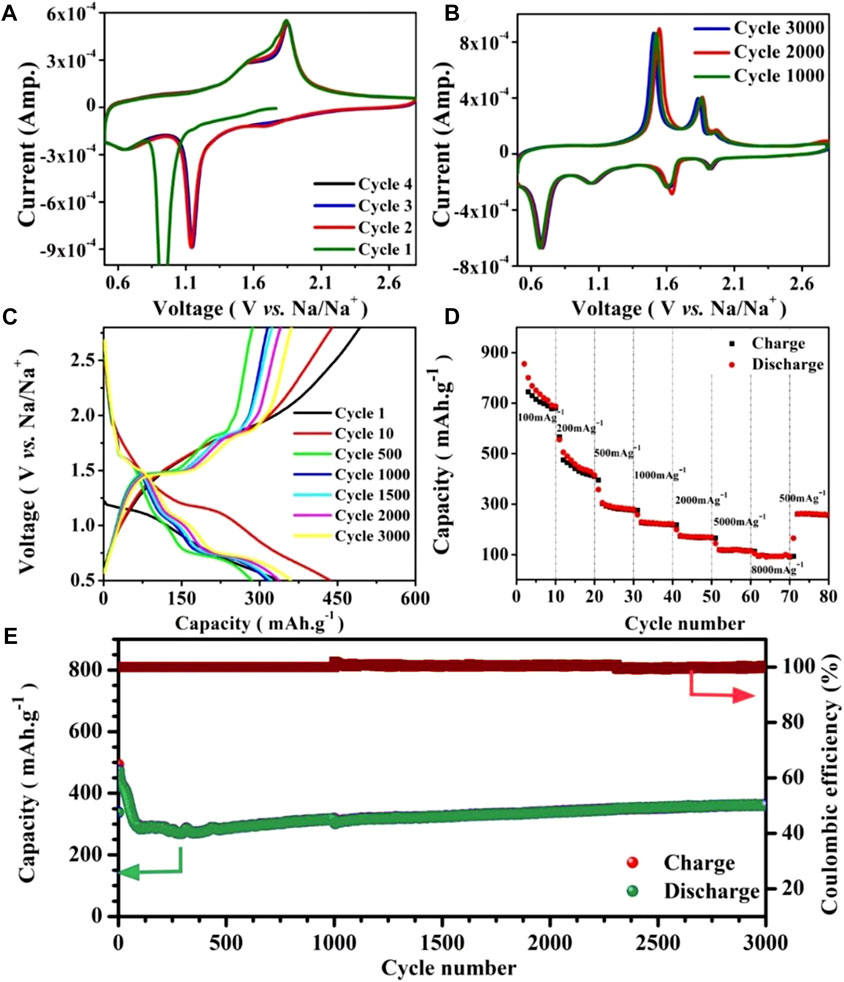
FIGURE 4. Electrochemical performance of CSSs70@rGO, (A) CV curves of as-prepared, and (B) cycled cells at the scan rate of 1 mV s−1 between 0.5 and 2.8 V. (C) Charge–discharge curves at 1,000 mA g−1 scan rate. (D) Rate performance of CSSs70@rGO. (E) Long-term cyclic performance of CSSs70@rGO at the scan rate of 1,000 mA g−1.
The performance rate of the CSSs70@rGO is presented in Figure 4D. As the current densities gradually increase from 100 to 200, 500, 1,000, and 2,000 mA g−1, the discharge capacities change from 855 to 555, 358, 258, and 200 mA h g−1, respectively. Even at ultrahigh current densities of 5,000 and 10,000 mA g−1, the discharge capacities remain 144 and 105 mA h g−1, respectively. Moreover, when the current density is reduced back to 500 mA g−1, the capacity can recover to 263 mA h g−1. This outstanding rate capability could arise from the intimate contact between crystallites of cobalt selenide and carbon matrix, which helps in faster ion/charge transfer at higher scan rates.
The long-term cycling performance of the CSSs70@rGO at a current rate of 1,000 mA g−1 is presented in Figure 4E. The energy capacity becomes stable after about 80 cycles, and the capacity values can reach 350 mA h g−1 even after 3,000 cycles at a scan rate of 1,000 mA g−1, which is 110 % and 72% of those at the 80th and 5th cycles, respectively. Specific capacity increases after 500 cycles because Na+ ions could diffuse to the outer reduced graphene oxide before they get to the active material. Therefore, the Na+ ions may diffuse into the CSS via vacancies or defects present in the reduced graphene oxide instead of the intercalation mechanism (Yousaf et al., 2019). The coulombic efficiency always ranges between 99.66 % and 102.21% for all the cycles, except for the first cycle.
Such outstanding electrochemical properties and extraordinary cyclability (380 mA h g−1 even after 3,000 cycles at 1 A g−1) render the CSSs@rGO as a potential anode material for SIBs. These unparalleled properties can be attributed to some reasons. First, carbon matrix existence in material improves the electronic conductivity and provides the buffer for volumetric changes during intercalation/deintercalation of ions. Second, for the redox processes, the major portion of charge storage is pseudocapacitive behaviors. The last but most important reason is the intimate contact between crystallites of cobalt selenide and the carbon matrix of miniaturized spheres, which makes a smooth transfer of charges during repeated cycles and eases off the kinetic impedance associated with the transport of ions through the material.
Conclusion
In summary, the monodispersed spheres of cobalt selenide embedded on rGO (CSSs@rGO) were produced via a one-step hydrothermal process. Controllable, different sizes of spheres were synthesized by varying reactant concentrations. As an anode of SIBs, the CSSs@rGO presented an extraordinarily reversible specific capacity of 390 mA h g−1 even after 3,000 cycles at the scan rate of 1,000 mA g−1. At a slow scan rate such as 100 mA g−1, the specific capacity was as high as 554 mA h g−1 after 50 cycles. This outstanding cycling performance can be attributed to the intimate contact between crystallites of cobalt selenide and the carbon matrix of nano-spheres. Moreover, the pseudocapacitive behaviors in the redox reaction also take part in enhancing rate capability and cyclability. Such intriguing electrochemical properties render CSSs@rGO a potential material for SIBs anode.
Experiments and methods
Synthesis of CSSs and CSS@rGO
CSSs were produced via a facile single-step hydrothermal process. In a typical synthesis; 0.3 mol of Co(NO₃)₂.6H₂O (cobalt nitrate hexahydrate) and 0.1 mol of H3O2Se (selenous acid) were dissolved in 40 ml of (CH3)2CHOH (isopropanol) + 20% glycerol, by magnetic stirring at 40°C for half an hour to obtain a dark purple solution, to which a separately prepared 5 ml rGO/isopropanol solution of 1 mg ml−1 was added. This solution was transferred to a Teflon-lined stainless-steel container and treated hydrothermally for 6 hours at 120°C–150°C. After natural cooling to room temperature, the product was centrifuged and washed thoroughly with absolute ethanol several times. As-obtained purple precipitates were dried at 70°C for 12 h under vacuum conditions. The product was then annealed at 400°C under a nitrogen environment for 5 h with a heating rate of 5°C per min. Then, reduced graphene oxide sheets were mixed with annealed material in absolute ethanol and sonicated for 1 h to obtain CoSe2@GO composite.
Material characterization
X-ray diffraction (XRD) studies were done using a PAN analytical X’Pert-3 Powder X-ray diffractometer equipped with Cu Kα radiation. The accelerating voltage and current were 40 kV and 40 mA, respectively. The XRD analysis was performed in the 2θ range of 5°–8 0°. The morphological characterization of the product was carried out using FEI Tecnai T20, F20, and F30 transmission electron microscopes. The elemental composition of composites was analyzed by an energy dispersive spectroscopy detector equipped with F30 and X-ray photoelectron spectroscopy (XPS), Kratos Axis Ultra with monochromatized Al Kα radiation (1,486.6 eV). The BET analysis was done using ASAP 2010. The thermogravimetric analysis (TGA) was carried out by an SDT Q600 (USA) in argon at a heating rate of 5°C per min from 25°C to 900°C.
Electrochemical analysis
The working electrode was prepared by mixing the active materials (CSSs or CSSs@rGO), conductive agent (acetylene black), and binder (carboxymethylcellulose sodium) in a weight ratio of 80:10:10, respectively. A homogenous slurry (obtained by mixing in deionized water) was then pasted onto a well-cleaned Cu foil and dried at 70°C for 24 h under a vacuum. The final mass loading of active material was between 1.00 and 1.2 mg cm−2. Na foil cut into circular disks was used as reference and counter electrodes. A glass fiber filter paper was used as a separator. The 2032-type coin half cells were fabricated in an Ar-filled glovebox. The electrolyte was 1 mol dm−3 of sodium trifluoro-methane-sulfonate (NaCF3SO3) in an ether-based solvent (diethylene glycol (DEG) and dimethyl ether (DME) in 1:1 volume ratio). Electrochemical measurements of the prepared half cells were carried out with a LAND CT 2001A analyzer at different current densities with a potential window of 0.1–2.8 V vs. Na+/Na. Cyclic Voltammetry tests were performed in the same voltage range, and electrochemical impedance spectroscopy (EIS) was carried out in the frequency range of 100 kHz to 100 mHz with a sinusoidal voltage signal of 5 mV using CHI 760C equipment (Shanghai Chenhua).
Data availability statement
The original contributions presented in the study are included in the article/Supplementary Material. Further inquiries can be directed to the corresponding author.
Author contributions
The manuscript was written with the contributions of all authors. All authors have given approval to the final version of the manuscript.
Funding
This work was supported by the seed funding scheme of the National University of Sciences and Technology (NUST), H-12, Islamabad, and Pakistan Science Foundation Grant PSF-NSFC-IV/Eng/C-NUST (22).
Conflict of interest
The authors declare that the research was conducted in the absence of any commercial or financial relationships that could be construed as a potential conflict of interest.
Publisher’s note
All claims expressed in this article are solely those of the authors and do not necessarily represent those of their affiliated organizations or those of the publisher, the editors, and the reviewers. Any product that may be evaluated in this article, or claim that may be made by its manufacturer, is not guaranteed or endorsed by the publisher.
Supplementary material
The Supplementary Material for this article can be found online at: https://www.frontiersin.org/articles/10.3389/fmats.2022.950673/full#supplementary-material
References
Ahsan, M. T., Ali, Z., Usman, M., and Hou, Y. (2022). Unfolding the structural features of NASICON materials for sodium-ion full cells. Carbon Energy, 1–44. doi:10.1002/cey2.222/cey2.222
Ahsan, M. T., Usman, M., Ali, Z., Javed, S., Ali, R., Farooq, M. U., et al. (2020). 3D hierarchically mesoporous zinc-nickel-cobalt ternary oxide (Zn0.6Ni0.8Co1.6O4) nanowires for high-performance asymmetric supercapacitors. Front. Chem. 8 (487). doi:10.3389/fchem.2020.00487
Ali, Z., Asif, M., Huang, X., Tang, T., and Hou, Y. (2018a). Hierarchically porous Fe2CoSe4 binary-metal selenide for extraordinary rate performance and durable anode of sodium-ion batteries. Adv. Mat. 30 (32), 1802745. doi:10.1002/adma.201802745
Ali, Z., Asif, M., Zhang, T., Huang, X., and Hou, Y. (2019). General approach to produce nanostructured binary transition metal selenides as high-performance sodium ion battery anodes. Small 15 (33), 1901995. doi:10.1002/smll.201901995
Ali, Z., Tang, T., Huang, X., Wang, Y., Asif, M., and Hou, Y. (2018b). Cobalt selenide decorated carbon spheres for excellent cycling performance of sodium ion batteries. Energy Storage Mater. 13, 19–28. doi:10.1016/j.ensm.2017.12.014
Ali, Z., Zhang, T., Asif, M., Zhao, L., Yu, Y., and Hou, Y. (2020). Transition metal chalcogenide anodes for sodium storage. Mater. Today 35, 131–167. doi:10.1016/j.mattod.2019.11.008
Asif, M., Ali, Z., Ali, M., and Rashad, M. (2022). Investigating role of ammonia in nitrogen-doping and suppressing polyselenide shuttle effect in Na-Se batteries. J. colloid interface Sci. 617, 641–650. doi:10.1016/j.jcis.2022.03.024
Asif, M., Ali, Z., Qiu, H., Rashad, M., and Hou, Y. (2020a). Confined polysulfide shuttle by nickel disulfide nanoparticles encapsulated in graphene nanoshells synthesized by cooking oil. ACS Appl. Energy Mat. 3 (4), 3541–3552. doi:10.1021/acsaem.0c00072
Asif, M., Rashad, M., Ali, Z., and Ahmed, I. (2020c). Synthesis of ternary metal oxides as positive electrodes for Mg–Li hybrid ion batteries. Nanoscale 12 (2), 924–932. doi:10.1039/C9NR08758C
Asif, M., Rashad, M., and Ali, Z. (2020b). Electrochemical intercalations of divalent ions inside Ni/Zn co-doped cobalt sulfide nanoparticle decorated carbon spheres with superior capacity. Nanoscale 12 (26), 14267–14278. doi:10.1039/D0NR02761H
Asif, M., Rashad, M., Ali, Z., Qiu, H., Li, W., Pan, L., et al. (2018). Ni-doped MnO2/CNT nanoarchitectures as a cathode material for ultra-long life magnesium/lithium hybrid ion batteries. Mater. Today Energy 10, 108–117. doi:10.1016/j.mtener.2018.08.010
Barpanda, P., Oyama, G., Nishimura, S.-i., Chung, S.-C., and Yamada, A. (2014). A 3.8-V earth-abundant sodium battery electrode. Nat. Commun. 5, 4358. doi:10.1038/ncomms5358
Cai, Y. S., Cao, X. X., Luo, Z. G., Fang, G. Z., Liu, F., Zhou, J., et al. (2018). Caging Na3V2(PO4)(2)F-3 microcubes in cross-linked graphene enabling ultrafast sodium storage and long-term cycling. Adv. Sci. (Weinh). 5 (9), 1800680. doi:10.1002/advs.201800680
Chen, Y., Liu, L., Xiong, J., Yang, T., Qin, Y., and Yan, C. (2015). Porous Si nanowires from cheap metallurgical silicon stabilized by a surface oxide layer for lithium ion batteries. Adv. Funct. Mat. 25 (43), 6701–6709. doi:10.1002/adfm.201503206
Cui, C., Wei, Z. X., Zhou, G., Wei, W. F., Ma, J. M., Chen, L. B., et al. (2018). Quasi-reversible conversion reaction of CoSe2/nitrogen-doped carbon nanofibers towards long-lifetime anode materials for sodium-ion batteries. J. Mat. Chem. A Mat. 6 (16), 7088–7098. doi:10.1039/c8ta01168k
Dahbi, M., Yabuuchi, N., Fukunishi, M., Kubota, K., Chihara, K., Tokiwa, K., et al. (2016). Black phosphorus as a high-capacity, high-capability negative electrode for sodium-ion batteries: Investigation of the electrode/electrolyte interface. Chem. Mat. 28 (6), 1625–1635. doi:10.1021/acs.chemmater.5b03524
Dong, B., Ju, Y., Huang, X., Li, W., Ali, Z., Yin, H., et al. (2018). A general strategy for facile synthesis of ultrathin transition metal hydroxide nanosheets. Nanoscale 11 (12), 5141–5144. doi:10.1039/C8NR09492F
Ganesan, P., Prabu, M., Sanetuntikul, J., and Shanmugam, S. (2015). Cobalt sulfide nanoparticles grown on nitrogen and sulfur codoped graphene oxide: An efficient electrocatalyst for oxygen reduction and evolution reactions. ACS Catal. 5 (6), 3625–3637. doi:10.1021/acscatal.5b00154
Ge, P., Hou, H. S., Li, S. J., Huang, L. P., and Ji, X. B. (2018). Three-dimensional hierarchical framework assembled by cobblestone-like CoSe2@C nanospheres for ultrastable sodium-ion storage. ACS Appl. Mat. Interfaces 10 (17), 14716–14726. doi:10.1021/acsami.8b01888
Gu, E. L., Liu, S. H., Zhang, Z. Z., Fang, Y. Y., Zhou, X. S., and Bao, J. C. (2018). An efficient sodium-ion battery consisting of reduced graphene oxide bonded Na3V2(PO4)(3) in a composite carbon network. J. Alloys Compd. 767, 131–140. doi:10.1016/j.jallcom.2018.07.082
Hu, Z., Zhu, Z., Cheng, F., Zhang, K., Wang, J., Chen, C., et al. (2015). Pyrite FeS2 for high-rate and long-life rechargeable sodium batteries. Energy Environ. Sci. 8 (4), 1309–1316. doi:10.1039/C4EE03759F
Huang, X., Shen, T., Zhang, T., Qiu, H., Gu, X., Ali, Z., et al. (2019). Efficient oxygen reduction catalysts of porous carbon nanostructures decorated with transition metal species. Adv. Energy Mat. 10 (11), 1900375. doi:10.1002/aenm.201900375
Jiang, Q., Xu, L., Chen, N., Zhang, H., Dai, L., and Wang, S. (2016). Facile synthesis of black phosphorus: An efficient electrocatalyst for the oxygen evolving reaction. Angew. Chem. Int. Ed. 55 (44), 13849–13853. doi:10.1002/anie.201607393
Kim, H., Hong, J., Park, Y.-U., Kim, J., Hwang, I., and Kang, K. (2015). Sodium storage behavior in natural graphite using ether-based electrolyte systems. Adv. Funct. Mat. 25 (4), 534–541. doi:10.1002/adfm.201402984
Kim, H., Kim, H., Ding, Z., Lee, M. H., Lim, K., Yoon, G., et al. (2016). Recent progress in electrode materials for sodium-ion batteries. Adv. Energy Mat. 6 (19), 1600943–n/a. doi:10.1002/aenm.201600943
Ko, Y. N., Choi, S. H., and Kang, Y. C. (2016). Hollow cobalt selenide microspheres: Synthesis and application as anode materials for Na-ion batteries. ACS Appl. Mat. Interfaces 8 (10), 6449–6456. doi:10.1021/acsami.5b11963
Lee, H.-W., Wang, R. Y., Pasta, M., Woo Lee, S., Liu, N., and Cui, Y. (2014). Manganese hexacyanomanganate open framework as a high-capacity positive electrode material for sodium-ion batteries. Nat. Commun. 5, 5280. doi:10.1038/ncomms6280
Li, Y., Wang, Z., Ali, Z., Tian, K., Xu, J., Li, W., et al. (2019). Monodisperse Fe3O4 spheres: Large-scale controlled synthesis in the absence of surfactants and chemical kinetic process. Sci. China Mat. 62 (10), 1488–1495. doi:10.1007/s40843-019-9466-x
Liu, X., Zhang, K., Lei, K., Li, F., Tao, Z., and Chen, J. (2016). Facile synthesis and electrochemical sodium storage of CoS2 micro/nano-structures. Nano Res. 9 (1), 198–206. doi:10.1007/s12274-016-0981-5
Lu, Y. Y., Zhang, N., Jiang, S., Zhang, Y. D., Zhou, M., Tao, Z. L., et al. (2017). High-capacity and ultrafast Na-ion storage of a self-supported 3D porous antimony persulfide-graphene foam architecture. Nano Lett. 17 (6), 3668–3674. doi:10.1021/acs.nanolett.7b00889
Luo, W., Calas, A., Tang, C., Li, F., Zhou, L., and Mai, L. (2016). Ultralong Sb2Se3 nanowire-based free-standing membrane anode for lithium/sodium ion batteries. ACS Appl. Mat. Interfaces 8 (51), 35219–35226. doi:10.1021/acsami.6b11544
Luo, W., Gaumet, J.-J., and Mai, L.-Q. (2017). Antimony-based intermetallic compounds for lithium-ion and sodium-ion batteries: Synthesis, construction and application. Rare Met. 36 (5), 321–338. doi:10.1007/s12598-017-0899-4
Mahmood, A., Ali, Z., Tabassum, H., Akram, A., Aftab, W., Ali, R., et al. (2020). Carbon fibers embedded with iron selenide (Fe3Se4) as anode for high-performance sodium and potassium ion batteries. Front. Chem. 8 (408), 408. doi:10.3389/fchem.2020.00408
Mahmood, A., Li, S., Ali, Z., Tabassum, H., Zhu, B., Liang, Z., et al. (2019). Ultrafast sodium/potassium-ion intercalation into hierarchically porous thin carbon shells. Adv. Mat. 31 (2), 1805430. doi:10.1002/adma.201805430
Maqbool, M., Guo, H., Bashir, A., Usman, A., Abid, A. Y., He, G., et al. (2020). Enhancing through-plane thermal conductivity of fluoropolymer composite by developing in situ nano-urethane linkage at graphene—Graphene interface. Nano Res. 13, 2741–2748. doi:10.1007/s12274-020-2921-7
Meinders, M. B. J., and van Vliet, T. (2004). The role of interfacial rheological properties on Ostwald ripening in emulsions. Adv. Colloid Interface Sci. 108-109, 119–126. doi:10.1016/j.cis.2003.10.005
Peng, S., Han, X., Li, L., Zhu, Z., Cheng, F., Srinivansan, M., et al. (2016). Unique cobalt sulfide/reduced graphene oxide composite as an anode for sodium-ion batteries with superior rate capability and long cycling stability. Small 12 (10), 1359–1368. doi:10.1002/smll.201502788
Rashad, M., Asif, M., and Ali, Z. (2020). Quest for magnesium-sulfur batteries: Current challenges in electrolytes and cathode materials developments. Coord. Chem. Rev. 415, 213312. doi:10.1016/j.ccr.2020.213312
Riaz, M. S., Yuan, X., Zhao, Y., Dong, C., Nong, S., Ali, Z., et al. (2019). Porous NiCo2S4/Co9S8 microcubes templated by sacrificial ZnO spheres as an efficient bifunctional oxygen electrocatalyst. Adv. Sustain. Syst. 3 (5), 1800167. doi:10.1002/adsu.201800167
Sajjad, A., Bhatti, S. H., Ali, Z., Jaffari, G. H., Khan, N. A., Rizvi, Z. F., et al. (2021). Photoinduced fabrication of zinc oxide nanoparticles: Transformation of morphological and biological response on light irradiance. ACS Omega 6 (17), 11783–11793. doi:10.1021/acsomega.1c01512
Slater, M. D., Kim, D., Lee, E., and Johnson, C. S. (2013). Sodium-ion batteries. Adv. Funct. Mat. 23 (8), 947–958. doi:10.1002/adfm.201200691
Su, D., Kretschmer, K., and Wang, G. (2016). Improved electrochemical performance of Na-ion batteries in ether-based electrolytes: A case study of ZnS nanospheres. Adv. Energy Mat. 6 (2), 1501785–n. doi:10.1002/aenm.201501785
Tang, Y., Zhao, Z., Hao, X., Wang, Y., Liu, Y., Hou, Y., et al. (2017). Engineering hollow polyhedrons structured from carbon-coated CoSe2 nanospheres bridged by CNTs with boosted sodium storage performance. J. Mat. Chem. A Mat. 5 (26), 13591–13600. doi:10.1039/C7TA02665J
Usman, M., Ahsan, M. T., Javed, S., Ali, Z., Zhan, Y., Ahmed, I., et al. (2022). Facile synthesis of iron nickel cobalt ternary oxide (FNCO) mesoporous nanowires as electrode material for supercapacitor application. J. Materiomics 8, 221–228. doi:10.1016/j.jmat.2021.03.012
Wang, Y., Li, M., Xu, L., Tang, T., Ali, Z., Huang, X., et al. (2019). Polar and conductive iron carbide@N-doped porous carbon nanosheets as a sulfur host for high performance lithium sulfur batteries. Chem. Eng. J. 358, 962–968. doi:10.1016/j.cej.2018.10.086
Wang, Y. S., Yu, X. Q., Xu, S. Y., Bai, J. M., Xiao, R. J., Hu, Y. S., et al. (2013). A zero-strain layered metal oxide as the negative electrode for long-life sodium-ion batteries. Nat. Commun. 4, 2365. doi:10.1038/ncomms3365
Wang, Z., Li, Z., Sun, Z., Wang, S., Ali, Z., Zhu, S., et al. (2020). Visualization nanozyme based on tumor microenvironment “unlocking” for intensive combination therapy of breast cancer. Sci. Adv. 6 (48), eabc8733. doi:10.1126/sciadv.abc8733
Wen, Y., He, K., Zhu, Y., Han, F., Xu, Y., Matsuda, I., et al. (2014). Expanded graphite as superior anode for sodium-ion batteries. Nat. Commun. 5, 4033. doi:10.1038/ncomms5033
Xiang, X., Zhang, K., and Chen, J. (2015). Recent advances and prospects of cathode materials for sodium-ion batteries. Adv. Mat. 27 (36), 5343–5364. doi:10.1002/adma.201501527
Xu, Y., Swaans, E., Basak, S., Zandbergen, H. W., Borsa, D. M., and Mulder, F. M. (2016). Reversible Na-ion uptake in Si nanoparticles. Adv. Energy Mat. 6 (2), 1501436–n/a. doi:10.1002/aenm.201501436
Xu, Y., Zhu, Y., Liu, Y., and Wang, C. (2013). Electrochemical performance of porous carbon/tin composite anodes for sodium-ion and lithium-ion batteries. Adv. Energy Mat. 3 (1), 128–133. doi:10.1002/aenm.201200346
Yousaf, M., Naseer, U., Li, Y., Ali, Z., Mahmood, N., Wang, L., et al. (2021). A mechanistic study of electrode materials for rechargeable batteries beyond lithium ions by in situ transmission electron microscopy. Energy Environ. Sci. 14 (5), 2670–2707. doi:10.1039/d0ee03295f
Yousaf, M., Wang, Y., Chen, Y., Wang, Z., Firdous, A., Ali, Z., et al. (2019). A 3D trilayered CNT/MoSe2/C heterostructure with an expanded MoSe2 interlayer spacing for an efficient sodium storage. Adv. Energy Mat. 9 (30), 1900567. doi:10.1002/aenm.201900567
Zhang, K., Hu, Z., Liu, X., Tao, Z., and Chen, J. (2015). FeSe2 microspheres as a high-performance anode material for Na-ion batteries. Adv. Mat. 27 (21), 3305–3309. doi:10.1002/adma.201500196
Zhang, K., Park, M., Zhou, L., Lee, G.-H., Li, W., Kang, Y.-M., et al. (2016). Urchin-like CoSe2 as a high-performance anode material for sodium-ion batteries. Adv. Funct. Mat. 26, 6728–6735. doi:10.1002/adfm.201602608
Zhang, K., Park, M., Zhou, L., Lee, G.-H., Shin, J., Hu, Z., et al. (2016b). Cobalt-Doped FeS2 nanospheres with complete solid solubility as a high-performance anode material for sodium-ion batteries. Angew. Chem. Int. Ed. 55 (41), 12822–12826. doi:10.1002/anie.201607469
Zhou, J., Zeng, C., Ou, H., Yang, Q., Xie, Q., Zeb, A., et al. (2021). Metal–organic framework-based materials for full cell systems: A review. J. Mat. Chem. C Mat. 9 (34), 11030–11058. doi:10.1039/d1tc01905h
Keywords: cobalt selenide, graphene, sodium-ion batteries, hydrothermal and solvothermal synthesis, nano-architecture
Citation: Ali Z, Ali M, Mehmood A, Ishfaq A, Akram MA, Zeb A and Lin X (2022) Nano-architectured cobalt selenide spheres anchored on graphene oxide sheets for sodium ion battery anode. Front. Mater. 9:950673. doi: 10.3389/fmats.2022.950673
Received: 23 May 2022; Accepted: 28 July 2022;
Published: 29 August 2022.
Edited by:
Mohammad Islam, GE Aviation Systems, United StatesReviewed by:
Asif Mahmood, The University of Sydney, AustraliaHaris Ansari, University of Calgary, Canada
Copyright © 2022 Ali, Ali, Mehmood, Ishfaq, Akram, Zeb and Lin. This is an open-access article distributed under the terms of the Creative Commons Attribution License (CC BY). The use, distribution or reproduction in other forums is permitted, provided the original author(s) and the copyright owner(s) are credited and that the original publication in this journal is cited, in accordance with accepted academic practice. No use, distribution or reproduction is permitted which does not comply with these terms.
*Correspondence: Zeeshan Ali, emVlc2hhbi5hbGlAc2NtZS5udXN0LmVkdS5waw==; Xiaoming Lin, bGlueG1Ac2NudS5lZHUuY24=