- 1Guangdong Engineering Research Center for Translation of Medical 3D Printing Application, Guangdong Provincial Key Laboratory of Digital Medicine and Biomechanics, National Key Discipline of Human Anatomy, School of Basic Medical Sciences, Southern Medical University, Guangzhou, China
- 2Department of Stomatology, Affiliated Hospital of Guangdong Medical University, Zhanjiang, China
With the development of sustainable materials, biomass-derived fiber materials have attracted significant research interest because of their excellent performance, cost-effectiveness, and environmental friendliness. Following their extensive use in biomedical applications, biomass-derived fiber materials are now increasingly used in antibacterial skin-wound dressings, bone tissue engineering, drug delivery, etc. However, their practical medical applications are still in their infancy, requiring some reference and strategic directions for the future clinical applications of biomaterials in medicine and cross-discipline use, some of which are provided in this review. The sources and characteristics of the different biomass-derived fibers are introduced briefly, encompassing polysaccharide fiber and protein fiber’s two categories. Then, we summarize the common applications of biomass and its derived fibers in the medical field in recent years. Finally, we discuss the application and advantages of three-dimensional printing technology combined with biomass-derived materials in modern medicine for a better understanding of the practical applications of biomass-derived fiber materials.
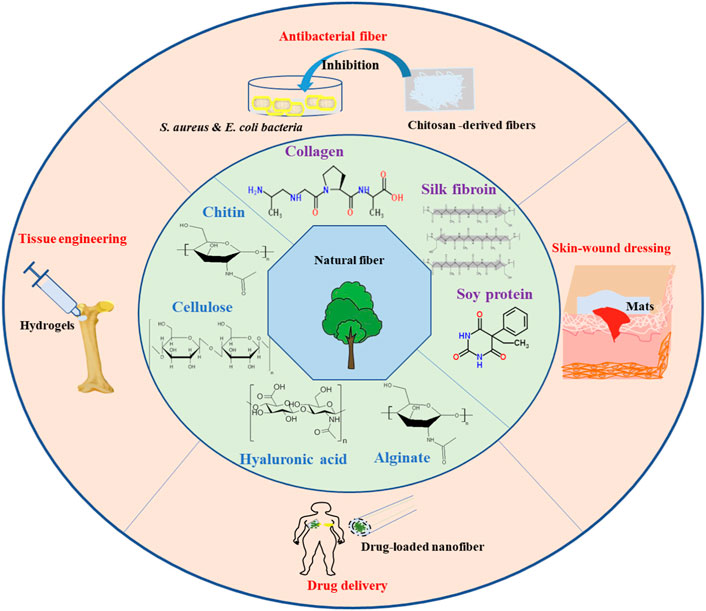
GRAPHICAL ABSTRACT | Schema of biomass-derived fiber materials (cellulose, chitin, alginate, silk fibroin, collagen, hyaluronic acid, etc.,) for biomedical applications, such as antibacterial skin-wound dressings, tissue engineering, and drug delivery.
1 Introduction
Polymers extracted from traditional petroleum materials have significantly promoted the development of modern life. However, petroleum-derived polymers cause environmental pollution and are non-renewable. Green chemistry makes chemical reactions and processes more environment-friendly (Pleissner and Kummerer, 2020). Over the past few years, renewable resources have provided an opportunity for the sustainable development of function by allowing us to use biomass materials in our daily lives (Srivastava et al., 2021). Biomass materials without chemical modification are mainly composed of carbon, hydrogen, and oxygen and are degraded easily by natural microorganisms into water, carbon dioxide, and other small molecules, which means their products can reenter the natural cycle. Therefore, advanced green biomass fibers, such as lignin-based carbon fibers and nanofibers, which are based on renewable resources, have great potential as sustainable composites (Venkata Mohan et al., 2016).
The sources of biomass materials are abundant and widely distributed. Biomass-derived materials mainly include polysaccharide fiber materials, such as cellulose, chitin, starch, sodium alginate, pectin, hyaluronic acid (HA), etc., and protein fiber materials, such as silk fibroin, collagen, corn protein, and soy protein. At present, biomass-derived fiber materials have been used in environmental protection (Hassan and Carr, 2021), biosensor sensors (Liedel, 2020), photocatalysts (Son et al., 2021), and others applications. Indeed, as long as specific quality measures are satisfied, biomass-derived fiber materials are promising candidates for the biomedical field. The applications of biomass-derived fibers have, recently, been extended from antibacterial skin-wound dressings to bone tissue engineering, artificial blood vessels, drug-sustained release, and other areas, because of their excellent biodegradability, non-toxicity, renewability, and polymer chemical reaction. For example, Huang et al. prepared injectable hydrogels for the treatment of deep burn wounds by mixing modified cellulose nanofibers with carboxymethyl chitosan (CS) (Huang et al., 2018). Kawata et al. demonstrated that calcium phosphate/chitin nanofiber hydrogels could promote the formation of calcium phosphate crystals, which can improve the mechanical properties of scaffolds according to mineralization time (Kawata et al., 2016). Additionally, with the development of three-dimensional (3D) printing technology in biomass-derived materials, the development of multiscale, multilateral, and multifunctional structures for biomedical products will be a trend in the future. Huang et al. fabricated a lung tissue scaffold with 10 layers with high shape fidelity and fiber alignment via 3D printing technology (Huang et al., 2021); Tsukamoto et al developed 3D cardiac scaffolds with an oriented structure, which possess better contractile properties and orientation-controlled structure (Tsukamoto et al., 2020); Sun et al prepared a 3D printed collagen/chitosan scaffold to ameliorate axon regeneration and neurological recovery after spinal cord injury (Sun et al., 2019). With the development of 3D printing technology, biomass-derived materials will be more suitable for the treatment of fine structures and complex tissues in the future.
In this review, we attempt to provide readers with an overview of the sources and characteristics of different biomass-derived fibers and a comprehensive study of the common applications of biomass and their derived fibers in the medical field in recent years. Moreover, we put special emphasis on the applications of biomass-derived fibers as bioinks for 3D printing and on the advantages that 3D printing technology combined with biomass-derived materials can bring to modern medicine, such as in tissue engineering, wound healing, and organoids. To reach the target property of 3D-printed biomass-derived materials, it is important to understand their composition and material characteristics. Overall, biomass-derived fiber materials are promising candidates for the commercialization of medical materials, and we expect the points shared in this review to give an impetus to further exploration of biomass-derived fiber materials for biomedical applications.
2 Source and composition of biomass-derived materials
2.1 Polysaccharide fiber materials
Polysaccharides originate from plants, animals, and microorganisms, and are abundant in the biological world with a wide range of functional and structural features. Polysaccharide fiber materials mainly include cellulose, chitin, starch, sodium alginate, pectin, and HA. They have been widely applied in various fields, including food, medicine, pharmaceuticals, energy components, cosmetics, packaging, dyes and pigments, antimicrobials, and water treatment (Akshay Kumar et al., 2021).
2.1.1 Cellulose
Cellulose, the main component of lignocellulosic biomass, is the most abundant natural polymer on earth. The annual global production of cellulose is approximately 75–100 billion tons (Du et al., 2019). Cellulose, a large molecular polysaccharide consisting of glucose, is synthesized in nature as a single molecule with linear chains of glucose-based residues, which self-assemble at biosynthetic sites. Approximately 30 individual cellulose molecules are assembled into fibrils, which are packaged into larger microfibrils, which in turn are assembled into cellulose fibers. The constituent molecules of individual microfibrils are packed tightly to prevent penetration by enzymes and small molecules such as water (Khalid et al., 2017). Therefore, cellulose is insoluble in water and organic solvents. The main sources of cellulose are wood pulp, dissolving wood pulp, cotton, cotton staples, and other fiber pulps. Cellulose derived from plants and bacteria exists in the form of cellulose nanofibers, nanoparticles, hydrogels, and aerogels. Cellulose has attracted increasing attention in the field of biomedical materials owing to its biocompatibility, biodegradability, renewability, environmental friendliness, and non-toxicity.
2.1.2 Chitin and chitosan
Chitin is the second most common polymer on earth after cellulose and is mainly derived from the exoskeletons of insects and marine crustaceans. In its native state, chitin occurs as ordered crystalline microfibers that form structural components in the exoskeletons of arthropods or the cell walls of fungi and yeast. Until now, the main commercial sources of chitin have been crab and shrimp shells. In industrial processes, chitin is extracted by dissolving calcium carbonate with an acid treatment and then dissolving the protein in an alkaline solution. Hence, chitin exhibits a high degree of thermodynamic stability. The poor solubility of chitin in solvent extraction makes the extraction process difficult and laborious (Zhang et al., 2021). With advances in technology and a better understanding of its physiological and biological properties, chitin can be deacetylated and converted into chitosan (CS), a water-soluble polysaccharide (El Knidri et al., 2018). Nanomaterials based on chitin and its derivatives are frequently used in biomedical fields, such as in hemostasis, wound healing, antimicrobial agents, cell culture, tissue regeneration, and skin protection, owing to their unique biocompatibility, biodegradability, non-toxicity, metal ion chelation, and antimicrobial properties (Liu et al., 2019a; Ahmad et al., 2020).
2.1.3 Sodium alginate
Alginate is a biopolymer commonly obtained from brown algae (Cyanobacteria, Alginidae) and bacteria (Azotobacter vineyard and Pseudomonas genus species) and it belongs to the polycationic copolymer family (Ling et al., 2019; Kothale et al., 2020). Alginate is a hydrophilic polysaccharide composed of linear copolymers containing (1,4) -linked β-d-mannouronic acid (M) and α-l-galonic acid (G) residues. The proportion of MM, GG, and MG blocks has a decisive influence on the physical properties of alginate (Sanchez-Ballester et al., 2021). Alginate has been used in the food and cosmetic industries as a thickener or viscosity increaser, owing to its viscosity, biodegradability, and non-toxicity (Kontominas, 2020). Ling et al. reported that alginate-CS microspheres could prevent coated drugs from damaging the reticulum endothelial system (Ling et al., 2019). Moreover, alginate has good biocompatibility, low cost, ease of gelation, inertness, chemical compatibility, derivatives of natural sources, and easily available and feasible synthesis methods with several attractive properties, making it the biopolymer most widely applied in wound dressings, bone regeneration, new angiogenesis, protein delivery, cell delivery, therapeutic agents, oral drug delivery, and controlled release systems (Dhamecha et al., 2019).
2.1.4 Hyaluronic acid
HA, a non-sulfated anionic polysaccharide, is widely present in the human body and is essential for many cellular and tissue functions. HA is a non-sulfated glycosaminoglycan consisting of repeated D-glucuronic acid polymeric disaccharides linked by glycosidic bonds in an arrangement of alternating β-(1→4) and β-(1→3) bonds. The HA structure has a remarkable ability to retain/capture approximately 1000 times its weight in water (Bukhari et al., 2018). HA is a sulfate-free glycosaminoglycan found throughout the body, from the vitreous of the eye to the extracellular stroma of cartilage tissue. HA can be degraded by hyaluronidase in the body; therefore, its half-life is a few hours to days (Burdick and Prestwich, 2011). HA can be modified into HA-derivatives (thiol-modified HA, haloacetate-modified HA, hydrazide-modified HA, aldehyde-modified HA, and aldehyde-modified HA) according to the properties of the resulting material. HA and its derivatives have been used clinically for more than three decades (Peptu and Kowalczuk, 2018). Owing to its abundance in animals and humans, its biodegradability, non-toxicity, biocompatibility, non-immunogenicity, and non-inflammatory properties, it can be used for skin repair, cancer diagnosis, wound healing, tissue regeneration, anti-inflammatory purposes, and immune regulation. (Burdick and Prestwich, 2011; Tiwari and Bahadur, 2019).
2.2 Protein fiber materials
Natural protein fiber materials exhibit enhanced biocompatibility, bioactivity, and biodegradability. Because of their excellent biodegradability and biocompatibility, these proteins (collagen, silk, gelatin, soy protein, keratin, elastin, zein, and soy) are mostly used in the medical field in stents, sutures, wound healing, ligament replacement, and drug delivery. Compared with other natural biomaterials, they can support increased cell migration and proliferation.
2.2.1 Silk fibroin protein
Silk fibroin (SF) is present in the glands of filament-producing arthropods, including silkworms, spiders, scorpions, mites, and bees, and is spun into fibers during metamorphosis. Silk has a large molecular weight (200–350 kDa or higher) and a large repetitive modular hydrophobic domain, which is interrupted by small hydrophilic groups. The N- and C-termini of the SF are highly preserved. The SF protein consists of a heavy chain (H) and a light chain (L) linked by disulfide bonds. The H chain has a hydrophobic domain, and the L chain is hydrophilic and relatively elastic. The ratio of H to L determines the mechanical properties, bioactivity, and degradation behavior of SF (Kundu et al., 2013). The extraction of silk protein usually requires the addition of a variety of concentrated salt solutions, such as lithium bromide, calcium chloride/ethanol/water, lithium chloride, or ionic liquids, under heated conditions. The low immunogenicity and antigenicity of SF rarely cause wound infections. Biodegradability and bio-absorbability materials are promising substitutes for many human protein (Kundu et al., 2013). Degummed filament silk fibers can form a variety of twisted structures, including membranes, hydrogels, 3D porous scaffolds, and particles, for use in vascular, skin, bone, cartilage, ligament, tendon, neural, and cardiac tissue regeneration (Yao et al., 2022).
2.2.2 Collagen and gelatin
Collagen is widely distributed in connective tissues throughout the body. The synthesis of natural collagen is complex and involves several biological processes in vivo. The processing property of collagen is an elegant structural motif in which three parallel polypeptide chains interact with each other in a left-handed polyproline type II (PPII) helical conformation coil, forming a single-residue right-handed triple helix. The abundant amide-amide hydrogen bonds in the triple helix structure give collagen strong thermal stability, mechanical strength, and the ability to interact with other biomolecules, making collagen an important structure for animal scaffolds (Shoulders and Raines, 2009). As a protein from mammals, collagen has advantages, such as an abundant source, rich biocompatibility, easy processing, hydrophilicity, low antigenicity, and being easily absorbable by the body (Sorushanova et al., 2019). Collagen has been used in leather, food, medicine, and photography. In the medical field, collagen scaffolds can serve as artificial skin, bones, tendons, and cartilage. Moreover, collagen can function as a carrier for drugs and growth factors. However, poor physicochemical properties of collagen, such as thermal stability, mechanical strength, and enzyme resistance, are often required to bind other synthetic polymers (polylactic acid) in bone regeneration (Liu X. et al., 2019).
Gelatin is a natural polymer with a molecular weight between 15 and 250 kDa that is hydrolyzed and degraded by collagen. Gelatin can be obtained from cow bones, fish, pig skin, and some insects through alkaline hydrolysis or acid hydrolysis (Campbell and Hotchkiss, 2017). Its unique amino acid structure gives it a variety of medical benefits: (1) It is highly soluble; (2) It contains important binding parts for cell attachment; (3) It is biodegradable and non-toxic to cells. However, for biological materials, gelatin has some drawbacks. The main disadvantages of gelatin-based materials are poor mechanical properties, poor thermal stability, and a relatively short degradation rate (Bello et al., 2020). Gelatin is used in various areas, such as personal care products, pharmaceuticals, photography, nutraceuticals, food and liquid snacks (dessert courses, dairy products, liquid snacks, poultry and meat products, and confectionery) among others (Campbell and Hotchkiss, 2017).
2.2.3 Soy protein
SF, collagen, and gelatin are examples of animal protein used in biomedicine. Soy globulin, the main plant protein, is the main protein in soy. Soy protein has a variety of functional physicochemical properties for food applications, such as emulsification, foaming, gel, water, and fat absorption (Jahangirian et al., 2019). Soy protein isolate is used with other materials as a coating for protection or for physical or chemical surface modification (Hadzieva et al., 2017). In recent years, soy protein has been gradually applied to the medical field, as it can be prepared into hydrogels, micro and nanoparticles, fibers, and porous structures, suitable for different medical applications, such as tissue engineering, drug delivery, etc. Plant-derived proteins have a lower immunogenic potential than animal proteins, are easy to process, are certainly less likely to transmit disease and are relatively inexpensive. In addition, they have relatively low molecular weight and exhibit greater polarity (Mohammadinejad et al., 2016). With the emergence of new technologies and the improvement of the function of these protein materials, there will be more opportunities for effective disease treatment in the future.
3 Biomass-derived fiber materials for biomedical applications
3.1 Antibacterial fiber
CS can resist various microbes, including bacteria, molds, and yeasts. The main reason for this is that the negative surface charge of the bacteria interacts with the positively charged amino group of CS, resulting in a change in cell permeability and disruption of the cell membrane (Matica et al., 2019). Another mechanism is that CS hydrolysis products can interact with microbial DNA, thereby disturbing mRNA, and protein synthesis (Romanazzi et al., 2018). Finally, CS can chelate metal ions (Ni2+, Zn2+, Co2+, Fe2+, and Cu2+), which are essential nutrients for bacteria (Hosseinnejad and Jafari, 2016). CS of different molecular weights have different antibacterial effects. CS with a low molecular weight can pass through the cytomembrane and combine with the cell nucleus. However, CS with a high molecular weight can adhere to bacteria and disrupt nutrient transport (Yuan et al., 2020). Unfortunately, their poor mechanical characteristics and water sensitivity have severely restricted their application.
CS fibers are the most widely used form of CS material to improve their mechanical properties. Currently, CS fibers are mainly fabricated with various polymers, such as PVA, PEO, and PCL, to function as antibacterial agents in wound healing, tissue repair, and regeneration (Guo et al., 2020; Wang et al., 2020). Zhu et al. prepared CS/gallnut tannin fibers via blended solution spinning; the results showed that it significantly improved the lower hydrophilicity and higher dry and wet breaking strengths. In addition, the antimicrobial properties increased from 49% to 99.7% (Zhu et al., 2019). The different bacterial inhibition rates of CS-derived fibers in recent years are displayed in Table 1. Generally, the inhibition efficiency of CS-derived fibers against S. aureus was superior to that of E. coli.
Guo et al. prepared coaxial electrospun nanofibrous mats with CS/PEO as the shell and PCL as the core. After 48 h of incubation, the mats exhibited excellent antibacterial performance against E. coli and S. aureus (Guo et al., 2020). Wang et al. fabricated HKUST-1/CS/PVA fibers for application in wound healing processes against E. coli and S. aureus with 99% antibacterial efficiency (Wang et al., 2020). Antifungal activity against C. albicans, C. glabrata, and S. cerevisiae (Verlee et al., 2017) has been recently discovered. Antifungal properties are related to molecular weight (MW). Low MW (LMW) CS (17.4 kDa) is more likely to inhibit the mycelial growth of R. stolonifera (Hernandez-Lauzardo et al., 2008), whereas high MW (HMW) CS (350 kDa) is more effective for spore germination and mycelial growth of A. kikuchiana (Meng et al., 2010). Compared with the HMW CS, the LWH CS can penetrate the fungal cell wall more easily.
3.2 Skin-wound dressings
Globally, wound dressings, as skin tissue engineering materials, have drawn considerable attention from scientists and clinicians. However, traditional dressings, including gauze, lint, cotton wool, and bandages, are often easily dried and adhere to the skin, causing wound pain and discomfort (Khalid et al., 2017). In addition, conventional dressings often cannot resist bacterial infections and provide sufficient tensile strength. Moreover, their single function in wound healing cannot fulfill the requirements of complex wounds, such as infected wounds and diabetic chronic wounds (Mostafalu et al., 2017; Chen et al., 2018). An ideal wound dressing can: (1) provide a moist environment, (2) remove the exudate, (3) possess air permeability, and (4) possess antibacterial properties. Currently, natural biopolymeric fibers show considerable potential as wound dressing candidates because of their excellent moisture retention, high sorption capacity, outstanding biodegradability, and appropriate mechanical properties (Xi et al., 2018; Homaeigohar and Boccaccini, 2020; Xi et al., 2020). Biomass-derived fibers mainly originate from naturally occurring polymers, such as cellulose, HA, chitin/CS, collagen, and SF.
Cellulose has been extensively investigated for tissue regeneration by mimicking the extracellular matrix (ECM) structure. Compared with commercial wound dressings, cellulose-fiber dressings are equipped with more advantages, including (1) maintaining a moist environment at the wound site to relieve pain; (2) promoting granulation tissues and re-epithelialization formation, and diminishing scar formation; (3) being equipped with good molding and mechanical properties to meet the requirements of complex wound healing (burns and chronic ulcers, damaged tissues, etc.); (4) protection of the wound against bacterial infection (Luo et al., 2021); and (5) excellent light transmittance and high porosity to speed up wound healing (Xia et al., 2020). Cellulose-fiber dressings can be multi-functionalized by incorporating other biopolymers, such as enzymes, collagen, CS, hormones, and gallic acid (Li et al., 2019; Wutticharoenmongkol et al., 2019). For instance, Xia et al. fabricated transparent wound dressings using porous cellulose fibers coated with CS, enabling visualization of wound healing (Xia et al., 2020). However, the special functions of cellulose fibers at different stages of wound healing, such as inflammation, proliferation, and remodeling, should be further investigated in subsequent studies. Hamid et al. prepared PVA/CS/silk sericin/tetracycline porous fibers possessing hygroscopic properties, antibacterial activity, and biocompatibility in vivo and in vitro (Bakhsheshi-Rad et al., 2020).
HA is a promising biopolymer for biomedical applications, especially wound dressings. The rich carboxyl and hydroxyl groups of the HA structure provide it with a highly hydrophilic character that facilitates cell adhesion and wound healing. Moreover, HA can encourage hemostasis, prompt collagen deposition and fibrosis, regulate inflammation balance, and promote re-epithelialization via the enzymatic reaction of hyaluronidase and chemical interaction (Zhu et al., 2017; Xia et al., 2020). The pivotal role of HA-fiber dressing in wound healing is in the inflammation phase (Graca et al., 2020). HA can facilitate the recruitment of neutrophils to eliminate debris and dead tissues and subsequently release cytokines, such as TNF-a, IL-1b, and IL-8, which are involved in the inflammatory response (Tavianatou et al., 2019). HA with a high molecular weight (HMW-HA, >1 × 106 Da) is fragmented to low molecular weight (LMW-HA, 1–25 × 104 Da), recruiting leukocytes and monocytes into the wound site. Finally, the cell surface receptors (TLR2 and TLR4) of these cells interact with LMW-HA to promote the expression of these cytokines, further cascading amplification effects (Zamboni et al., 2018; Tavianatou et al., 2019). However, the mechanical stability of HA-fiber dressings is a significant challenge. To date, the characteristics of HA-fiber dressings have been constantly developed through the incorporation of biomacromolecules and chemical modification to be widely applied in the clinic, for example, the HylaSponge® System (Mahedia et al., 2016), Hyalomatrix® (Longinotti, 2014), and Hyalosafe® (Longinotti, 2014). Moreover, HA-fibers advance into differently structured dressings (sponges, films, hydrogels, and electrospun membranes) with natural and synthetic biomolecules, such as PCL, PLGA, collagen, SF, and CS (Zhou et al., 2016; Graca et al., 2020). In the future, HA-fiber dressings with multiple functions will likely be developed.
Chitin is often insoluble in common solvents; thus, it is usually deacetylated to CS in an alkaline environment by dissolving it in organic acids, such as dilute aqueous formic, acetic, and lactic acids. In the early stages of wound healing, CS can induce polymorphonuclear neutrophils to migrate to the wound area, leading to the formation of granulation tissues. In the late phase of the wound healing process, CS can promote the re-epithelialization and regeneration of the granular layer of skin, facilitating collagen synthesis and fibrosis formation (Alven and Aderibigbe, 2020). In addition, positive results of CS on the activation of microphases have been reported (Simoes et al., 2018). One amino group and two hydroxyl groups of CS can interact with different materials, including polyethylene oxide, PVA, and collagen, to fabricate nanofibers, nanoparticles, sponges, and hydrogels. Among these forms, CS-fiber is the major form because of its easy formability and appropriate mechanistic properties.
Collagen possesses excellent biocompatibility, which promotes human keratinocyte and epidermal cell proliferation, differentiation, and migration, thus favoring the recovery of skin wounds. However, the drawback of collagen-fiber dressing is that it is often derived from animal sources (bovine, porcine, and avian) and may stimulate an immunological response and transfer pathogens to the host tissues. In addition, the stability of the poll mechanism and the fast degradation rate of collagen are the challenges of bio-utilization in vivo (Alven and Aderibigbe, 2020). More importantly, the difficulty in collagen extraction and purification limits its wide application.
SF has drawn great attention from scientists and clinicians owing to its abundance, low immunogenicity, slow degradation, high water content, and oxygen uptake. Moreover, good mechanical properties, such as breaking elongation (15%) and elasticity (>30%), are beneficial for elastic fibers in vivo (Mehrotra et al., 2019). The high solubility of SF makes it suitable for processing different structures, including electrospun nanofibers, films, hydrogels, and sponges (Yuan et al., 2020). Evidence has shown that the arginine-glycine-aspartic acid motifs of SF can alleviate attachment to the integrin receptor, thus accelerating the wound-healing process (Gupta et al., 2015). At present, SF optimally reduces the time of wound recovery, minimizes the formation of scarring, and alleviates atopic dermatitis in vitro and in vivo, laying the foundation for SF as a promising alternative option for wound dressing (Tu et al., 2019). More detailed information of SF in wound dressings can be found in a published review (Farokhi et al., 2018). Biomass materials, such as cellulose, HA, chitin, collagen, and SF, can be fabricated into nanofibers and hydrogels by fusing other polymer materials, and can be effectively applied to skin-wound repair (Figure 1).
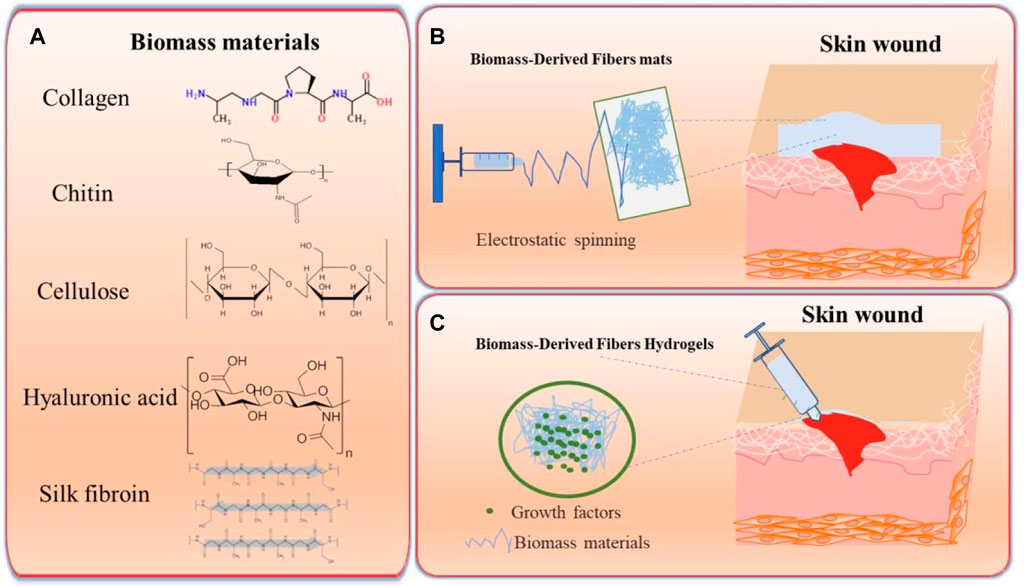
FIGURE 1. Schema of biomass-derived fibers for skin-wound dressings. (A) Biomass material and their corresponding structural formulas. (B) Schema of Biomass-derived fiber mats via electrostatic spinning used for the repair of skin wounds. (C) Schema of Biomass-derived fiber hydrogels for the repair of skin wounds.
3.3 Tissue engineering
Bone and cartilage tissue compose the skeleton and provide protection for the whole body. Currently, the main therapeutic methods for bone defects are autogenous and allogenous bone transplantation and metal implants. However, limited donor tissues, potential infection, and immune rejection rate sharply restrict the implications of traditional grafts. Rapid developments in tissue engineering and regenerative medicine have made bone defect repair and regeneration possible. An ideal bone repair scaffold should have the following characteristics: (1) stable mechanical properties, (2) excellent biocompatibility and degradability, (3) beneficial for the delivery of osteoprogenitor cells or/and growth factors (Bhattacharjee et al., 2017); and (4) appropriate porosity (100–710 μm) (Przekora, 2019).
Bone is mainly composed of macromolecules, such as collagen, osteocalcin, osteonectin, hyaluronan, and proteoglycans, and of hydroxyapatite (Roseti et al., 2017). Biopolymeric nanofibers can mimic the bone ECM, where the cell can interact with the nanostructure surface of the material and rapidly grow. Chitin and CS, similar to the ECM, can be degraded into small molecular amino acids and polysaccharides in vivo, and have been processed into different structures, such as nanofibers, hydrogels, and aerogels for application in bone tissue repair and regeneration. Chitin/CS-fiber scaffolds and calcium phosphate/chitin hydrogels have been shown to facilitate the repair of bone defects without an inflammatory response in vivo (Gupta et al., 2015). Moreover, Chitin and CS can be dissolved in acidic solvents with other polymers, such as PCL, PLA, and nylon 6, to change their mechanical performance and biological properties. Research has shown that the function mechanism of chitin/CS is that it can activate osteogenesis-related signaling pathways, Runx2, BMP-2, BMP-4, and collagen-1, and facilitate the expression of alkaline phosphatase (ALP) (early biomineralization marker) and osteointegration relative protein, which contributes to mineralization and bone regeneration (Liu et al., 2013). Overall, chitin/CS-fiber scaffolds mainly affect bone regeneration engineering by promoting cell growth and osteogenic differentiation, accelerating angiogenesis of mineralized bone tissue, and promoting drug delivery. Apart from Chitin and CS, alginate has shown great potential in bone tissue engineering owing to its good biocompatibility, biodegradation, and size handling. In addition, alginate nanofiber blended with calcium phosphate cement can activate osteoblastic cell immobilization, proliferation, and differentiation (Jayachandran et al., 2022). Alginate scaffolds can promote human umbilical cord mesenchymal stem cell differentiation, and express ALP and osteosarcoma-associated proteins (Su et al., 2021). Alginate saline gel has strong gelling ability, low toxicity, high availability, and low cost, characteristics that make it the most common material in bone tissue engineering and bio-printing.
Proteins with non-toxicity, biodegradability, and biocompatibility make them an alternative to bone, ligaments, and cartilage. Owing to swelling in vivo, collagen must cross-link with other polymers (CaPs) to overcome its disadvantages and poor mechanical and dimensional stability (Bao et al., 2020). The nature of the robust elastic property of SF protein corresponds with the robust mechanical strength of the bone. A group of SF surfaces, such as -OH, -COOH, and -N-H, can provide sites for hydroxyapatite (Kundu et al., 2012), and then the hydroxyapatite is mineralized to form bone under certain conditions. Typically, SF can guide calcium phosphate formation and promote the deposition of osteoblasts along nanofibers to produce a new matrix. More importantly, the degradation rate of SF is 6–12 months in vivo, which is helpful for the repair of bone defects, and the degradation products of SF (glycine and alanine) can be reused as templates for neo-protein synthesis. Therefore, SF does not often stimulate excessive inflammatory reactions. Moreover, SF-chitin hybrid nanofiber/TGF-β1-functionalized scaffolds displayed a significant effect on chondrocyte proliferation, difference, and adhesion in vivo and in vitro (Cheng et al., 2021). Cheng et al developed core-shell SF/PCL/PVA mats by loading a controlled release system of BMP2 and CTGF using layer-by-layer techniques. Its positive effects on bone tissue repair and healing were confirmed in vitro and in vivo (Cheng et al., 2019). SF has been manufactured in a variety of forms, including films, artificial fibers, sponges, and hydrogels, which have been successfully deployed in a variety of tissue engineering applications (Sun et al., 2021). In the future, the fabrication by 3D bioprinting of nanoscale SF multi-level structures with high structural resolutions will make the application of SF more popular.
3.4 Drug delivery
Cancer, as one of the world’s biggest challenges, causes enormous medical and financial burdens. Traditionally, cancer therapy has included surgery, radiation, and chemotherapy. However, these treatments can easily cause systemic adverse reactions while killing the tumors. Progress has been made through biomaterial-based implantable drug delivery in cancer therapy (Yadav et al., 2021). The ideal drug delivery includes the following features: (1) local drug delivery (the drug is released directly into the tumor, thus sharply reducing the drug dose and side effects of the drug); (2) sustained drug release (the drug should avoid abrupt drug release and maintain function concentration for a longer period); and (3) drug stability (the drug-delivery system should preserve the loaded drug to avoid degradation and removal by enzymes or other media in vivo before it is released) (Bastiancich et al., 2016; Conde et al., 2016; Talebian et al., 2018).
Cellulose is a natural and promising material in the biomedical field owing to its availability and good mechanical properties. Its notable mechanical characteristics, water solubility, hydrolytic stability, and natural biocompatibility facilitate its biomedical application. It has been produced on electrospun fibers coated with four model drugs (naproxen, indomethacin, ibuprofen, and sulindac) (Tungprapa et al., 2007). However, this treatment has the risk of causing initial burst release; thus, different modified strategies have been developed, including the application of tri-axial electrospinning (Yang et al., 2019), increasing the surface hydrophobicity of drug carriers, and increasing porosity (Chen et al., 2020). Cellulose sulfate is an ester or cellulose sulfated from cellulose. Compared to natural cellulose, its antimicrobial properties and solubility are greatly improved. In addition, the excellent film-forming property allows it to effectively encapsulate drugs (rifampicin and risedronate) (Vehlow et al., 2016) and cytokines, such as BMP2 (Muller et al., 2018). Cellulose nitrate, acetate cellulose (Chen et al., 2020), carboxymethyl cellulose (Chen et al., 2019), ethyl cellulose, and methyl cellulose are effective carriers for drug delivery (Oprea and Voicu, 2020). Schematics of biomass-derived fibers for drug delivery are shown in Figure 2.
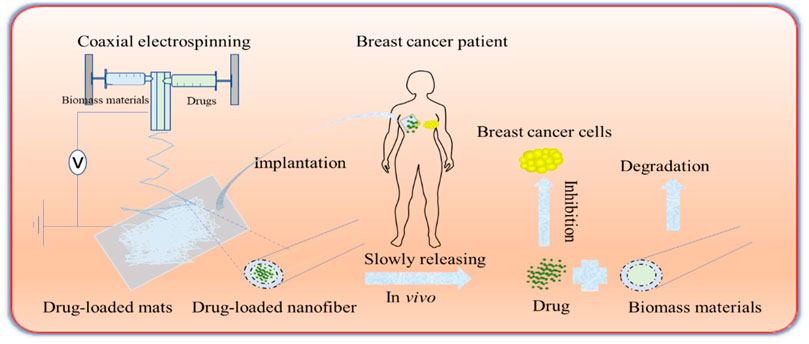
FIGURE 2. Schema of biomass-derived fibers for drug delivery. The drugs and biomass materials are made into porous nanofiber mats by coaxial electrospinning. The mats are transferred into the tumor areas of patients with breast cancer, etc. The drugs are released gradually, and the drugs inhibit tumor growth, while the biomass materials can be degraded naturally.
4 3D printing of biomass-derived fiber materials
4.1 From material bionics to structure bionics
Ideal medical biomimicry materials model the complexity and heterogeneity of these tissues, including the required biological functions, sufficient strength, and stiffness to maintain structural integrity, and microscopic porous structures. But, common nanofibers via traditional process methods, such as electrostatic spinning, chemical vapor deposition, electrospinning, and solution blowing, can only still create biomaterials for tissues with relatively simple structures and compositions, such as skin and bone. However, the fine structure and complex composition of organisms are far beyond the capabilities of manufacturing technology, which limits biomimetic research on tissue regeneration. 3D printing has shown great promise in the design of biomaterials with multiscale, multilateral, and multifunctional structures (Ashammakhi et al., 2019; Ng et al., 2019). According to statistics, the proportion of biomass materials (∼85%) in 3D printing is much higher than that of polymer materials (Khoeini et al., 2021) (Figure 3). Collagen, accounting for 26%, is the most common 3D-printed biomass material, which represents the major components of our natural ECM, providing overall organizational stiffness and integrity with distinct properties, and functionalities (Lee C et al., 2021). Alginate (24%) is the second most used biomass material in bioinks, which can improve the quality of the built (reduced spattering) or printed entity and the viscosity of the ink (Rastogi and Kandasubramanian, 2019). HA (11%) stands out for its excellent physical, chemical, and biological properties, which can provide printing suitability, improve its mechanical properties and printing with loaded cells, etc. (Ding et al., 2023). With this technology, biomass-derived materials have been successfully made into fibers, channels, sheets, coils, meshes, and porous 3D constructs that mimic tissue components. Rees A et al. discovered that, via 3D bioprinting, CS was formed into a 3D scaffold of grid structure with 9 layers, which has open porosity and the potential to carry and release antimicrobial components (Rees et al., 2015). Su H et al. fabricated free-standing multifurcated vessels and complicated vascular networks in heterogeneous porous scaffolds by 3D printing technology (Su et al., 2022). Gupta D et al. prepared a 3D printed gelatin/carboxymethyl chitin/hydroxyapatite composite bioactive gel scaffold with a controlled hierarchical structure (Gupta et al., 2019).
4.2 Advantages of 3D printing of biomass-derived composite fiber
Biomass-derived materials, including cellulose, lignin, starch, collagen, alginate, and CS, are the most used materials for 3D printing. They not only meet the needs of sustainability, but also reduce the side effects of some synthetic polymers in biomedical applications, e.g., degradability, recyclability, harmful decomposition of products, release of additives, and reduced cell attachment (Liu et al., 2019b). However, the inherent physicochemical properties of some biomass-derived materials, such as poor stability and adhesion, certainly pose difficult challenges to their 3D printing application. Hence, mixing different types of biopolymers with complementary advantages or combining with other ingredients are alternative methods or strategies to formulate composite inks with good processability, printability, mechanics, and bioactivity, and the performance and function of biomaterial products can also be improved and biomass-derived applications can be broadened. For instance, by combining agarose and type I collagen, Kopf, M. et al. developed a hydrogel mixture capable of coating human umbilic artery smooth muscle cells for a long time and that could be 3D printed on demand (Kopf et al., 2016). 3D structure-scaffolds can be prepared by adding methylcellulose into a 3% alginate solution, providing better printability, and maintaining the advantage for cell embedding (Schutz et al., 2017). 3D bioprinters can distribute materials while moving in the X, Y, and Z directions, which can prepare complex cartilage structures, such as a human ear and sheep meniscus, with high shape fidelity and printing resolution (Markstedt et al., 2015). Stiglic et al. developed an organic acid cross-linked 3D-printed cellulose nanocomposite biological scaffold with controllable porosity, mechanical strength, and biocompatibility (Stiglic et al., 2022).
4.3 3D printed polysaccharide/protein fiber materials in medicine application
Biomass-derived materials that are 3D printed can be applied in bone/articular cartilage defect repair, nerve tissue repair, soft tissue repair, and other types of repair. Ventola et al. incorporated human nasal septum chondrocytes into cellulose-alginate composite ink for 3D bioprinting to improve the shape fidelity and stability of biomedical materials (Ventola, 2014). Jiang et al. developed multilayer 3D printed scaffolds for rotator cuff tendon regeneration via combined collagen-fibrin hydrogels (Jiang et al., 2020) (Figure 4). Yao M et al. prepared HA, poly (dopamine), and carboxymethyl CS composite scaffolds using 3D printing technology, which had an anti-osteosarcoma effect and bone repair properties (Yao et al., 2021). Liu H et al. used 3D printing technology to develop SF/hydroxyapatite/collagen scaffolds with ideal water absorption and porosity, which could significantly promote the reconstruction of mandibular defects (Liu et al., 2022). Those 3D-printed polysaccharide/protein fiber materials possess high mechanical properties and biocompatibility, which can be widely applied in bone tissue engineering. Recent polysaccharide/protein fiber-based 3D printing studies with various applications regarding the different types of biomass fibers are summarized in Table 2; Table 3.
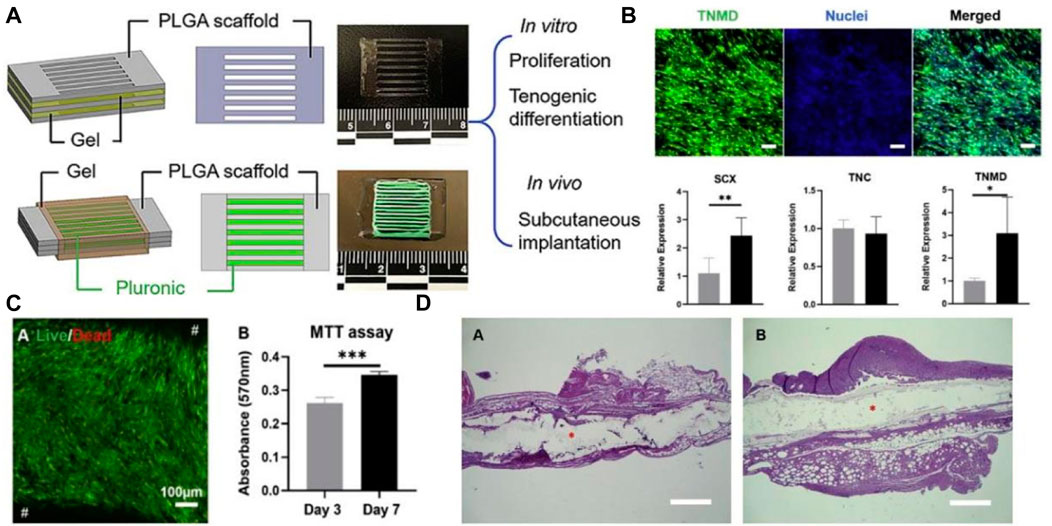
FIGURE 4. (A) Schema of PLGA scaffold models with collagen-fibrin hydrogel. (B) collagen fibrin hydrogels induce cell differentiation; (C) Effect of collagen fibrin hydrogels on cell activity and proliferation; (D) Post-implantation H&E staining of collagen fibrin hydrogels. Reproduced with permission (Jiang et al., 2020). Copyright 2020, Elsevier.
5 Conclusion and outlook
With the development of interdisciplinary research between medicine and bioengineering, emerging research has been devoted to the application of biomass materials in medicine. Combining the clinical needs and physical characteristics of biomass-derived fiber materials, the development of excellent and novel medical materials is promising for application in a wider range of fields. In general, most biomass-derived fiber materials contain polysaccharide and protein fibers from two major sources, which offer excellent antibacterial properties and a significant recovery function of tissues that contribute to the development of antibacterial/antibiofilm materials, skin-wound dressings, tissue engineering, drug-sustained release, and other areas. Furthermore, the multiscale, multilateral, and multifunctional structures of biomass-derived fiber materials are available by 3D printing technology, which has been recognized as an effective and promising method for preparing biomass-derived fibers, owing to its advantages in speed, accuracy, and flexibility (Ji et al., 2020).
Meanwhile, 3D printing of biomass-derived fiber materials is also a promising prospect for drug detection. In past decades, drug screening and the development of new drugs have often relied on genetically modified animals as disease models. However, the use of animal models raises serious ethical issues and brings with it inevitable limitations in accurately representing human tissues in the pathophysiological context due to genetic differences (Vanderburgh et al., 2017). 3D printing of biomass-derived materials can provide platforms for drug delivery, screening, and development by accurately depositing biomaterials containing patient-derived cells and simulating the natural environment of the diseased body. Falcone G et al. prepared floating ricobendazole delivery systems, which can prolong gastric retention times (Falcone et al., 2022). Li Q et al. developed novel gastro-floating tablets with 3D extrusion-based printing, prolonging the gastric residence time of dipsin and increasing the drug release rate (Li et al., 2018). As for floating drug delivery systems, 3D printing of biomass-derived materials can highly prolong the drug action time, thereby improving bioavailability, patient compliance, and therapeutic efficacy. In addition, 3D printing of biomass-derived materials can also serve as the platform for drug screening and development. Yi et al. prepared a radial oxygen gradient using a 3D gas permeation barrier containing ECM (glycosaminoglycan and HA) of brain cancer cells and endothelial cells to generate central hypoxia and simulate an anoxic environment for drug resistance research (Yi et al., 2019). Clark C et al. prepared hyaluronan and collagen bioink-supported 3D patient-derived brain tumor organoids, which can provide viable and accurate organoids for drug screening and development studies and precision medicine applications (Clark et al., 2022). Although 3D printing of biomass-derived materials has great advantages in drug delivery, screening, and development, its long-term effects and biosafety in vivo are still uncertain, and, thus, it needs more clinical studies in the future.
In conclusion, we summarized the common applications of biomass and its derived fibers in the medical field in recent years and discussed the application and advantages of 3D printing technology combined with biomass-derived materials in modern medicine. Our review may benefit from a better understanding of practical applications in biomass-derived fiber materials. Overall, although there have been several successes in vitro and in vivo trials of biomaterials, none have been processed to the human testing stage. Therefore, the translational benefits of biomass-based tissue engineering are still far from being applied to patients. This applies not only to tissue engineering, drug development, and organoids but also to other biomedicine fields. Therefore, translational research is a challenge to overcome in the future.
Author contributions
DL wrote the initial manuscript and created the figures and table. YW conceptualized and discussed the review. HG and WH reviewed and edited the manuscript. All authors have contributed to the work and approved the final version of the manuscript.
Funding
This work was financially supported by the National Natural Science Foundation of China (Grant No. 31972915, 32271181), the Characteristic Innovation Projects of Ordinary Universities in Guangdong Province (Grant No. 2020KTSCX040), the Guangdong Basic and Applied Basic Research Foundation (Grant No. 2020B1515120001), and the Discipline construction project of Guangdong Medical University (G622280009, 4SG22260G). The authors would like to acknowledge those who contributed to this article.
Conflict of interest
The authors declare that the research was conducted in the absence of any commercial or financial relationships that could be construed as a potential conflict of interest.
Publisher’s note
All claims expressed in this article are solely those of the authors and do not necessarily represent those of their affiliated organizations, or those of the publisher, the editors and the reviewers. Any product that may be evaluated in this article, or claim that may be made by its manufacturer, is not guaranteed or endorsed by the publisher.
References
Ahmad, S. I., Ahmad, R., Khan, M. S., Kant, R., Shahid, S., Gautam, L., et al. (2020). Chitin and its derivatives: Structural properties and biomedical applications. Int. J. Biol. Macromol. 164, 526–539. doi:10.1016/j.ijbiomac.2020.07.098
Akshay Kumar, K. P., Zare, E. N., Torres-Mendieta, R., Waclawek, S., Makvandi, P., Cernik, M., et al. (2021). Electrospun fibers based on botanical, seaweed, microbial, and animal sourced biomacromolecules and their multidimensional applications. Int. J. Biol. Macromol. 171, 130–149. doi:10.1016/j.ijbiomac.2020.12.205
Alven, S., and Aderibigbe, B. A. (2020). Chitosan and cellulose-based hydrogels for wound management. Int. J. Mol. Sci. 21 (24), 9656. doi:10.3390/ijms21249656
Apelgren, P., Amoroso, M., Saljo, K., Lindahl, A., Brantsing, C., Stridh Orrhult, L., et al. (2021). Long-term in vivo integrity and safety of 3D-bioprinted cartilaginous constructs. J. Biomed. Mater Res. B Appl. Biomater. 109 (1), 126–136. doi:10.1002/jbm.b.34687
Ashammakhi, N., Ahadian, S., Xu, C., Montazerian, H., Ko, H., Nasiri, R., et al. (2019). Bioinks and bioprinting technologies to make heterogeneous and biomimetic tissue constructs. Mater. Today Bio 1, 100008. doi:10.1016/j.mtbio.2019.100008
Augustine, R., Rehman, S. R. U., Ahmed, R., Zahid, A. A., Sharifi, M., Falahati, M., et al. (2020). Electrospun chitosan membranes containing bioactive and therapeutic agents for enhanced wound healing. Int. J. Biol. Macromol. 156, 153–170. doi:10.1016/j.ijbiomac.2020.03.207
Bakhsheshi-Rad, H. R., Ismail, A. F., Aziz, M., Akbari, M., Hadisi, Z., Omidi, M., et al. (2020). Development of the PVA/CS nanofibers containing silk protein sericin as a wound dressing: In vitro and in vivo assessment. Int. J. Biol. Macromol. 149, 513–521. doi:10.1016/j.ijbiomac.2020.01.139
Bao, W., Li, M., Yang, Y., Wan, Y., Wang, X., Bi, N., et al. (2020). Advancements and Frontiers in the high performance of natural hydrogels for cartilage tissue engineering. Front. Chem. 8, 53. doi:10.3389/fchem.2020.00053
Bao, Y., Zhou, Q., Zhang, M., Zhang, H., Luan, Q., Zhou, W., et al. (2019). Wet-spun nanoTiO2/chitosan nanocomposite fibers as efficient and retrievable absorbent for the removal of free fatty acids from edible oil. Carbohydr. Polym. 210, 119–126. doi:10.1016/j.carbpol.2019.01.035
Bastiancich, C., Danhier, P., Preat, V., and Danhier, F. (2016). Anticancer drug-loaded hydrogels as drug delivery systems for the local treatment of glioblastoma. J. Control Release 243, 29–42. doi:10.1016/j.jconrel.2016.09.034
Bello, A. B., Kim, D., Kim, D., Park, H., and Lee, S. H. (2020). Engineering and functionalization of gelatin biomaterials: From cell culture to medical applications. Tissue Eng. Part B Rev. 26 (2), 164–180. doi:10.1089/ten.TEB.2019.0256
Bhattacharjee, P., Kundu, B., Naskar, D., Kim, H. W., Maiti, T. K., Bhattacharya, D., et al. (2017). Silk scaffolds in bone tissue engineering: An overview. Acta Biomater. 63, 1–17. doi:10.1016/j.actbio.2017.09.027
Bukhari, S. N. A., Roswandi, N. L., Waqas, M., Habib, H., Hussain, F., Khan, S., et al. (2018). Hyaluronic acid, a promising skin rejuvenating biomedicine: A review of recent updates and pre-clinical and clinical investigations on cosmetic and nutricosmetic effects. Int. J. Biol. Macromol. 120, 1682–1695. doi:10.1016/j.ijbiomac.2018.09.188
Burdick, J. A., and Prestwich, G. D. (2011). Hyaluronic acid hydrogels for biomedical applications. Adv. Mater 23 (12), H41–H56. doi:10.1002/adma.201003963
Campbell, R., and Hotchkiss, S. (2017). “Carrageenan industry market overview,” in Tropical seaweed farming trends, problems and opportunities (Berlin, Germany: Springer), 193–205.
Chen, G., Yu, Y., Wu, X., Wang, G., Ren, J., and Zhao, Y. (2018). Bioinspired multifunctional hybrid hydrogel promotes wound healing. Adv. Funct. Mater. 28 (33), 1801386. doi:10.1002/adfm.201801386
Chen, J., Duan, H., Pan, H., Yang, X., and Pan, W. (2019). Two types of core/shell fibers based on carboxymethyl chitosan and Sodium carboxymethyl cellulose with self-assembled liposome for buccal delivery of carvedilol across TR146 cell culture and porcine buccal mucosa. Int. J. Biol. Macromol. 128, 700–709. doi:10.1016/j.ijbiomac.2019.01.143
Chen, Y., Qiu, Y., Chen, W., and Wei, Q. (2020). Electrospun thymol-loaded porous cellulose acetate fibers with potential biomedical applications. Mater Sci. Eng. C Mater Biol. Appl. 109, 110536. doi:10.1016/j.msec.2019.110536
Cheng, G., Dai, J., Dai, J., Wang, H., Li, Z., liu, Y., et al. (2021). Extracellular matrix imitation utilizing nanofibers-embedded biomimetic scaffolds for facilitating cartilage regeneration. Chem. Eng. J. 410 (2), 128379. doi:10.1016/j.cej.2020.128379
Cheng, G., Yin, C., Tu, H., Jiang, S., Wang, Q., Zhou, X., et al. (2019). Controlled Co-delivery of growth factors through layer-by-layer assembly of core-shell nanofibers for improving bone regeneration. ACS Nano 13 (6), 6372–6382. doi:10.1021/acsnano.8b06032
Clark, C., Yoo, K. M., Sivakumar, H., Strumpf, K., Laxton, A., Tatter, S. B., et al. (2022). Immersion bioprinting of hyaluronan and collagen bioink-supported 3D patient-derived brain tumor organoids. Biomed. Mater 18, 015014. doi:10.1088/1748-605X/aca05d
Conde, J., Shomron, N., and Artzi, N. (2016). Biomaterials for abrogating metastasis: Bridging the gap between basic and translational research. Adv. Healthc. Mater 5 (18), 2312–2319. doi:10.1002/adhm.201600414
Dhamecha, D., Movsas, R., Sano, U., and Menon, J. U. (2019). Applications of alginate microspheres in therapeutics delivery and cell culture: Past, present and future. Int. J. Pharm. 569, 118627. doi:10.1016/j.ijpharm.2019.118627
Ding, Y.-W., Zhang, X.-W., Mi, C.-H., Qi, X.-Y., Zhou, J., and Wei, D.-X. (2023). Recent advances in hyaluronic acid-based hydrogels for 3D bioprinting in tissue engineering applications. Smart Mater. Med. 4, 59–68. doi:10.1016/j.smaim.2022.07.003
Du, H., Liu, W., Zhang, M., Si, C., Zhang, X., and Li, B. (2019). Cellulose nanocrystals and cellulose nanofibrils based hydrogels for biomedical applications. Carbohydr. Polym. 209, 130–144. doi:10.1016/j.carbpol.2019.01.020
Dumont, M., Villet, R., Guirand, M., Montembault, A., Delair, T., Lack, S., et al. (2018). Processing and antibacterial properties of chitosan-coated alginate fibers. Carbohydr. Polym. 190, 31–42. doi:10.1016/j.carbpol.2017.11.088
El Knidri, H., Belaabed, R., Addaou, A., Laajeb, A., and Lahsini, A. (2018). Extraction, chemical modification and characterization of chitin and chitosan. Int. J. Biol. Macromol. 120, 1181–1189. doi:10.1016/j.ijbiomac.2018.08.139
Falcone, G., Real, J. P., Palma, S. D., Aquino, R. P., Del Gaudio, P., Garofalo, E., et al. (2022). Floating ricobendazole delivery systems: A 3D printing method by Co-extrusion of sodium alginate and calcium chloride. Int. J. Mol. Sci. 23 (3), 1280. doi:10.3390/ijms23031280
Farokhi, M., Mottaghitalab, F., Fatahi, Y., Khademhosseini, A., and Kaplan, D. L. (2018). Overview of silk fibroin use in wound dressings. Trends Biotechnol. 36 (9), 907–922. doi:10.1016/j.tibtech.2018.04.004
Graca, M. F. P., Miguel, S. P., Cabral, C. S. D., and Correia, I. J. (2020). Hyaluronic acid-based wound dressings: A review. Carbohydr. Polym. 241, 116364. doi:10.1016/j.carbpol.2020.116364
Guo, H., Tan, S., Gao, J., and Wang, L. (2020). Sequential release of drugs form a dual-delivery system based on pH-responsive nanofibrous mats towards wound care. J. Mater Chem. B 8 (8), 1759–1770. doi:10.1039/c9tb02522g
Gupta, A. K., Mita, K., Arunkumar, K. P., and Nagaraju, J. (2015). Molecular architecture of silk fibroin of Indian golden silkmoth, Antheraea assama. Sci. Rep. 5, 12706. doi:10.1038/srep12706
Gupta, D., Singh, A. K., Dravid, A., and Bellare, J. (2019). Multiscale porosity in compressible cryogenically 3D printed gels for bone tissue engineering. ACS Appl. Mater Interfaces 11 (22), 20437–20452. doi:10.1021/acsami.9b05460
Hadzieva, J., Mladenovska, K., Crcarevska, M. S., Dodov, M. G., Dimchevska, S., Geskovski, N., et al. (2017). Lactobacillus casei encapsulated in soy protein isolate and alginate microparticles prepared by spray drying. Food Technol. Biotechnol. 55 (2), 173–186. doi:10.17113/ftb.55.02.17.4991
Hassan, M. M., and Carr, C. M. (2021). Biomass-derived porous carbonaceous materials and their composites as adsorbents for cationic and anionic dyes: A review. Chemosphere 265, 129087. doi:10.1016/j.chemosphere.2020.129087
Hernandez-Lauzardo, A. N., Bautista-Banos, S., Velazquez-Del Valle, M. G., Mendez-Montealvo, M. G., Sanchez-Rivera, M. M., and Bello-Perez, L. A. (2008). Antifungal effects of chitosan with different molecular weights on in vitro development of Rhizopus stolonifer (Ehrenb.:Fr.) Vuill. Carbohydr. Polym. 73 (4), 541–547. doi:10.1016/j.carbpol.2007.12.020
Homaeigohar, S., and Boccaccini, A. R. (2020). Antibacterial biohybrid nanofibers for wound dressings. Acta Biomater. 107, 25–49. doi:10.1016/j.actbio.2020.02.022
Hosseinnejad, M., and Jafari, S. M. (2016). Evaluation of different factors affecting antimicrobial properties of chitosan. Int. J. Biol. Macromol. 85, 467–475. doi:10.1016/j.ijbiomac.2016.01.022
Huang, J., Cheng, Y., Wu, Y., Shi, X., Du, Y., and Deng, H. (2019). Chitosan/tannic acid bilayers layer-by-layer deposited cellulose nanofibrous mats for antibacterial application. Int. J. Biol. Macromol. 139, 191–198. doi:10.1016/j.ijbiomac.2019.07.185
Huang, L., Du, X., Fan, S., Yang, G., Shao, H., Li, D., et al. (2019). Bacterial cellulose nanofibers promote stress and fidelity of 3D-printed silk based hydrogel scaffold with hierarchical pores. Carbohydr. Polym. 221, 146–156. doi:10.1016/j.carbpol.2019.05.080
Huang, L., Yuan, W., Hong, Y., Fan, S., Yao, X., Ren, T., et al. (2021). 3D printed hydrogels with oxidized cellulose nanofibers and silk fibroin for the proliferation of lung epithelial stem cells. Cellul. (Lond). 28 (1), 241–257. doi:10.1007/s10570-020-03526-7
Huang, W., Wang, Y., Huang, Z., Wang, X., Chen, L., Zhang, Y., et al. (2018). On-demand dissolvable self-healing hydrogel based on carboxymethyl chitosan and cellulose nanocrystal for deep partial thickness burn wound healing. ACS Appl. Mater Interfaces 10 (48), 41076–41088. doi:10.1021/acsami.8b14526
Jahangirian, H., Azizi, S., Rafiee-Moghaddam, R., Baratvand, B., and Webster, T. J. (2019). Status of plant protein-based green scaffolds for regenerative medicine applications. Biomolecules 9 (10), 619. doi:10.3390/biom9100619
Jayachandran, V., Murugan, S. S., Dalavi, P. A., Gurushanthappa Vishalakshi, Y. D., and Seong, G. H. (2022). Alginate-based composite microspheres: Preparations and applications for bone tissue engineering. Curr. Pharm. Des. 28 (13), 1067–1081. doi:10.2174/1381612828666220518142911
Ji, A., Zhang, S., Bhagia, S., Yoo, C. G., and Ragauskas, A. J. (2020). 3D printing of biomass-derived composites: Application and characterization approaches. RSC Adv. 10 (37), 21698–21723. doi:10.1039/d0ra03620j
Jiang, X., Wu, S., Kuss, M., Kong, Y., Shi, W., Streubel, P. N., et al. (2020). 3D printing of multilayered scaffolds for rotator cuff tendon regeneration. Bioact. Mater 5 (3), 636–643. doi:10.1016/j.bioactmat.2020.04.017
Kawata, M., Azuma, K., Izawa, H., Morimoto, M., Saimoto, H., and Ifuku, S. (2016). Biomineralization of calcium phosphate crystals on chitin nanofiber hydrogel for bone regeneration material. Carbohydr. Polym. 136, 964–969. doi:10.1016/j.carbpol.2015.10.009
Khalid, A., Khan, R., Ul-Islam, M., Khan, T., and Wahid, F. (2017). Bacterial cellulose-zinc oxide nanocomposites as a novel dressing system for burn wounds. Carbohydr. Polym. 164, 214–221. doi:10.1016/j.carbpol.2017.01.061
Khoeini, R., Nosrati, H., Akbarzadeh, A., Eftekhari, A., Kavetskyy, T., Khalilov, R., et al. (2021). Natural and synthetic bioinks for 3D bioprinting. Nat. Synthetic Bioinks 3D Bioprinting 1 (8), 2000097. doi:10.1002/anbr.202000097
Kontominas, M. G. (2020). Use of alginates as food packaging materials. Foods 9 (10), 1440. doi:10.3390/foods9101440
Kopf, M., Campos, D. F., Blaeser, A., Sen, K. S., and Fischer, H. (2016). A tailored three-dimensionally printable agarose-collagen blend allows encapsulation, spreading, and attachment of human umbilical artery smooth muscle cells. Biofabrication 8 (2), 025011. doi:10.1088/1758-5090/8/2/025011
Kothale, D., Verma, U., Dewangan, N., Jana, P., Jain, A., and Jain, D. (2020). Alginate as promising natural polymer for pharmaceutical, food, and biomedical applications. Curr. Drug Deliv. 17 (9), 755–775. doi:10.2174/1567201817666200810110226
Kundu, B., Rajkhowa, R., Kundu, S. C., and Wang, X. (2013). Silk fibroin biomaterials for tissue regenerations. Adv. Drug Deliv. Rev. 65 (4), 457–470. doi:10.1016/j.addr.2012.09.043
Kundu, S. C., Kundu, B., Talukdar, S., Bano, S., Nayak, S., Kundu, J., et al. (2012). Nonmulberry silk biopolymers. Biopolymers 97 (6), 455–467. doi:10.1002/bip.22024
Lee, C. F., Hsu, Y. H., Lin, Y. C., Nguyen, T. T., Chen, H. W., Nabilla, S. C., et al. (2021). 3D printing of collagen/oligomeric proanthocyanidin/oxidized hyaluronic acid composite scaffolds for articular cartilage repair. Polym. (Basel) 13 (18), 3123. doi:10.3390/polym13183123
Lee, J. M., Suen, S. K. Q., Ng, W. L., Ma, W. C., and Yeong, W. Y. (2021). Bioprinting of collagen: Considerations, potentials, and applications. Macromol. Biosci. 21 (1), e2000280. doi:10.1002/mabi.202000280
Leppiniemi, J., Lahtinen, P., Paajanen, A., Mahlberg, R., Metsa-Kortelainen, S., Pinomaa, T., et al. (2017). 3D-Printable bioactivated nanocellulose-alginate hydrogels. ACS Appl. Mater Interfaces 9 (26), 21959–21970. doi:10.1021/acsami.7b02756
Li, D., Cheng, Y., Shahzadi, I., Jiang, G., Yi, Y., Shi, X., et al. (2019). Egg source natural proteins LBL modified cellulose nanofibrous mats and their cellular compatibility. Carbohydr. Polym. 213, 329–337. doi:10.1016/j.carbpol.2019.02.096
Li, J., Fang, T., Yan, W., Zhang, F., Xu, Y., and Du, Z. (2020). Structure and properties of oxidized chitosan grafted cashmere fiber by amide covalent modification. Molecules 25 (17), 3812. doi:10.3390/molecules25173812
Li, Q., Guan, X., Cui, M., Zhu, Z., Chen, K., Wen, H., et al. (2018). Preparation and investigation of novel gastro-floating tablets with 3D extrusion-based printing. Int. J. Pharm. 535 (1-2), 325–332. doi:10.1016/j.ijpharm.2017.10.037
Li, X. H., Zhu, X., Liu, X. Y., Xu, H. H., Jiang, W., Wang, J. J., et al. (2021). The corticospinal tract structure of collagen/silk fibroin scaffold implants using 3D printing promotes functional recovery after complete spinal cord transection in rats. J. Mater Sci. Mater Med. 32 (4), 31. doi:10.1007/s10856-021-06500-2
Li, Z., Wu, N., Cheng, J., Sun, M., Yang, P., Zhao, F., et al. (2020). Biomechanically, structurally and functionally meticulously tailored polycaprolactone/silk fibroin scaffold for meniscus regeneration. Theranostics 10 (11), 5090–5106. doi:10.7150/thno.44270
Liedel, C. (2020). Sustainable battery materials from biomass. ChemSusChem 13 (9), 2110–2141. doi:10.1002/cssc.201903577
Lin, H. H., Chao, P. G., Tai, W. C., and Chang, P. C. (2021). 3D-Printed collagen-based waveform microfibrous scaffold for periodontal ligament reconstruction. Int. J. Mol. Sci. 22 (14), 7725. doi:10.3390/ijms22147725
Ling, K., Wu, H., Neish, A. S., and Champion, J. A. (2019). Alginate/chitosan microparticles for gastric passage and intestinal release of therapeutic protein nanoparticles. J. Control Release 295, 174–186. doi:10.1016/j.jconrel.2018.12.017
Liu, H., Peng, H., Wu, Y., Zhang, C., Cai, Y., Xu, G., et al. (2013). The promotion of bone regeneration by nanofibrous hydroxyapatite/chitosan scaffolds by effects on integrin-BMP/Smad signaling pathway in BMSCs. Biomaterials 34 (18), 4404–4417. doi:10.1016/j.biomaterials.2013.02.048
Liu, H., Wang, C., Sun, X., Zhan, C., Li, Z., Qiu, L., et al. (2022). Silk fibroin/collagen/hydroxyapatite scaffolds obtained by 3D printing technology and loaded with recombinant human erythropoietin in the reconstruction of alveolar bone defects. ACS Biomater. Sci. Eng. 8, 5245–5256. doi:10.1021/acsbiomaterials.2c00690
Liu, J., Song, P., Zhang, J., Nangong, Z., Liu, X., Gao, Y., et al. (2019a). Characteristics and function of the chitin binding protein from xenorhabdus nematophila. Protein Pept. Lett. 26 (6), 414–422. doi:10.2174/0929866526666190327143335
Liu, J., Sun, L., Xu, W., Wang, Q., Yu, S., and Sun, J. (2019b). Current advances and future perspectives of 3D printing natural-derived biopolymers. Carbohydr. Polym. 207, 297–316. doi:10.1016/j.carbpol.2018.11.077
Liu, X., Zheng, C., Luo, X., Wang, X., and Jiang, H. (2019c). Recent advances of collagen-based biomaterials: Multi-hierarchical structure, modification and biomedical applications. Mater Sci. Eng. C Mater Biol. Appl. 99, 1509–1522. doi:10.1016/j.msec.2019.02.070
Longinotti, C. (2014). The use of hyaluronic acid based dressings to treat burns: A review. Burns Trauma 2 (4), 162–168. doi:10.4103/2321-3868.142398
Luo, H., Lan, H., Cha, R., Yu, X., Gao, P., Zhang, P., et al. (2021). Dialdehyde nanocrystalline cellulose as antibiotic substitutes against multidrug-resistant bacteria. ACS Appl. Mater Interfaces 13 (29), 33802–33811. doi:10.1021/acsami.1c06308
Ma, L., Wang, X., Zhao, N., Zhu, Y., Qiu, Z., Li, Q., et al. (2018). Integrating 3D printing and biomimetic mineralization for personalized enhanced osteogenesis, angiogenesis, and osteointegration. ACS Appl. Mater Interfaces 10 (49), 42146–42154. doi:10.1021/acsami.8b17495
Mahedia, M., Shah, N., and Amirlak, B. (2016). Clinical evaluation of hyaluronic acid sponge with zinc versus placebo for scar reduction after breast surgery. Plast. Reconstr. Surg. Glob. Open 4 (7), e791. doi:10.1097/GOX.0000000000000747
Markstedt, K., Mantas, A., Tournier, I., Martinez Avila, H., Hagg, D., and Gatenholm, P. (2015). 3D bioprinting human chondrocytes with nanocellulose-alginate bioink for cartilage tissue engineering applications. Biomacromolecules 16 (5), 1489–1496. doi:10.1021/acs.biomac.5b00188
Matica, M. A., Aachmann, F. L., Tondervik, A., Sletta, H., and Ostafe, V. (2019). Chitosan as a wound dressing starting material: Antimicrobial properties and mode of action. Int. J. Mol. Sci. 20 (23), 5889. doi:10.3390/ijms20235889
Maturavongsadit, P., Narayanan, L. K., Chansoria, P., Shirwaiker, R., and Benhabbour, S. R. (2021). Cell-Laden nanocellulose/chitosan-based bioinks for 3D bioprinting and enhanced osteogenic cell differentiation. ACS Appl. Bio Mater 4 (3), 2342–2353. doi:10.1021/acsabm.0c01108
Mehrotra, S., Chouhan, D., Konwarh, R., Kumar, M., Jadi, P. K., and Mandal, B. B. (2019). Comprehensive review on silk at nanoscale for regenerative medicine and allied applications. ACS Biomater. Sci. Eng. 5 (5), 2054–2078. doi:10.1021/acsbiomaterials.8b01560
Meng, X. H., Yang, L. Y., Kennedy, J. F., and Tian, S. P. (2010). Effects of chitosan and oligochitosan on growth of two fungal pathogens and physiological properties in pear fruit. Carbohydr. Polym. 81 (1), 70–75. doi:10.1016/j.carbpol.2010.01.057
Mohammadinejad, R., Karimi, S., Iravani, S., and Varma, R. S. J. G. C. (2016). Plant-derived nanostructures: Types and applications. Plant-derived nanostructures types Appl. 18 (1), 20–52. doi:10.1039/c5gc01403d
Mostafalu, P., Kiaee, G., Giatsidis, G., Khalilpour, A., Nabavinia, M., Dokmeci, M. R., et al. (2017). A textile dressing for temporal and dosage controlled drug delivery. Adv. Funct. Mater. 27 (41), 1702399. doi:10.1002/adfm.201702399
Muller, M., Urban, B., Reis, B., Yu, X., Grab, A. L., Cavalcanti-Adam, E. A., et al. (2018). Switchable release of bone morphogenetic protein from thermoresponsive poly(NIPAM-co-dmaema)/cellulose sulfate particle coatings. Polym. (Basel) 10 (12), 1314. doi:10.3390/polym10121314
Ng, W. L., Chua, C. K., and Shen, Y.-F. (2019). Print me an organ! Why we are not there yet. Prog. Polym. Sci. 97, 101145. doi:10.1016/j.progpolymsci.2019.101145
Oprea, M., and Voicu, S. I. (2020). Recent advances in composites based on cellulose derivatives for biomedical applications. Carbohydr. Polym. 247, 116683. doi:10.1016/j.carbpol.2020.116683
Palaganas, N. B., Mangadlao, J. D., De Leon, A. C. C., Palaganas, J. O., Pangilinan, K. D., Lee, Y. J., et al. (2017). 3D printing of photocurable cellulose nanocrystal composite for fabrication of complex architectures via stereolithography. ACS Appl. Mater Interfaces 9 (39), 34314–34324. doi:10.1021/acsami.7b09223
Peptu, C., and Kowalczuk, M. (2018). “8 - biomass-derived polyhydroxyalkanoates: Biomedical applications,” in Biomass as renewable raw material to obtain bioproducts of high-tech value. Editors V. Popa, and I. Volf (Amsterdam, Netherlands: Elsevier), 271–313.
Pleissner, D., and Kummerer, K. (2020). Green chemistry and its contribution to industrial biotechnology. Adv. Biochem. Eng. Biotechnol. 173, 281–298. doi:10.1007/10_2018_73
Przekora, A. (2019). The summary of the most important cell-biomaterial interactions that need to be considered during in vitro biocompatibility testing of bone scaffolds for tissue engineering applications. Mater Sci. Eng. C Mater Biol. Appl. 97, 1036–1051. doi:10.1016/j.msec.2019.01.061
Rastogi, P., and Kandasubramanian, B. (2019). Review of alginate-based hydrogel bioprinting for application in tissue engineering. Biofabrication 11 (4), 042001. doi:10.1088/1758-5090/ab331e
Romanazzi, G., Feliziani, E., and Sivakumar, D. (2018). Chitosan, a biopolymer with triple action on postharvest decay of fruit and vegetables: Eliciting, antimicrobial and film-forming properties. Front. Microbiol. 9, 2745. doi:10.3389/fmicb.2018.02745
Roseti, L., Parisi, V., Petretta, M., Cavallo, C., Desando, G., Bartolotti, I., et al. (2017). Scaffolds for bone tissue engineering: State of the art and new perspectives. Mater Sci. Eng. C Mater Biol. Appl. 78, 1246–1262. doi:10.1016/j.msec.2017.05.017
Sanchez-Ballester, N. M., Bataille, B., and Soulairol, I. (2021). Sodium alginate and alginic acid as pharmaceutical excipients for tablet formulation: Structure-function relationship. Carbohydr. Polym. 270, 118399. doi:10.1016/j.carbpol.2021.118399
Schutz, K., Placht, A. M., Paul, B., Bruggemeier, S., Gelinsky, M., and Lode, A. (2017). Three-dimensional plotting of a cell-laden alginate/methylcellulose blend: Towards biofabrication of tissue engineering constructs with clinically relevant dimensions. J. Tissue Eng. Regen. Med. 11 (5), 1574–1587. doi:10.1002/term.2058
Shi, W., Sun, M., Hu, X., Ren, B., Cheng, J., Li, C., et al. (2017). Structurally and functionally optimized silk-fibroin-gelatin scaffold using 3D printing to repair cartilage injury in vitro and in vivo. Adv. Mater 29 (29), 1701089. doi:10.1002/adma.201701089
Shoulders, M. D., and Raines, R. T. (2009). Collagen structure and stability. Annu. Rev. Biochem. 78, 929–958. doi:10.1146/annurev.biochem.77.032207.120833
Simoes, D., Miguel, S. P., Ribeiro, M. P., Coutinho, P., Mendonca, A. G., and Correia, I. J. (2018). Recent advances on antimicrobial wound dressing: A review. Eur. J. Pharm. Biopharm. 127, 130–141. doi:10.1016/j.ejpb.2018.02.022
Son, B. T., Long, N. V., and Nhat Hang, N. T. (2021). The development of biomass-derived carbon-based photocatalysts for the visible-light-driven photodegradation of pollutants: A comprehensive review. RSC Adv. 11 (49), 30574–30596. doi:10.1039/d1ra05079f
Sorushanova, A., Delgado, L. M., Wu, Z., Shologu, N., Kshirsagar, A., Raghunath, R., et al. (2019). The collagen suprafamily: From biosynthesis to advanced biomaterial development. Adv. Mater 31 (1), e1801651. doi:10.1002/adma.201801651
Srivastava, R. K., Shetti, N. P., Reddy, K. R., Kwon, E. E., Nadagouda, M. N., and Aminabhavi, T. M. (2021). Biomass utilization and production of biofuels from carbon neutral materials. Environ. Pollut. 276, 116731. doi:10.1016/j.envpol.2021.116731
Stiglic, A. D., Gurer, F., Lackner, F., Bracic, D., Winter, A., Gradisnik, L., et al. (2022). Organic acid cross-linked 3D printed cellulose nanocomposite bioscaffolds with controlled porosity, mechanical strength, and biocompatibility. iScience 25 (5), 104263. doi:10.1016/j.isci.2022.104263
Su, H., Li, Q., Li, D., Li, H., Feng, Q., Cao, X., et al. (2022). A versatile strategy to construct free-standing multi-furcated vessels and a complicated vascular network in heterogeneous porous scaffolds via combination of 3D printing and stimuli-responsive hydrogels. Mater Horiz. 9 (9), 2393–2407. doi:10.1039/d2mh00314g
Su, X., Wang, T., and Guo, S. (2021). Applications of 3D printed bone tissue engineering scaffolds in the stem cell field. Regen. Ther. 16, 63–72. doi:10.1016/j.reth.2021.01.007
Sun, W., Gregory, D. A., Tomeh, M. A., and Zhao, X. (2021). Silk fibroin as a functional biomaterial for tissue engineering. Int. J. Mol. Sci. 22 (3), 1499. doi:10.3390/ijms22031499
Sun, Y., Yang, C., Zhu, X., Wang, J. J., Liu, X. Y., Yang, X. P., et al. (2019). 3D printing collagen/chitosan scaffold ameliorated axon regeneration and neurological recovery after spinal cord injury. J. Biomed. Mater Res. A 107 (9), 1898–1908. doi:10.1002/jbm.a.36675
Talebian, S., Foroughi, J., Wade, S. J., Vine, K. L., Dolatshahi-Pirouz, A., Mehrali, M., et al. (2018). Biopolymers for antitumor implantable drug delivery systems: Recent advances and future outlook. Adv. Mater 30 (31), e1706665. doi:10.1002/adma.201706665
Tavianatou, A. G., Caon, I., Franchi, M., Piperigkou, Z., Galesso, D., and Karamanos, N. K. (2019). Hyaluronan: Molecular size-dependent signaling and biological functions in inflammation and cancer. FEBS J. 286 (15), 2883–2908. doi:10.1111/febs.14777
Tiwari, S., and Bahadur, P. (2019). Modified hyaluronic acid based materials for biomedical applications. Int. J. Biol. Macromol. 121, 556–571. doi:10.1016/j.ijbiomac.2018.10.049
Tsukamoto, Y., Akagi, T., and Akashi, M. (2020). Vascularized cardiac tissue construction with orientation by layer-by-layer method and 3D printer. Sci. Rep. 10 (1), 5484. doi:10.1038/s41598-020-59371-y
Tu, H., Wu, G., Yi, Y., Huang, M., Liu, R., Shi, X., et al. (2019). Layer-by-layer immobilization of amphoteric carboxymethyl chitosan onto biocompatible silk fibroin nanofibrous mats. Carbohydr. Polym. 210, 9–16. doi:10.1016/j.carbpol.2019.01.047
Tungprapa, S., Jangchud, I., and Supaphol, P. (2007). Release characteristics of four model drugs from drug-loaded electrospun cellulose acetate fiber mats. Polymer 48 (17), 5030–5041. doi:10.1016/j.polymer.2007.06.061
Vanderburgh, J., Sterling, J. A., and Guelcher, S. A. (2017). 3D printing of tissue engineered constructs for in vitro modeling of disease progression and drug screening. Ann. Biomed. Eng. 45 (1), 164–179. doi:10.1007/s10439-016-1640-4
Vehlow, D., Schmidt, R., Gebert, A., Siebert, M., Lips, K. S., and Muller, M. (2016). Polyelectrolyte complex based interfacial drug delivery system with controlled loading and improved release performance for bone therapeutics. Nanomater. (Basel) 6 (3), 53. doi:10.3390/nano6030053
Venkata Mohan, S., Nikhil, G. N., Chiranjeevi, P., Nagendranatha Reddy, C., Rohit, M. V., Kumar, A. N., et al. (2016). Waste biorefinery models towards sustainable circular bioeconomy: Critical review and future perspectives. Bioresour. Technol. 215, 2–12. doi:10.1016/j.biortech.2016.03.130
Ventola, C. L. (2014). Medical applications for 3D printing: Current and projected uses. P T 39 (10), 704–711.
Verlee, A., Mincke, S., and Stevens, C. V. (2017). Recent developments in antibacterial and antifungal chitosan and its derivatives. Carbohydr. Polym. 164, 268–283. doi:10.1016/j.carbpol.2017.02.001
Wang, S., Yan, F., Ren, P., Li, Y., Wu, Q., Fang, X., et al. (2020). Incorporation of metal-organic frameworks into electrospun chitosan/poly (vinyl alcohol) nanofibrous membrane with enhanced antibacterial activity for wound dressing application. Int. J. Biol. Macromol. 158, 9–17. doi:10.1016/j.ijbiomac.2020.04.116
Wutticharoenmongkol, P., Hannirojram, P., and Nuthong, P. (2019). Gallic acid-loaded electrospun cellulose acetate nanofibers as potential wound dressing materials. Polym. Adv. Technol. 30, 1135–1147. doi:10.1002/pat.4547
Xi, Y., Ge, J., Guo, Y., Lei, B., and Ma, P. X. (2018). Biomimetic elastomeric polypeptide-based nanofibrous matrix for overcoming multidrug-resistant bacteria and enhancing full-thickness wound healing/skin regeneration. ACS Nano 12 (11), 10772–10784. doi:10.1021/acsnano.8b01152
Xi, Y., Ge, J., Wang, M., Chen, M., Niu, W., Cheng, W., et al. (2020). Bioactive anti-inflammatory, antibacterial, antioxidative silicon-based nanofibrous dressing enables cutaneous tumor photothermo-chemo therapy and infection-induced wound healing. ACS Nano 14 (3), 2904–2916. doi:10.1021/acsnano.9b07173
Xia, J., Zhang, H., Yu, F., Pei, Y., and Luo, X. (2020). Superclear, porous cellulose membranes with chitosan-coated nanofibers for visualized cutaneous wound healing dressing. ACS Appl. Mater Interfaces 12 (21), 24370–24379. doi:10.1021/acsami.0c05604
Yadav, M., Goel, G., Hatton, F. L., Bhagat, M., Mehta, S. K., Mishra, R. K., et al. (2021). A review on biomass-derived materials and their applications as corrosion inhibitors, catalysts, food and drug delivery agents. Curr. Res. Green Sustain. Chem. 4, 100153. doi:10.1016/j.crgsc.2021.100153
Yang, Y., Li, W., Yu, D. G., Wang, G., Williams, G. R., and Zhang, Z. (2019). Tunable drug release from nanofibers coated with blank cellulose acetate layers fabricated using tri-axial electrospinning. Carbohydr. Polym. 203, 228–237. doi:10.1016/j.carbpol.2018.09.061
Yao, M., Zou, Q., Zou, W., Xie, Z., Li, Z., Zhao, X., et al. (2021). Bifunctional scaffolds of hydroxyapatite/poly(dopamine)/carboxymethyl chitosan with osteogenesis and anti-osteosarcoma effect. Biomater. Sci. 9 (9), 3319–3333. doi:10.1039/d0bm01785j
Yao, X., Zou, S., Fan, S., Niu, Q., and Zhang, Y. (2022). Bioinspired silk fibroin materials: From silk building blocks extraction and reconstruction to advanced biomedical applications. Mater Today Bio 16, 100381. doi:10.1016/j.mtbio.2022.100381
Yi, H. G., Jeong, Y. H., Kim, Y., Choi, Y. J., Moon, H. E., Park, S. H., et al. (2019). A bioprinted human-glioblastoma-on-a-chip for the identification of patient-specific responses to chemoradiotherapy. Nat. Biomed. Eng. 3 (7), 509–519. doi:10.1038/s41551-019-0363-x
Yuan, M., Dai, F., Li, D., Fan, Y., Xiang, W., Tao, F., et al. (2020). Lysozyme/collagen multilayers layer-by-layer deposited nanofibers with enhanced biocompatibility and antibacterial activity. Mater Sci. Eng. C Mater Biol. Appl. 112, 110868. doi:10.1016/j.msec.2020.110868
Zamboni, F., Vieira, S., Reis, R. L., Oliveira, J. M., and Collins, M. N. (2018). The potential of hyaluronic acid in immunoprotection and immunomodulation: Chemistry, processing and function. Prog. Mater. Sci. 97, 97–122. doi:10.1016/j.pmatsci.2018.04.003
Zhang, X., Yuan, J., Li, F., and Xiang, J. (2021). Chitin synthesis and degradation in Crustaceans: A genomic view and application. Mar. Drugs 19 (3), 153. doi:10.3390/md19030153
Zhou, J., Zhang, B., Liu, X., Shi, L., Zhu, J., Wei, D., et al. (2016). Facile method to prepare silk fibroin/hyaluronic acid films for vascular endothelial growth factor release. Carbohydr. Polym. 143, 301–309. doi:10.1016/j.carbpol.2016.01.023
Zhu, X., Hou, X., Ma, B., Xu, H., and Yang, Y. (2019). Chitosan/gallnut tannins composite fiber with improved tensile, antibacterial and fluorescence properties. Carbohydr. Polym. 226, 115311. doi:10.1016/j.carbpol.2019.115311
Keywords: biomass-derived materials, antibacterial fiber, skin wound dressings, bone tissue engineering, three-dimensional printing
Citation: Li D, Wang Y, Huang W and Gong H (2023) Biomass-derived fiber materials for biomedical applications. Front. Mater. 10:1058050. doi: 10.3389/fmats.2023.1058050
Received: 30 September 2022; Accepted: 13 January 2023;
Published: 03 February 2023.
Edited by:
Gareth Williams, University College London, United KingdomReviewed by:
Iriczalli Cruz Maya, Department of Chemical Sciences and Materials Technologies (CNR), ItalySangram Keshari Samal, Regional Medical Research Center (ICMR), India
Wei Long Ng, Nanyang Technological University, Singapore
Copyright © 2023 Li, Wang, Huang and Gong. This is an open-access article distributed under the terms of the Creative Commons Attribution License (CC BY). The use, distribution or reproduction in other forums is permitted, provided the original author(s) and the copyright owner(s) are credited and that the original publication in this journal is cited, in accordance with accepted academic practice. No use, distribution or reproduction is permitted which does not comply with these terms.
*Correspondence: Wenhua Huang, Z29uZ2hhaWh1YW4yMDEzQDE2My5jb20=; Haihuan Gong, aHVhbmd3ZW5odWEyMDA5QDEzOS5jb20=
†These authors have contributed equally to this work