Use of shear thickening fluids in sport protection applications: a review
- 1Sport Technology LAB, Department of Civil, Chemical, Environmental and Materials Engineering, University of Bologna, Bologna, Italy
- 2Laboratory for Bioinspired, Bionic, Nano, Meta, Materials & Mechanics, Department of Civil, Environmental and Mechanical Engineering, University of Trento, Trento, Italy
- 3School of Engineering and Materials Science, Queen Mary University of London, London, United Kingdom
- 4RE-SPORT srl, Bologna, Italy
Nowadays, safety devices (e.g., helmets, safety barriers, body protectors, etc.) producers are developing and testing innovative smart materials to reach the ever-growing request for higher performances arising from international standards and markets. Shear thickening fluids (STFs) have attracted special attention because of their non-linear mechanical response and high-energy dissipation effectiveness. In particular, within STF, shear thickening gels (STGs), a class of high-molecular-weight and crosslinked polymers have exhibited great stability and shear-dependent properties ascribable to the presence of dynamic boron-oxygen crosslinks within the polymeric structure. Hence, it is essential to investigate the rheological behavior of these system for their application in new flexible and impact protection devices. This review focuses on the description of the peculiar advantages of using STFs with regards to traditional materials in sport protective equipment. Moreover, the preparation and use of STFs for impact protection including all the scientific productions and the conspicuous patent literature on this field - from dilatant suspension until the latest STGs–have been reviewed in detail. Commercial helmets and body protectors containing STFs have also been reviewed and reported. Finally, the use of STGs not embedded in foams in sport helmet is also discussed and a final part on future perspective of STFs for sport protection is provided.
1 Introduction
It is well known that sports and leisure activities in general, involve several benefits on both physical and mental health. Every year, a significant number of people from young to adults, participate in organized competitions and sport games such as football, basketball, baseball or tennis, in either public and private playgrounds (Eather et al., 2023). However, the current sports and fitness activities along with governments promotion of healthy lifestyles have led to a significant increase in injuries as well. Although mostly are not considered to be fateful, we cannot neglect the fact that sport injuries cause physical disabilities as well as pain and other types of health discomfort, resulting in high expenditures for the healthcare system (Guskiewicz et al., 2007; Meeuwisse and Dvorak, 2017; Harmon et al., 2019; Moreland and Barkley, 2021; Committee SCAR, 2023).
It has been reported that in EU nearly 2.7 million people, were affected by sports-related injuries in 2020, with 4.9% needing emergency department (ED) treatment (Giustini et al., 2023). Among the primary sports that causes injuries (Figure 1) (Giustini et al., 2023), team-ball sports account for 44% (35% of which associated to football). In the United States, for example, for 2022 the sport medicine market has been estimated around 6.3 million of dollars and it has been forecasted to grow in the next years (Digital Journal, 2023; OpenPR, 2023). In 2022, nearly 3.6 million of people suffered from sports trauma, with 92% of these ED treated and released (National Safety Council, 2023). As per data from the National Electronic Injury Surveillance System (NEISS), the number of reported sports and recreational injuries hit a historic low in 2020. However, there was a notable uptick in injuries, with a 20% increase in 2021, followed by another 12% increase in 2022. Notably, the age group most affected by these injuries falls within the 5 to 24-year-old bracket. The activities most commonly linked to these injuries include exercise, cycling, and basketball (Figure 1) (National Safety Council, 2023).
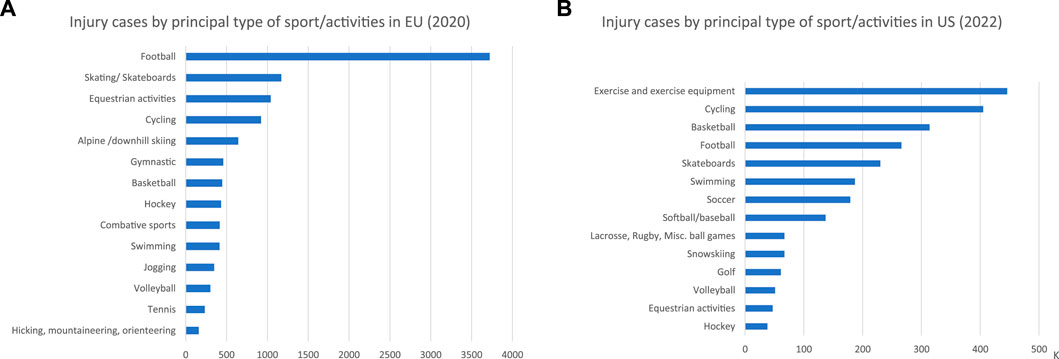
FIGURE 1. Injury cases by principal type of sport/activities in Europe (A) and U.S. (B). Data adapted from references (Giustini et al., 2023; National Safety Council, 2023).
Both in EU and U.S. countries, common sport-related injuries may include fractures, dislocations, sprains, strains, open wounds and tendinitis, located in various part of the body such as head/face, shoulder, elbow, wrist, knee and ankle (National Institute of Health, 2021; Giustini et al., 2023). In the U.S. for example, the annual estimated cost of medical care following ED treatment for some of the non-fatal injuries that could also occur in sport, is detailed in Table 1(12). Medical expenses could represent a heavy burden especially for those without health insurance coverage, who can experience unanticipated and unaffordable healthcare costs. As a consequence, injuries prevention has gained particularly attention over the past years due to the increase in sports participation and traumas associated. Among the countermeasures regarding injury prevention, the use of appropriate protective clothes and equipment is undoubtedly one of the most relevant (Emery and Pasanen, 2019). Several studies have shown that the types of injuries can significantly vary from a sport to another also due to the required protective equipment (Brunner et al., 2019; Chen et al., 2019; Emery and Pasanen, 2019; Knapik et al., 2019; Polmann et al., 2020; Dickson and Terwiel, 2021; Emery, 2023).

TABLE 1. Yearly expenses in United States for selected non-fatal injuries by body region. (Adapted from Peterson C, Xu L, Florence C. Average medical cost of fatal and non-fatal injuries by type in the United States. Inj Prev. 2021; 27 1):1–10.).
The sporting goods industry has evolved throughout the years in order to meet the recent safety standards and the different needs of the athletes and consumers all over the world. The industry has also, in many circumstances, promoted and helped the development of new sports, leading to new types of products. Sport protective market was estimated $7.118 million in 2018 and it is expected to grow up to $10.171 million by 2026 (Bhandalkar and Deshmukh, 2019). In the past times, most sporting equipment of the late 19th and early 20th centuries, was extremely basic and only offered little protection. The majority of the protective equipment was made up of leather padded with horse hair. Helmets with rigid plastic shells became popular from 1940–1950 in some contact sports such as baseball or football and later in 1980, they became the norm also for hockey with manufacturers adding cages and full-face shields made of plastics as well (Dlugosch et al., 2012; The Evolution of Sports Equipment, 2016). Since then, impact protectors have gained increasing importance in the sports device market becoming an emerging sub-category as a consequence of the discoveries and innovations of these recent years.
The introduction of various regulatory bodies and organizations such as ASTM (American Society for Testing and Materials), NOCSAE (National Operating Committee on Standards for Athletic Equipment), ISO (International Organization of Standardization), ANSI (American National Standard Institute) and CPSC (Consumer Product Safety Commission) have contributed to improve the quality and efficacy of protectors by setting standards and rules for the different equipment (United States Consumer Product Safety Commission, 2014; Pfriem, 2016; OECD, 2021). Significance of standards for sport protective equipment can vary, contingent upon the specific sport or activity in question. However, certain standards are universally recognized and of paramount importance.
In the realm of ice hockey, ASTM F1045 (F1045-16 A, 2016) plays a critical role, ensuring that ice hockey helmets offer effective protection against high-impact collisions, emphasizing the need for reliable retention systems. For recreational snow sports, such as skiing and snowboarding, ASTM F2040 (ASTM F2040-02, 2002) comes to the fore. It underscores the necessity of impact protection and secure retention systems in helmets designed for these winter activities, safeguarding participants from potential head injuries. ASTM F1492 (International A, 2008) outlines safety requirements and test methods for skateboarding helmets, ensuring they provide adequate protection against head injuries. NOCSAE standards set the bar for safety in various sports equipment, with specific standards for football helmets (ND002) (National Operating Committee on Standards for Athletic Equipment NOCSAE, 2012) and lacrosse helmets (ND041) (NOCSAE, 2015). Adherence to these standards is indispensable for ensuring the wellbeing of athletes in these physically demanding sports. In the context of bicycle safety in the United States, CPSC Regulations (16 CFR Part 1203) (Part et al., 2023) reign supreme. These regulations govern bicycle helmets, dictating criteria for impact performance, retention systems, and other safety features. Their adherence is pivotal for safeguarding the heads of cyclists on American roads. For motorcyclists, ECE 22.0 (Homologation ECE-2206, 2024) regulated by the Economic Commission for Europe (ECE) establishes the benchmarks for protective helmets. This standard sets safety and performance requirements for motorcycle helmets sold and used in many countries within Europe and other parts of the world. A part of the helmets, the most important standards for sport body protectors, such as those used in equestrian sports and motorcycling, are typically the European standards EN 13158 (Group, 2000) that sets safety requirements and test methods for equestrian body protectors and the EN 1621.2 (EN BS, 2014) that specifies safety requirements and test methods for back protectors designed for motorcycle riders.
Generally, impact protective garments and equipment should cover wide areas of the human body and thus, must possess the lowest density and thickness (or at least their product thus the mass per unit area) as possible in order to improve the overall comfort (also thanks to a reduced bending rigidity, scaling with the thickness cube) as well as thermal moisture management, while not affecting the sport performances. Most of the sporting equipment is made of soft cushioning materials such as deformable foams or Non-Newtonian fluids layers, combined with rigid and stiff materials (hard-shell). These special designed products enable reducing the peak forces of instantaneous impacts by shock absorption and opportune pressure distribution (Dlugosch et al., 2012; Haid et al., 2023). For the most part, impacts are attenuated by elastic, viscoelastic and also plastic deformation of the soft material due to its lower compressive rigidity compared to the hard shell (Duncan et al., 2018). One of the peculiarities of viscoelastic materials is in fact the ability to reduce the intensity of the impact forces. This is possible by spreading the impact event over a wider time and/or by absorbing the energy associated to the impact. The force during impact in sporting protective equipment should in principle exhibit a maximum value at small strain, followed by a plateau region where the material continues deforming without any additional load increment, in order to increase the energy absorption (that can be expressed as the integral of the transmitted force over the impact time or deformation) and simultaneously limit the transmitted force, before foam densification occurring at high strains causing high deceleration and higher force transmission (Duncan et al., 2018).
Impact protective equipment comprises different models and devices, including helmets for head protection and various type of body protectors (e.g., back protectors, knee and elbow pads) to safeguard vital organs and prevent fractures (Dlugosch et al., 2012). Non-resilient materials (such as polystyrene-based foams or tubular structures), are used for one-time or occasional impacts and need to be replaced once the material has been altered or permanently damaged, whereas highly resilient materials are often chosen for multi-impact protection purposes because of their ability to regain their original shape after the impact and therefore being able to protect from additional impacts (Caswell et al., 2007).
In general, a wide range of materials are employed in the manufacture of a sportive protective equipment. Rigid polymeric resins, for example, can be used for the production of the outer part of a protection device. The aforementioned deflection and shock absorption mechanisms from the shell to the inner core of a device are critical stages to ensure a great performance along with an adequate protection (Caswell et al., 2007). Accordingly, the selection of materials and construction of a sportive protective equipment will directly affect the protection performances of the final products. For most of the athletic sports the polymer materials chosen for the shell fabrication are divided in three subcategories. i) Bisphenol A polycarbonate (PC) shells are the best option to ensure the highest level of impact protection and shock absorption. This material is particularly used in high performances and multi-impact products such as in football helmets. ii) Acrylonitrile–butadiene–styrene (ABS) resins are commonly employed for the shell material construction in medium energy impact systems such as in ice-hockey, lacrosse and baseball head protections. iii) Single high-impact, high-density and less expensive devices such as shoulder pads or bicycling and skateboarding helmets are typically made up of polyolefins, including polyethylene (PE) and polypropylene (PP) materials (Caswell et al., 2007).
Advanced lightweight composite materials such as carbon-fibre and aramid-fibre epoxy matrix systems, initially used in military field, are also employed in the production of many sportive devices because of their high performances in terms of low weight per unit area vs. impact protection. One of the main limits regarding the use of such materials is undeniably their cost. Composite fabrication technologies are labour-intensive, require post-finishing operations, and have very high cure times that limit their utility for high-volume sporting goods (Caswell et al., 2007).
Foams are widely used as cushioning material and essentially, they can be divided in two subcategories: open-cell or closed-cell foams. Open-cell foams are low-density materials able to absorb fluids (both gases and liquids), and are typically used as padding material for bones and/or hard edges of protective equipment (Physiopedia. Protective Sports Equipments, 2022). Among the open-cell foams, polyurethane (PU) based materials are the most popular and broadly used in shock absorption applications. Unfortunately, because of their low density, open-cell foams are considered to be not suitable materials for high-energy impact protection (Manske et al., 2011). On the contrary, closed-cell ones exhibit better shock damping properties along with lower fluid absorption and for these reasons are preferred over the open-cell foams for impact protection purposes (Caine et al., 2009; Manske et al., 2011; Physiopedia. Protective Sports Equipments, 2022). Commonly used closed-cell foams for impact protection devices, such as in helmet cushioning, include expanded polystyrene (EPS), polyvinylchloride (PVC), polyethylene (PE), and polypropylene (PP) - based materials. These foams are also often selected whether the application requires long levels of moisture contact, since PU systems usually undergo hydrolytic degradation with long-term moisture (Caswell et al., 2007). Dual-density foams are also commercially available. These systems are basically made of a high-density foam layer glued together with a low-density one in order to modulate density and maintain an adequate level of protection at the same time. Some high-density foams are also thermo-moldable and thus, when heated they start softening, allowing them to be customized as needed to different shapes and sizes. After these materials are cooled they are able to preserve their final shape until reheated. Cramer High Density Foam is an example of a commercial thermo-moldable foam that can be heated and shaped as needed, thus improving the ergonomic comfort (Caswell et al., 2007; Manske et al., 2011).
Air management pads are a new type of protective padding, which are mainly used to secure a high level of shock absorption in lightweight devices. These pads consist of several open and closed-cell foam pads encapsulated in a polyurethane or nylon material (Caine et al., 2009). These systems are often sealed in order to prevent quick deformation of the foam thus enabling energy dissipation over a wider area. Air pads are commonly employed in football shoulder pads with sport companies designing various foam patterns to be placed within the nylon covering. These devices are higher-priced than traditional sport equipment, and the linings need to be changed or fixed once the nylon covering is damaged, thus avoiding air to escape during the impact. Nylon is a particularly resistant material which help restraining fluids entrance inside the pads, avoid to weight down the equipment. Furthermore, most of the body fluids can be easily cleaned from the nylon material with adequate products commercially available, whereas, the cleaning of traditional padding is more complicated because the exposed foam (even the closed-cell ones) is able to absorb fluids losing its original features and thus, affecting also the mechanical properties of the product (Manske et al., 2011).
Felt, in various thicknesses, is another broadly used material in protective equipment manufacture. In the past, felt has been employed for the production of protective padding (DeHass, 2009), however, it has been partially outraced by foams because of their better waterproof properties and protection in term of superior shock-absorption. Felt is still used for creating various types of supports and protective pads. It can be shaped in many different ways in order to offer the support needed and to ensure a better pressure distribution on the areas (Manske et al., 2011). Dainese and Alpina, for example, have recently launched on the market their multi-sport back protectors: Flexagon PL Waistcoat (Dainese, 2023), incorporated into a Polartec Power Wool fabric vest comprising two layers of crash absorb memory foam and PROLAN a wool protector made from pressed sheep’s wool. This latter multi-layer structure meets the TÜV standard 1621-2 Level 1 for protectors and has temperature-independent damping properties (Alpina, 2023).
Other polymeric materials, starting from rubber-like products such as gutta percha, have been employed in the production of mouthguards and other sportive devices in order to provide protection against soft tissue facial injuries. Examples of polymers used in the design and production of mouthguards include: polyethylene–polyvinylacetate copolymers (EVA), methyl-methacrylates, silicone rubbers, polyvinylchlorides (PVC), natural rubber, and polyurethane (PU) elastomers (Going et al., 1974; Tran et al., 2001).
From what reported in the previous paragraphs it is clear that the actual systems used for impact protection lack of some fundamental characteristics and needs to be implemented in order to guarantee safety, performances and comfort. In particular, the classical systems made of a rigid outer shell and a soft liner (for example, for body protectors and helmets) can be used for single impact applications and due to the rigidity of the outer shell (and in the case of the helmets also of the inner liner) are not ergonomically comfortable. Moreover, these systems are generally heavy, thus limiting the performances of athletes. The multi-material construction of these systems also significantly limits the possibility to recycle the protective devices at the end of their use since multi-material assemblies are significantly more difficult to be separated and therefore recycled, thus affecting the environmental sustainability of the protective devices.
In order to solve the above-mentioned problems, in the last few years protective systems based on non-Newtonian fluids have been widely developed and commercialized (Zhao et al., 2020; Polyanswer, 2023b; COLPHARMA Srl, 2023; NORSOREX®, 2023; Rogers Corporation, 2023; D3O, 2023b; RHEON Labs, 2023; LEATT, 2020; POC Sports, 2023; Fluid Inside, 2023). As will be described in the next paragraphs, this type of materials has a soft behavior at low shear strain rate thus providing a good comfort, while at the high shear strain rates that occur during an impact, they behave as a rigid material, thus dissipating the impact on a wider zone (Deshpande et al., 2010). In this way, a single material is able to substitute the different layers generally present in classical hard-shell protectors, thus permitting an easier recycle. Moreover, this class of material can be impacted multiple times and can also have self-healing characteristics. In the next paragraphs, a review of the characteristics of the non-Newtonian fluids, their production and application is reported, highlighting the main improvement that this class of materials permits with regards to more traditional systems.
2 Shear thickening fluids: classification and characteristics
Several polymeric materials used in various chemical and industrial applications do not adhere to the conventional Newtonian viscosity postulate and therefore are classified as non-Newtonian fluids. In general, non-Newtonian fluids can behave as an elastic solid under some conditions, or as a viscous liquid under other circumstances since their viscosity depends on the applied shear strain rate (Cross, 1965). Many high-molecular weight substances such as suspensions, polymer solutions, various synthetic polymers, pharmaceutical formulations and biological fluids, present complex rheological behaviour that differs from the common ordinary low-molecular-weight Newtonian fluids such as air, water, oils, etc. (Hady, 1995). Numerous researches have been carried out on non-Newtonian fluids because of their many valuable applications in the design of equipment and in industrial processing. On a scientific base real fluids and their models can be divided into three main categories (Hady, 1995; Sochi, 2010):
1) Time-independent fluids, which exhibit properties independent by time;
2) Viscoelastic fluids, showing both viscous and elastic response;
3) Time-dependent fluids, having properties changing with time while the fluid is deformed;
Time-independent fluids are further classified into four main categories denominated as follows (Figure 2) (Hady, 1995):
1) Newtonian fluids
2) Viscoplastic fluids
3) Shear thinning fluids (pseudo-plastic fluids)
4) Shear thickening fluids (dilatant fluids)
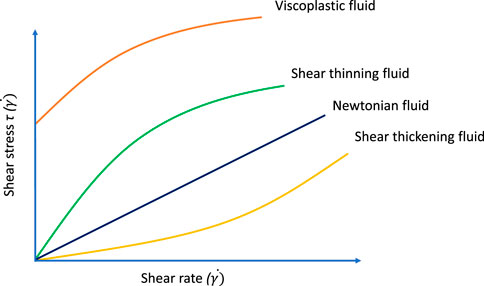
FIGURE 2. Classification of time-independent non-Newtonian fluids. (Adapted from: Deshpande AP, Krishnan JM, Kumar PBS. Rheology of complex fluids. Rheology of Complex Fluids. 2010. 1–257 p.).
Their classification depends on the viscosity function
Newtonian fluids exhibit a behaviour according to which the viscosity is constant. Their characteristic flow curve, at a given temperature and pressure, is perfectly linear and passes through the origin, as shown in Figure 2. The slope of the flow curve, which is constant, is the viscosity of the fluid. In viscoplastic materials, flow is initiated by a non-zero shear stress and below this critical stress value (yield stress τγ) there is no deformation and the material behaves as a (perfectly rigid) solid, but when this limit is exceeded the material flows like a viscous fluid. The related flow curve (Figure 2), exhibits the same features of a shear-thinning material but does not pass through the origin. Indeed, the flow curve of shear thinning materials passes through the origin and the rate of increase in shear stress decreases as the shear rate grows, thus the slope/viscosity decreases with the shear rate.
Lastly, shear thickening fluids (STFs)–also known as dilatant or shear stiffening fluids - show a flow curve passing through the origin and a viscosity increasing with the shear rate (Deshpande et al., 2010; Fester et al., 2012). This particular behaviour is retained to be a unique phenomenon in both polymeric materials and fluids. Because of their features and structures, shear thickening materials are able to adapt to different stress conditions, reversibly reacting to external influences and possessing extraordinary self-healing properties. For these reasons, as will be reported in detail in the following paragraphs, they are suitable for multi-impact protection devices and vibration damping systems. Dilatant materials can be grouped into two subcategories: suspensions and crosslinked gels (Wei et al., 2022).
2.1 STSs composition and properties
Shear thickening suspensions (STSs) usually consist of a dispersed phase, mainly inorganic or organic particles such as silica (Li et al., 2017), titania (Yang et al., 2001), calcium carbonate (Egres and Wagner, 2005), cornstarch (Fall et al., 2008) or polymer nanoparticles and a viscous carrier fluid (mainly polyethylene glycol or ethylene glycol). In general, fumed silica is preferred over other particles for STFs synthesis due to its better behaviour under shear forces. Beside enhancing impact resistance of STSs, this material is used in a variety of industrial applications (e.g., coatings and reinforcing fillers) (Sema, 2013). Fumed silica is produced by flame pyrolysis of silicon precursors (e.g., silicon tetrachloride or quartz sand) and consists of primary particles of amorphous silica fused into branched secondary particles which then create further agglomerates (Flörke et al., 2000; Garrett, 2017). The hydrogen bonding between hydroxyl groups on the surface of primary particles of fumed silica generates net-like structures and is responsible of its shear thickening behaviour and hydrophilicity (Sema, 2013). Furthermore, this powder, possesses extremely low bulk density along with high surface area (Flörke et al., 2000). Carrier fluids play also a significant role in controlling the rheological behaviour of shear thickening suspensions. Indeed, these fluids are adsorbed on the surface of the dispersed material increasing their diameter and increasing both viscosity and stability of the suspension (Sema, 2013).
2.1.1 Rheology of STSs
The properties of these systems depend on the dispersed phase and dispersion media (Zarei and Aalaie, 2020). Based on the combinations of both phase, suspensions exhibit different rheological behaviour, in terms of discontinuous shear thickening (DST) and continuous shear thickening (CST) (Zhao et al., 2020). Carrier fluids possess a Newtonian behaviour, meaning that dispersed particles are mainly responsible for the shear stiffening mechanism of the suspensions (Gürgen et al., 2017). As illustrated in Figure 3, at low strain rate particles in STFs are dispersed in the liquid media and are in equilibrium. With the shear rate increase, STFs exhibit shear thinning properties and the viscosity decreases due to formation of layered structures. As the shear rate exceed a critical value, these materials show shear thickening behaviour (Figure 3) (Zhao et al., 2020).
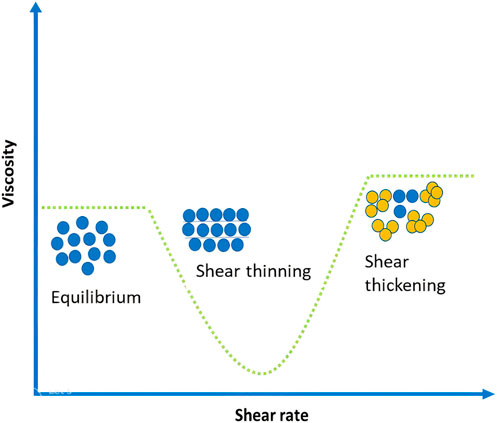
FIGURE 3. Rheological behaviour of STSs. (Adapted from: Wagner, N.J., and Brady, J.F. (2009). Shear thickening in colloidal dispersions. Phys. Today 62, 27–32).
Traditionally, the rheological properties of these suspensions have been explained by the order-disorder and hydrocluster theories (Gürgen et al., 2017; Zarei and Aalaie, 2020). The order-disorder theory describes transition from an ordered flow with low shear rate and little interactions between particles to a disordered system with enhanced shear rate and interaction between particles (Zarei and Aalaie, 2020). The hydrocluster theory relates with tendency of particles to create clusters under certain shear forces, responsible for the increase in drag forces between particles (Zarei and Aalaie, 2020). Hoffmann introduced a model, mainly based on physical contact within particles (Hoffman, 1998; Gürgen et al., 2017). According to this theory, particles are disposed in layers, as the shear thickening mechanism begins, a hydrodynamic force, lead particles out of this ordered status (Hoffman, 1972; Hoffman, 1974). At this stage, interactions between particles are due to both physical contact or clustering. Further studies, suggested that also frictional and lubrification forces play an important role in both continuous and discontinuous shear thickening mechanism (Zhou et al., 1999; Seto et al., 2013; Wyart and Cates, 2014). According to rheology models based on physical contact, at low shear rate there is low contact between particles with hydrodynamic lubrification being responsible of the shear thinning behaviour of STFs (Zhou et al., 1999; Seto et al., 2013; Wyart and Cates, 2014; Zarei and Aalaie, 2020). At a critical value of shear stress, contact forces increase and colloidal particles start interacting at high strain rate, generating discontinuous shear thickening (Zarei and Aalaie, 2020). Thus, these contacted particles are responsible for the network formation and expansion within the suspensions.
2.1.2 Constitutive relations for STSs
In shear thickening fluids such as STSs, the power low based on Smoluchowski equation (Wagner and Russel, 1989; Lionberger and Russel, 1997; Goral et al., 2010), can be used to determine their viscosity function (Eq. 2), where
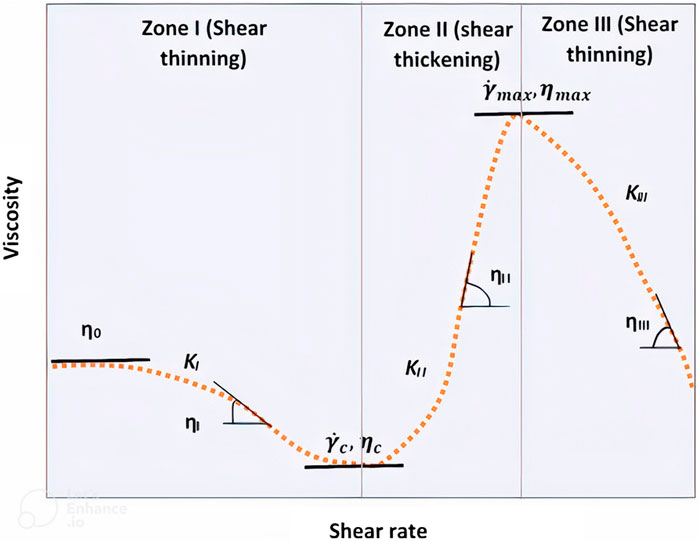
FIGURE 4. Typical viscosity curve of STFs. (Adapted from: Zarei, M.; Aalaie, J. Application of Shear Thickening Fluids in Material Development. J. Mater. Res. Technol. 2020, 9, 10411–10433, doi: 10.1016/j.jmrt.2020.07.049).
Several studies have been conducted to develop new functions in order to cover the other regions of the viscosity curve of STFs. Galindo-Rosales et al. (Galindo-Rosales et al., 2011), developed a function which can be used to fit the various regions of this curve. In the viscosity curve of STFs, three regions can be identified as indicated in Figure 4 (Zarei and Aalaie, 2020). The n-terms represents the slope of the curve for each area, η0, and ηmax indicate the initial and final viscosity and K-terms are time constants (Galindo-Rosales et al., 2011). On the basis of these definitions, a model based on three expressions (Eq. 3), was developed to capture the characteristics of the three regions (Galindo-Rosales et al., 2011):
This system was further improved (Galindo-Rosales et al., 2011; Vickers, 2017), by introducing λi notation for the time constant Ki, and changing the sign of this latter parameter to avoid negative values. Therefore λi = - Ki in the I and II region of the curve flow, while λIII = - KIII in the third one (Eq. 4).
The present model has been used to predict rheological behaviour of both single and multi-phase STFs with good results (Zarei and Aalaie, 2020). The rheological properties of these systems can be modified accordingly by varying various parameters (Sema, 2013; Gürgen et al., 2017). The volume fraction of the particles, i.e., the fraction of the total volume in relation to the particle volume, plays an important role in determining the rheology of STFs. In general, as the volume fraction of the particles increases, the shear thickening effect becomes stronger and vice versa (Gürgen et al., 2017; Zarei and Aalaie, 2020). Particles of different shapes and sizes, can lead to different rheological responses in STFs. The use of rod-shaped particles (Barnes, 1989) resulted in an increase in the shear thickening behaviour of STSs compared to other shapes. High aspect ratio particles can improve the viscosity of STFs due to the increased interaction between the particles in the flow (Wetzel et al., 2004). Other studies have shown that hydrodynamic forces are proportional to the cube of the greater dimension of the hydro cluster, explaining why elongated particles have a higher influence on the shear thickening behaviour of STFs than spherical ones (Bossis and Brady, 1989; Phung et al., 1996). The functionalisation of dispersed particles can influence the viscosity of suspensions (Zarei and Aalaie, 2020). Some researchers have modified silica particles with polydopamine (PDA) before dispersion in polyethylene glycol 200 (PEG-200) (Liu et al., 2016). Results suggested better shear thickening behaviour of the functionalised silica STF compared to the virgin one (Liu et al., 2016). This can be ascribable to the chemical structure of PDA, comprising a large number of both hydroxyl and amine groups, resulting in a higher affinity of functionalised silica for PEG than virgin silica particles. Furthermore, the large number of hydroxyl groups in functionalised silica, promotes interactions between particles and formation of clusters (Zarei and Aalaie, 2020). Another important parameter affecting viscosity of STSs is the carrier fluid (Lee et al., 2009; Gürgen et al., 2017; Zarei and Aalaie, 2020). Various studies report that higher molecular weight fluid can enhance viscosity of suspensions since the longer molecular chains hinder the adjacent fluid layers from moving (Khandavalli and Rothstein, 2014; Moriana et al., 2016). Furthermore, it has been reported (Zarei and Aalaie, 2020) that the use of PEG-600, for example, increases the shear thickening behaviour of STS compared to low molecular weight fluids. This phenomenon can be attributed to the high adsorption of this polymer on the surface of the particles, due to the chemical interaction between the polymer and particle functional groups and the long polymer chains promoting adsorption (Shenoy and Wagner, 2005). High molecular weight fluid improves shear thickening behaviour of suspensions, whether the fraction of dispersed phase is low (Baharvandi et al., 2016). STSs have been successful employed in various industrial fields such as in protection or electronical devices. Nevertheless, the instability of these suspensions and their unavoidable sedimentation have significantly limited their use in many of these applications (Wang et al., 2016a).
2.2 Shear thickening gels
Shear thickening gels (STGs), in general, refers to non-particle polymeric systems exhibiting interesting shock and vibration damping properties because of their particular crosslinked networks (Liang and Zhang, 2015; Lin et al., 2018; Zhao et al., 2020). Over the past few years, several companies have developed a wide range of STG-based products having different peculiarities. Viscoelastic polyurethanes such as Sorbothane® (Sorbothane, 2023b),Rheon® (Rheon, 2023) and Poron XRD® (Charkarska et al., 2008), polynorbornene-based polymers (Norsorex®) (Nickel et al., 2012), vibro-absorbent elastomers (Noene®) (Nickel et al., 2012) and silicone-based materials such as Polyanswer® (Polyanswer, 2023a) and D3O® (D3O, 2023a) are currently used as cushioning material to easily dissipate and absorbs impact forces. These materials are considered to be high-quality vibration damping materials, good acoustic insulators, and considerably long-lasting products.
One of the first man-made system based on this technology has been Silly Putty® now commercialized by DuPont under the trade name Molykote 3179 (previously Dowsil 3179). This dilatant compound was originally a toy made of polydimethylsiloxane, boric acid and other components. This material showed unusual behaviour as it was able to bounce and recover after breaking under a severe blow and even flow as liquid in certain conditions because of its viscoelasticity (Cross, 2012a). Because of their unique properties and easy preparation, polyborosiloxanes (PBSs) - have gained popularity within the research community and as materials for impact protection. From a rheological point of view, both the storage modulus (E’) and viscosity of these polymeric gels increase at high external strain rates mainly due to the reversible and irreversible interactions occurring between poly (dimethylsiloxane) (PDMS) and boric acid functional groups (Liu et al., 2014). Compared to the shear thickening suspensions, these latter gel systems based on siloxane-boron networks are more viscous and stable in terms of less sedimentation of the dispersed phase and chemical side reactions (Zhao et al., 2020).
2.2.1 Synthesis and main features of polyborosiloxanes
According to the literature, there are different synthetic routes to prepare polyborosiloxanes. All these methods can be grouped into two subcategories: solution and mass-phase synthesis. Regarding the first option, it has been reported the synthesis of structure-controlled PBSs starting from hydroxy-terminated PDSM precursors with different molecular masses and boric acid dispersed in a toluene solution to reduce the viscosity of the reactive system (Tang et al., 2016). The mixture was stirred at room temperature for 2 h and then heated to 120°C for 2 days to allow further reaction. Finally, raw materials were collected and purified in hexane to remove the unreacted boric acid. The whole reaction was followed with FTIR spectroscopy, taking samples from the reactive mixture at different times. The spectra of the resulting PBSs showed the presence of the characteristic absorption bands indicating the presence of Si(CH3)2 groups at 860 cm−1 and formation of Si-O-B bonds (1340 cm−1) as result of the successful condensation between OH- groups from both siloxane and boric acid. Furthermore, it was noticed that the elastic modulus of the resulting PBSs increased along with the molecular weight growth of PDMS precursors (Tang et al., 2016).
PBSs under milder conditions have been prepared from a solution of sodium tetraborate and dichlorphenylmethylsilane adding hydroxyl silicone oil after heating the mixture at 80°C for 12 h (Li et al., 2014). Reaction was followed through FTIR analysis and the chemical structure of PBSs was confirmed by Si-NMR spectroscopy. From the resulting spectra it has been deducted that the Si atoms of the existing units in the copolymers structures were present as (R2SiO) and (RSiO3/2) respectively. In addition, it was found the presence of a physical network structure within polyborosiloxanes and also that high temperatures increase the motion of the polymeric chains, resulting in fewer weak bonding interactions and thus a weaken network with a lower elastic modulus (Li et al., 2014).
Several studies have been also carried out about mass-synthesis methods. Liu et al. (2014) synthetized a PBS starting from trimethylsiloxy terminated linear poly (dimethylsiloxane) and boric acid at 200°C for about 6 h (Liu et al., 2014). They have developed a refinement process in which the polydisperse polar end groups that can be converted into predominately −Si−O−B(OH)2 boron groups, by hydrolysis of boroxane bonds (B−O−B) in order to obtain supramolecular elastomers thanks to hydrogen bonding among the end groups. Moreover, it was found out that the dynamic modulus increases significantly after the modification, as a consequence of the strong interactions between the modified chain ends (Liu et al., 2014).
Borosiloxane materials were obtained also by mixing PDMS hydroxy-terminated with boric acid and keeping the mixture at room temperature for 24 h (Mashchenko et al., 2020). According to this synthetic route, by changing the conditions of synthesis and introducing other components as plasticizers, it is possible to modulate the self-healing and elastic properties of borosiloxane-based materials in order to use them in certain application where the restoration of the material is required (Mashchenko et al., 2020). An efficient and timesaving way to prepare PBSs, involve the use of hydroxy-terminated PDMS and boric acid mixed for 90 min at 180°C (Mashchenko et al., 2020). The mixture formed a cross-linked, sticky and viscous gel during the heating process, exhibiting unique rate-dependent properties with the storage modulus growing with the increase of shear frequency. Furthermore, due to their viscoelastic deformation and shear thickening behaviour, the resulting borosiloxanes, showed great impact force and energy absorption (Mashchenko et al., 2020). In addition, network modification of PBSs has also been reported. In order to manage the cold-flow related issues of PBSs, different polymeric materials have been added to the reactive system to improve its elasticity and resistance. For example, a PBS gel was mixed with methyl vinyl silicone rubber (MVQ) and successively co-vulcanized to obtain a cured silicone rubber (Wang et al., 2018a; Zhang et al., 2020). The shear-hardening gel was homogeneously dispersed into the system due to the presence of covalent bonds and intermolecular forces as well, and according to the results, both the damping performance of MVQ/PBS rubber and its initial elasticity were significantly improved (Zhang et al., 2020).
Boron-containing adhesion promoters (PhBSiO and MeBSiO) were also mixed to a pure silicone resin in order to enhance its adhesive strength to plastic substrates such as epoxy resin, polypropylene, polycarbonate and polyphthalamide (PPA) widely used in the electronic industry (Pan et al., 2020). Wu et al. (Wu et al., 2019), have developed a highly stretchable elastomer with self-healing properties by mixing PBS and PDMS (Wu et al., 2019). The resulting system exhibited both a dynamic network due to boron-oxygen bonds and a permanent one because of PDMS crosslinked structure. As a consequence, the elasticity and the stability of this elastomer were improved, overcoming the common drawbacks such as fluidity and irreversible deformation of the conventional solid–liquid materials (Wu et al., 2019). Use of fillers in borosiloxones compounds has been also investigated since the use of different additives and fillers in PBSs is possible because of its chemical stability and inactivity. For example, calcium carbonate has been added to PBS to enhance its storage modulus (Zhao et al., 2020). Electromagnetic particles have been also successfully employed in order to make these materials suitable for a wider range of applications (Wang et al., 2016a; Fan et al., 2019). Magnetic shear stiffening gels were obtained by adding carbonyl iron particles and ferric oxide to PBS. The resulting materials exhibited a greater initial storage modulus as well as improved mechanical properties under magnetic field (Wang et al., 2014; Fan et al., 2019). Moreover, enhanced thermal self-healing properties have been observed in many of these systems, in which, thanks to their unique recovery process, even cut samples of PBS are able to reconnect (Wang et al., 2018a; Zhao et al., 2020).
Fe-Co-Ni nanowires were also used as additives to prepare magnetic shear thickening gels and also in this case an improvement of the storage modulus has been observed under a magnetic field (Golinelli et al., 2015). Further studies have been carried out in this field, investigating the effect of different fillers such as carbon black (Zhang et al., 2018), Ag nanoparticles (Wang et al., 2018b) or carbon nanotubes (Wang et al., 2015) on the mechanical and electromagnetic properties of the resulting materials. The results showed that in some cases the presence of a magnetic filler could improve the rheological properties of these modified PBSs also affecting their electric performances (Zhao et al., 2020).
From a rheological point of view, PBS-like gels in their natural condition behave as perfectly viscous material, with the Loss Modulus (E″) exceeding the Storage one (E′) at low strain rate. Their elastic modulus and thus their rigidity grow as the applied shear stress increases up to a critical stage; from this point on, a viscoelastic transition between (E″ and E’) has been observed within the system with the elastic modulus overcoming the loss modulus (Liang and Zhang, 2015; Rudolf et al., 2016). The viscous character which dominated at low shear rate, becomes less evident after crossing the transition point and the whole system shows its shear-stiffening character behaving as a high elastic material (Zhao et al., 2020). Tests performed on PBS using SHPB technology, have shown that these polymers can go from an elastic state to a glassy state under a high strain rate (14,132 s−1) where the material becomes highly resistant and brittle (Jiang et al., 2014). The rheological behaviour of such materials has been studied through various models. Cross et al. (Cross, 2012a; Cross, 2012b), developed a standard linear solid model based on the combination of spring element in series with a classical Maxwell element to describe the visco-elastic properties of shear thickening gels such as PBSs. In order to justify the fracture mechanism of PBSs, the SLS models has been further modified by adding another series of Maxwell element (Wang et al., 2018a). Experimental results were in line with theoretical data. Other researchers, used a ternary Maxwell parallel model to study temperature dependence of visco-elastic PBSs (Goertz et al., 2009).
2.2.2 PBSs: structure and mechanism of action
According to various research studies, polyborosiloxanes are classified as supramolecular elastomers (Liu et al., 2014), and their dilatant properties depend not only on covalent bonds but also on non-covalent physical interactions (Tang et al., 2016). In these materials, the constituted Si-O-B bonds act as branched points whereas the supramolecular interactions are mainly due to the hydrogen-bonding of Si-O-B(OH)2 end groups and the Si-O:B weak bonds (Scheme 1C) (Li et al., 2014; Liu et al., 2014; Tang et al., 2016). For PBSs obtained from PDMS precursors with high average molecular masses, topological entanglements might be also present in the resulting system and act as physical crosslinks (Scheme 1B), affecting both the mechanical and the rheological properties with, as an example, an increased elastic modulus (Tang et al., 2016).
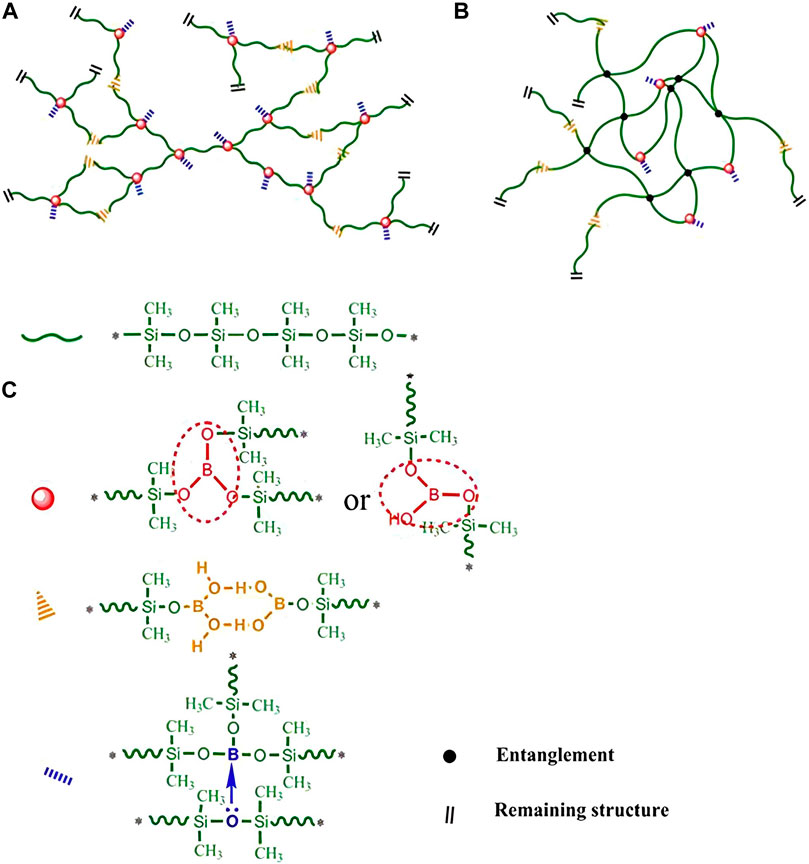
SCHEME 1. Illustration of the chemical structures and physical interactions for PBSs. PBS obtained from precursors with low (A) and high (B) molecular masses (C) Reversible and irreversible interactions within PBS structure. (Adapted from Tang M, Wang W, Xu D, Wang Z. Synthesis of structure-controlled polyborosiloxanes and investigation on their viscoelastic response to molecular mass of polydimethylsiloxane triggered by both chemical and physical interactions. Ind Eng Chem Res. 2016;55(49):12582–9).
Si-O:B weak bonds are due to the ionic nature of boron oxides. Indeed, boron has 2p orbitals able to accept a pair of electrons from the oxygen of the silicone backbone, thus creating a sort of electron bridge with the adjacent molecules (Li et al., 2014; Zhao et al., 2020). As a result, physical crosslinks are formed via temporary and thermally reversible B-O dative bonds and, depending on the different amount of boron, the final polymer can achieve certain unique properties (Li et al., 2014). Academic results have suggested that variation in boron content can significantly affect the tendency of the elastic modulus of the material. As a matter of fact, weak-bonding interactions are remarkably present in boron-rich samples, implying more network structures due to physical crosslinks, thus leading to an increase in the conservative modulus (E’) with relatively high values (Li et al., 2014).
The Fourier transform infrared spectroscopy (FTIR) techniques have been widely used to investigate the presence of cooperative bonding between boron and oxygen. Typical stretching vibrations (Figure 5) of Si-CH3 (1260 cm−1), Si-O (1020–1090 cm−1) and Si-O-B (1340 cm−1) have been found in the resulting PBSs (Tang et al., 2016). Nevertheless, with the strain rate increasing, the timescale for the polymer chain motion become shorter than the necessary time for B-O networks to break and these bonds represent the main obstacles to the movement of molecular chains (Zhao et al., 2020). With most of the polymeric chain motions being hindered, only the rotation is responsible for the mechanical properties of PBSs, resulting in an enhanced stiffness for the material at high strain rate (Goertz et al., 2009; Cross, 2012b; Li et al., 2014). Furthermore, at the above-mentioned condition, a shift from a rubbery state to a brittle glassy phase has been also noticed (Zhao et al., 2020).
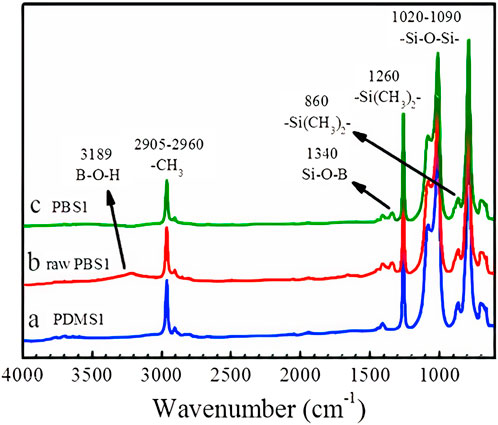
FIGURE 5. Typical FT-IR spectra of PBS and PDMS precursor. (Adapted from: Tang, M.; Wang, W.; Xu, D.; Wang, Z. Synthesis of Structure-Controlled Polyborosiloxanes and Investigation on Their Viscoelastic Response to Molecular Mass of Polydimethylsiloxane Triggered by Both Chemical and Physical Interactions. Ind. Eng. Chem. Res. 2016, 55, 12582–12589, doi:10.1021/acs.iecr.6b03823).
In correspondence of the critical point of the material where the stress reaches the yield point, all the crosslinks are broken and thus, the whole structure easily cracks (Wang et al., 2016a). It has been reported that PBS-like materials possess unique self-healing properties, precisely because of their reversible and active B-O bonds, which are able to reconnect after breakage whether they are close enough to each other (Martin et al., 2015). With the shear thickening effect and fracture failure acknowledged as the main mechanism behind the impact dissipation properties of PBSs, in the past few years, these materials have been broadly used in all the industrial applications where shock absorption and reduced vibrations were necessary (Zhang et al., 2020).
3 STFs in sport protection applications
3.1 Use of STSs in sport products
Several polymeric materials used in various chemical and industrial applications do not adhere to the conventional Newtonian viscosity postulate and therefore are classified as non-Newtonian fluids. Several studies have been conducted on the preparation of high-performance sport products such as rackets, snowboards, footwear, head and body protectors with the aim to provide the best protection for their users. An athletic racket containing STSs has been developed in order to be used in various sport as tennis, badminton, paddle or squash (Lammer et al., 2012). The application of such materials, improved the product performance during the aforementioned games as well as the shear thickening behaviour of the suspension dissipated the energy and vibration generated by the ball impacts. Thus, the STSs acted as an energy absorbing barrier between the impact region and the user’s arm (Lammer et al., 2012). Furthermore, the application of shear thickening materials in other sport products has been shown to improve the user performances and safety as well. Introduction of STSs in footwears can maximize the impact absorption between user’s shoes and the surface, protecting foot from injuries against sharp objects or ground surfaces (Zarei and Aalaie, 2020). It has also been reported that the use of STSs in skis or snowboard can improve both the energy absorption and reduction of vibrations (Lammer et al., 2012). Several sport products including goalkeeper gloves (YUDONG, 2021), joint-saving sleeves (Holt and Perez, 2015), protective apparel (Witek et al., 2020) and athletic tape (Holt and Perez, 2015) using impregnated or filled fabric with shear thickening fluids have been developed. Different fibrous/porous materials such as nylon, cotton, polyester (Gürgen et al., 2017; Zarei and Aalaie, 2020) and even polymeric foams impregnated with STSs have been employed in such padded protections (Dawson et al., 2009; Soutrenon and Michaud, 2014). Incorporation of Non-Newtonian fluids in sport gears surrounding the joints ensure protection for soft tissue against unexpected twisting, abrupt joint stress and violent shocks, decreasing the risk of injuries related to the instability within the knee, ankle, and elbow (Holt and Perez, 2015). In another research, a smart hip protector has been created by dispersing fumed silica particles into PEG and incorporating this fluid into a conventional foam for the production of such devices. Under impact, the filled foam showed a decreased peak force absorbing 7.4 times more energy than the unfilled foam, thus demonstrating that the presence of STSs enhanced the overall safety performances of conventional hip protectors (Barlaz, 2010).
Various sports helmets containing STSs have also been patented during the recent years. In particular, one or more layers of fluid polymer (such as a shear thinning and shear thickening polymers) have been incorporated between the user’s head and a hard helmet shell (Plant, 2009; Frias et al., 2017; Pannikottu and Abraham, 2017; Plant, 2020). A protective football helmet with a Non-Newtonian fluid liner system has been made with an external shell possessing an outer surface and an inner surface defining a shell interior comprising a pad system. The Non-Newtonian fluid was disposed into the bladder interior (Morgan and Morgan, 2022). Furthermore, a system comprising a helmet and a protective carapace has been developed in order to decrease the force due to collision so that brain is protected against severe injuries. Physical communication between the helmet and shoulder pads has been achieved by the means of a force bearing member including a non-Newtonian dilatant suspension (Metts and Gower, 2021). Scientific evidences showed that protective liners combined with non-Newtonian fluids provided increased control in energy dissipation over several impacts (Spinelli et al., 2018), mitigating pressure of collision and impulse compared to standard foamed liners (Mears, 2019). In some cases STSs can even improve the impact responses of materials providing comparable results with commercial available products (e.g., kneepads) with dilatant behavior (Kaewpradit, 2021). Regarding face protection, a dental appliance has been also reported to protect users against injury from impacts occurring in sports and other leisure activities responsible for teeth damages. The device containing an energy absorbing fluid made of suspended particles in a viscous media showed a shear thickening behaviour dissipating both horizontal and axial forces upon impact reducing the risk for dental injuries (SCHWANK et al., 2017).
In general, over the past few years, devices based on STSs, have shown enhanced levels of protection, flexibility and comfort compared to the standard protective equipment. However, many of the reported applications have suffered from several drawbacks mainly due to the instability of the STSs. These disadvantages included evaporation, sensitivity to humidity, sedimentation or leakage of carrier fluids and reduced air and moisture permeability, which have seriously affected the overall performances of STS-based devices (Ding et al., 2013). Hence, in order to overcome these limitations, novel engineered materials based on more physically and chemically stable crosslinked structures such as polyborosiloxanes gels have been developed and proposed for impact protection devices.
3.2 Use of STGs in sport products
3.2.1 STG - hybrid composites
STGs have been widely used to realize shock absorbing composites, because of their great shear stiffening properties at high strain rate. Shear thickening gels have been added into porous matrices (foams and fabrics) in order to enhance their mechanical properties and energy absorption (Zhao et al., 2020). Considering their chemical inertness and low viscosity under low shear stress, these polymeric gels can be introduced into a porous scaffold to produce a stable system. According to the scientific literature on this topic, the first attempt has been made with a polyurethane (PU) foam impregnated with a PBS gel using a “dip and dry method” (Wang et al., 2016b). The resulting hybrid system exhibited great shear stiffening effect, enhanced creep resistance and adhesion properties. It has also been reported that storage modulus of the composite system compared to that of standard PU foams was perfectly adaptable and could increase even 3 orders of magnitude with the shear frequency applied, reaching a maximum of 1.55 MPa (Wang et al., 2016b). Moreover, the resulting system showed also great damping properties by reducing the impact force of 2 orders of magnitude even under consecutive impact cycles without undergoing degradation. Other porous structures such as polyurea-urethane (PUU) based foams were also reported. In this case the structure of PBS has been modified in order to achieve high flexibility and long-term stability (Martin et al., 2015).
A lightweight and flexible hybrid system with both anti-impact and electromagnetic shielding performances based on a melamine/MXene/PBS sponge has also been developed (Sang et al., 2021). The foam has been firstly dipped into a solution of MXene and subsequently immersed into an ethanol solution of PBS containing Benzoyl Peroxide (BPO). Finally, the resulting sponge has been vulcanized to obtain a nanocomposite system to be integrated into a safety sport protective equipment. The resulting system showed excellent energy dissipation ability by spreading the external impact force as a result of the shear stiffening characteristic of PBS and great self-healing properties by resisting to repeated external damages (Sang et al., 2021). A method to make auxetic closed cell foam that is infused with shear thickening gel has also been presented (Parisi et al., 2023). Auxetic closed cell foam has been shown to frequently outperform conventional foam in impacts of various severity, with peak forces in unconverted foam reaching double those in auxetic foam. Results were in line with a non-linear viscoelastic contact model developed by authors, which demonstrated accurate prediction of peak impact force, within 20% of experimental data (Parisi et al., 2023). In another academic work, the impact-protection ability of a novel STG hybrid composite based on PDMS was investigated according to the EN 1621–2CE standard for assessing back protectors (Liu et al., 2022a). As the STG content increased, the energy absorption efficiency at the same impact velocity gradually increased up to 85.97% at an impact speed of 4.43 m s−1, showing excellent performance in the protection of impacts (Liu et al., 2022a). Eco-friendly hybrids based on cork and STGs have been developed for head protection and subject to impact tests of 20 and 100 J (less and lower than the 65J expected by the EN 1078 norm for bicycle helmets) (Serra et al., 2022). Authors compared composites of the same thickness and dimensions, determining the influence of the material supplementary to cork in the impact performance. Hybrids with STF displayed a reduction up to 8.5% in peak acceleration for a given amount of energy absorbed per unit volume, being a potential solution for helmets used in micro-mobility.
A sensing-shock absorber kneepad has been also created by replacing a part of the inner cushion of the device with a conductive carbon black/STG/PU sponge. The obtained kneepad traced the basic motion of users with an extremely quick response and reduced the impact force by more than 40% compared to the unmodified kneepad (Zhang et al., 2018). On the basis of these promising results, this novel product has been suggested for the use in sports protection applications and signal feedback for athlete training (Zhang et al., 2018). Moreover, conductive STGs can be applied in wearable anti-impact hand protection because of flexible safeguarding performance and fast self-feedback response to external loading (Wang et al., 2017; Zhao et al., 2020).
On industrial-scale the most produced material for impact protection based on STGs has been D3O® which possess a shock absorption parameter several times superior to that of a conventional polyurethane foam that is twice as thick (Jachowicz and Owczarek, 2020). D3O is currently used in several personal sport protective equipment (PPE) because of its great flexibility, high comfort and unique safety performances as also claimed by the company (D3O, 2023a). Research conducted on this material has showed how D3O is able to absorb a greater amount of energy than its standard counterparts, lowering the peak of the transmitted force and stiffening in a very short time when hit by a high speed impact in real applications (Venkatraman and Tyler, 2016; Tang et al., 2017; Shargawi et al., 2022; Shargawi et al., 2023). D3O has first been produced in 2005 (Wang et al., 2013) and since its launch on the global market, several products based on a similar technology including Polylyanswer® (Ashokkumar et al., 2010) products have been developed and commercialized for impact protection purposes. Basically, D3O® is an elastomeric foam made of a branched polymeric network incorporating PBS particles. The physical interaction of the polymer chains grafted or adsorbed to the surface of the above-mentioned gel particles maintains them respectively separated helping stabilizing the shear thickening gel (Wang et al., 2013). The producers report that under low-speed stress, the material is soft and flexible as its molecules are able to slide over each other because of the presence of weak bonds within the polymeric chain. Nevertheless, under a high-speed impact the molecules re-arrange in a well-organized structure creating hydrogen bonds within milliseconds (Wang et al., 2013). Hence, in case of a shock event the shear stiffening gel particles lock together and the whole material become hard, absorbing the kinetic energy of the impact.
3.2.2 STG-based personal sports protective equipment
STG-based materials such as those used in many of the D3O® (D3O, 2023a), Sorbothane® (Sorbothane, 2023a) and Rheon® (Rheon, 2023) products, for example, are nowadays used as materials of choice for most of the production of PPE, in order to prevent severe injuries while maximizing the comfort performances. Shargawi et al., tested three different D3O back protector models both quasi statically and dynamically. As mentioned by these authors, D3O® material exhibited a higher energy absorbency level (p = 0.00)and a higher dampening ratio compared to traditional silicone-based materials, overall showing potential damping both to impact loading under low-frequency and high-impact loading (Shargawi et al., 2022; Shargawi et al., 2023). In another study (Nicotra et al., 2014), several back-protectors from different commercial brands including dilatant-foam based ones, have been studied to investigate the thermo-mechanical and impact properties of materials used for such devices in order to understand the mechanism of action of such hybrid systems used for protective equipment (Figure 6). According to the results from impact tests performed at 20°C on the aforementioned samples (Figure 7A), the dilatant-foam based protectors (samples 1-2-4) and standard soft-shell device (sample 3) present three regions in the impact curve, typical of visco-elastic foams.
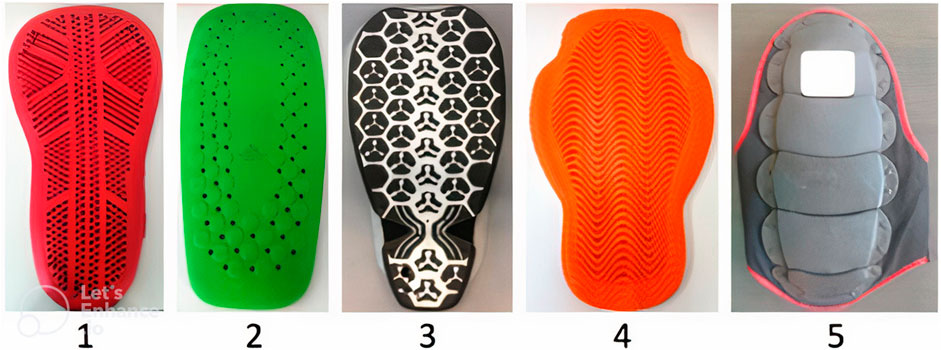
FIGURE 6. Tested commercial back protectors. (Available from Nicotra M, Moncalero M, Messori M, Fabbri E, Fiorini M, Colonna M. Thermo-mechanical and impact properties of polymeric foams used for snow sports protective equipment. Procedia Eng. 2014; 72: 678–83. Available from: http://dx.doi.org/10.1016/j.proeng.2014.06.115).
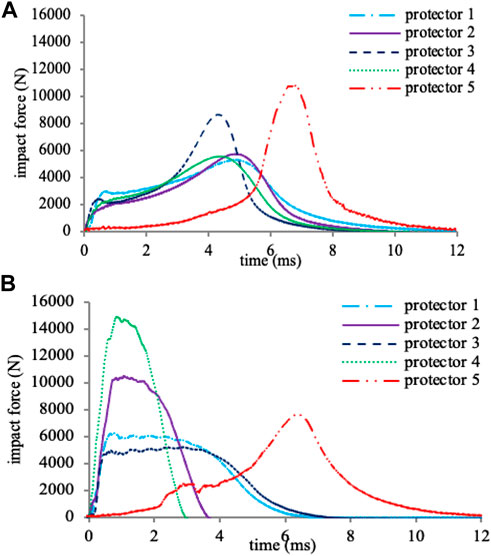
FIGURE 7. Results of the impact tests performed on the different protectors at 20°C (A) and −5°C (B). (Available from Nicotra M, Moncalero M, Messori M, Fabbri E, Fiorini M, Colonna M. Thermo-mechanical and impact properties of polymeric foams used for snow sports protective equipment. Procedia Eng. 2014; 72:678–83. Available from: http://dx.doi.org/10.1016/j.proeng.2014.06.115).
Firstly, a linear elastic region (ascribed to cell wall bending and stretching) followed by a plateau area (due to non-linear elastic buckling). These two regions are separated by a clear yield point. Finally, densification is reached and the force increases sharply (controlled by collapse of cell walls) with a higher peak force for the standard soft-shell protector (Nicotra et al., 2014). The non-dilatant rigid-shell sample (protector 5) behaves as a typical rigid polymer with a high impact force concentrated in a short time. As also demonstrated by DMTA analysis, dilatant-based and soft-shell materials are strongly temperature dependent while the hard-shell protector is less affected. Therefore, this latter has not exhibited a significant change under impacts at low temperature such as the other protectors since the mechanism of impact protection is performed by energy dissipation over a greater area, without a viscous absorption of the impact (Figure 7B) (Nicotra et al., 2014). The above-mentioned samples have also been tested under multiple impacts (Figures 8A, B) and results suggest that the hard-shell protector (sample 5) has a sensible increase in the peak force after multiple impacts due to the yielding effect of the hard material under repeated impacts (Nicotra et al., 2014). Furthermore, the fractures have occurred in the external part of the device at low temperature confirming its impossibility to be used in multiple-impact applications.
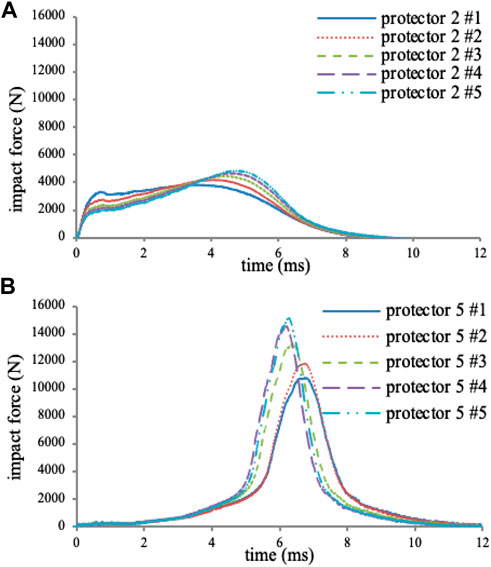
FIGURE 8. Multiple impact at 20°C performed on soft-shell protector 2 (A) and hard-shell protector 5 (B). (Available from Nicotra M, Moncalero M, Messori M, Fabbri E, Fiorini M, Colonna M. Thermo-mechanical and impact properties of polymeric foams used for snow sports protective equipment. Procedia Eng. 2014; 72:678–83. Available from: http://dx.doi.org/10.1016/j.proeng.2014.06.115).
Analytical models and finite element methods (FEM) simulations have also been proposed in order to rationalize experimental data from mechanical and impact tests (Signetti et al., 2019; Liu et al., 2022b). Signetti et al. (Signetti et al., 2019), developed a model to describe the impact process of shear stiffening gel-base protectors in the drop weight tests. They started from a differential equation describing the frictionless impact between two solid bodies, without any vibration as in the case of soft materials, according to the equation:
In which w(t) is the displacement of the substrate in the central area of the impact area, m is correlated to the masses of the impactor and substrate, c is a coefficient of the viscous damping and k is the contact stiffness of the substrate when a flat impactor is used. This latter depends on the radius R of the impactor and on both Young’s modulus E and Poisson’s ratio
FEM simulations were conducted reproducing the experimental setup for impact tests, using a cylindrical plate to represent the protectors and changing the properties of the materials according to values obtained from mechanical tests (DMTA) previously performed on protectors. Theoretical results were in line with the experimental data, thus demonstrating how soft-shell and dilatant devices provides better protection compared to hard-shell solutions. Moreover, the analytical-simulation approach could be used to predict the impact behaviour of such equipment from material characterization, saving costs and time for design and optimization (Signetti et al., 2019). The same authors have also reported that the dilatant foams can be highly perforated while maintaining good impact properties, thus permitting a high thermal and moisture exchange that provide an excellent thermal comfort for this class of body protectors (Dotti et al., 2016).
In the recent years, research has been focused on the application of dilatant hybrid composites especially for the head protection. Jachowitz and colleagues conducted experiments to assess three different commercial foamed materials with non-Newtonian behaviour as potential inserts for protective helmets subjecting them to impact at varying temperatures (Jachowicz and Owczarek, 2020). Their findings revealed that, within the examined density range (235–336 ± 1 kg/m3), energy suppression (Es) in the samples remained constant irrespective of density but was influenced by temperature. The peak force value exhibited the most rapid changes at sub-zero temperatures (mainly at −30°C) and changed the least at 50°C. Notably, the highest-density sample showed the most significant changes at −30°C. Consequently, taking into account the typical temperature range for protective helmets, spanning from −10 (or optionally −30) to 50°C, materials of higher density will provide enhanced protection without significantly affecting user’s comfort (Jachowicz and Owczarek, 2020).
Another comparative study on football helmets has been conducted (Zuckerman et al., 2019). Authors have investigated the impact performances of a commercial helmet using two STG-like materials as outer shell (Dow Dilatant Compound and Sorbothane) placed in the front boss, side and back critical areas of the head. Comparted to the standard helmet, the prototype with the Sorbothane outer shell reduced linear acceleration by 5.8% (from 75.4 to 71.1 g; p < 0.001) and 10.8% (from 89.5 to 79.8 g; p = 0.033) at the side and front boss locations, respectively, and reduced rotational acceleration by 49.8% (from 9312.8 rad/s2 to 4671.7 rad/sed2; p < 0.001) at the front boss location, thus resulting in an overall improvement of safety performances (Zuckerman et al., 2019). In a more recent work, five commercial headgears (both with Newtonian and non-Newtonian behaviour) approved by the World Rugby, have been impacted at varying speeds between 1.7 and 3.4 m*s and five different angles on the surface. In comparison to not wearing any headgear, all tested headgear significantly reduced both linear and rotational accelerations, with a more substantial reduction by up to 50% and 60% respectively, for Non-Newtonian headgears (Stitt et al., 2022). Shock-absorbing effectiveness of viscoelastic polymer in such head protections was also observed also by Ganly et al. in their research. Authors outlined that the N-Pro viscoelastic head guard reduced linear impacts by 75% compared to a commercial rugby head guard and maintained its impact energy reduction even after repeated impacts. It also reduced rotational impact energy by an average of 34% across various test conditions compared to bare head-forms (Ganly and McMahon, 2018).
Several sports companies have started to use these materials for the production of their sport helmets. Leatt® company, for example, uses a dilatant foam system for the design of turbine-shaped units (Figure 9) (Siegkas et al., 2019) which are connected to the head (LEATT Turbine 360°) (LEATT et al., 2015; LEATT, 2020). With regards to such systems, Siegkas et al. conducted a study combining real-world impact tests and computer simulations to assess new viscoelastic helmet components effectiveness in reducing brain forces during head impacts (Siegkas et al., 2019). The viscoelastic turbines were created by injection moulding Rheon® material. These components were then affixed to the interior liner of a high-end motorcycle helmet. Testing both equipped and non-equipped helmets under realistic conditions, authors found significant reductions in peak accelerations, primarily for frontal impacts, reaching up to 27% reduction in mean translational acceleration (p = 0.017) and 22% reduction in mean rotational acceleration (p = 0.03). These values were below the threshold of the AIS 2 head injuries (Siegkas et al., 2019). These components decreased also strain and strain-rate for low-speed frontal impacts, demonstrating their potential in improving helmet protection and mitigating brain tissue deformation.
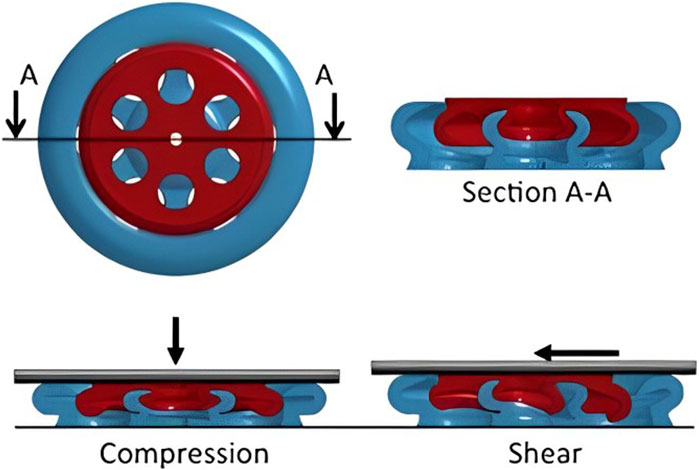
FIGURE 9. Turbine-shaped units and their components fitted together (small and large revolver), exhibiting different responses in compression and shear loading. (Available from Siegkas P, Sharp DJ, Ghajari M. The traumatic brain injury mitigation effects of a new viscoelastic add-on liner. Sci Rep. 2019; 9 (1):1–10).
Others producers use STGs encapsulated in flexible bags. In particular, Fox Racing® and POC Sports® employ the products Fluid Inside system and Spin system respectively, developing helmets which comprise capsule of STG placed on the inner liner in direct contact with head (Fluid Inside, 2023; Fox Racing, 2023; POC Sports, 2023). Nevertheless, the low friction with hairs or sweat on the human head might affect the energy dissipation upon impact since this system is in direct contact with the user’s head. Interestingly, none of the mentioned system is able to provide mobility between the inner and outer part of the helmet while dissipating energy in all directions during this movement. More recently, a 3D printed unit for a motorcycle helmet, made of a rigid shell containing a STG with a dipped pad, free to move in all direction, has been patented (Figure 10A) (Colonna et al., 2021). The top of the unit is closed with a flexible cap and fixed to the outer part of the helmet whereas the bottom is connected with the inner part of the helmet. The connection between the inner and outer part of the helmet is secured by a series of these above-mentioned pad units and therefore is able to rotate (Figure 10B) (Colonna et al., 2021).
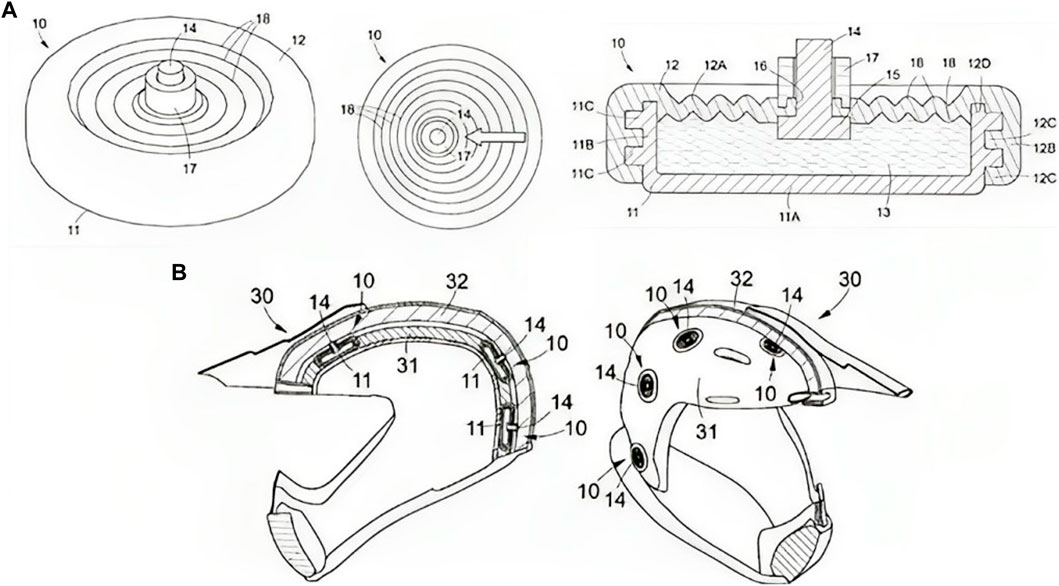
FIGURE 10. Illustration of the 3D printed pad containing the STG and its parts (A) and of the positioning of the pads in the helmet (B). (Available from: Martino C, Mariafederica P, Giuseppe L fauci. DEVICE FOR ABSORBING SHOCKS ON HELMETS AND CORRESPONDING HELMET. Italy; WO2023017552A1, 2021. p. 19).
From the results of impact tests conducted on the pad units using an experimental set-up that selectively mimics the tangential impacts at 1.25 and 2.50 J, it has been found that the presence of the STG is able to dissipate the tangential impact energy responsible for most of the head concussions (Figures 11A, B) (La Fauci et al., 2023). In particular, this fluid is responsible for the reduction of the peak force and increase of the time-to-peak meaning that the impact is spread over a wider range of time and a lower force is transmitted to the human head. It has been reported that the use of a dilatant fluid such as a borosiloxane compound can help decreasing the transmitted impact force from 55 up to 80% (depending on the impact energy) compared to a standard system in which the inner and outer parts are rigidly connected, postponing the time to peak to more than 10 milliseconds (Figure 11A) (La Fauci et al., 2023). However, this novel system is still at a developing stage, thus an optimization of the unit design as well as the employment of STGs with different properties such as a PBDMS with a different boron content, should provide different threshold energy values at which the pad is able to efficiently absorb the tangential impact energy.
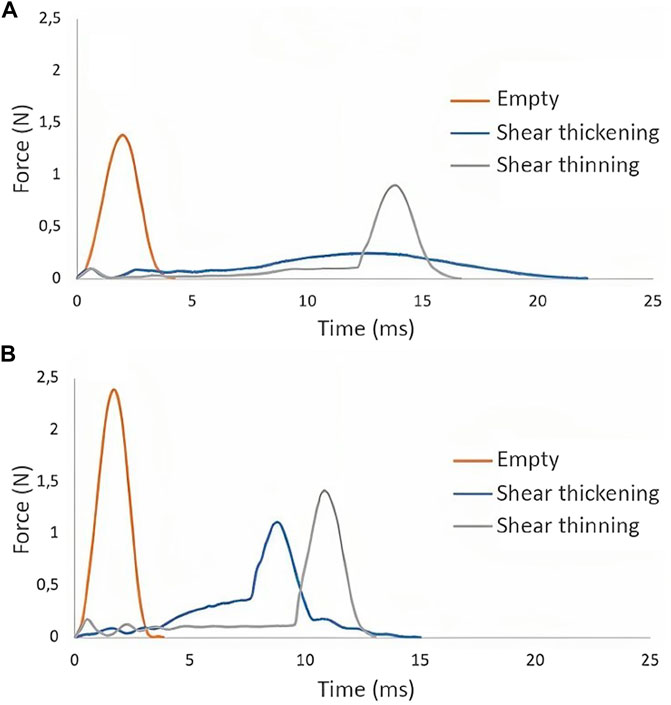
FIGURE 11. Measured transmitted force for the empty and filled experimental set-up that selectively mimics the tangential impacts at 1.25 J (A) and 2.50 J (B) (Available from La Fauci G, Parisi M, Nanni A, Crosetta L, Pugno NM, Colonna M. Design and proof-of-concept of an advanced protective system for the dissipation of tangential impact energy in helmets, based on non-Newtonian fluids. Smart Mater Struct. 2023;32(4):044004).
Building upon the example of STF material’s application in sports protection devices, it is essential to explore the broader implications and advantages of this innovative technology. The cost-effectiveness of STF-based sports protection gear compared to traditional alternatives can vary depending on several factors. STF-based gear is designed to provide superior protection and injury prevention by leveraging its unique ability to stiffen upon impact (Nicotra et al., 2014; Venkatraman and Tyler, 2016; Tang et al., 2017; Ganly and McMahon, 2018; Siegkas et al., 2019; Signetti et al., 2019; Zuckerman et al., 2019; Jachowicz and Owczarek, 2020; Shargawi et al., 2022; La Fauci et al., 2023; Shargawi et al., 2023). This characteristic holds the potential to reduce the risk of injuries and associated costs related to medical treatments, rehabilitation, and long-term healthcare (Peterson et al., 2021). Moreover, STF-based gear tends to be more durable and long-lasting than traditional options (Nicotra et al., 2014; Wang et al., 2018a; Ganly and McMahon, 2018; Wu et al., 2019; Mashchenko et al., 2020), thus contributing to lower long-term equipment costs, as athletes may not need frequent replacements. This heightened safety can also have a positive impact on athletic performance, potentially leading to greater success and financial gains for athletes or teams (Qiu, 2020). Additionally, the customization potential of STF-based gear with additive manufacturing (AM) as will be discussed in the following section, could open up to personalized fits, enhancing comfort and performance while concurrently reducing the risk of injury (Cazón-Martín et al., 2019; Beiderbeck et al., 2020). As reported in the study conducted by Cazón and colleagues (Cazón-Martín et al., 2019), embracing a multi-material AM approach for crafting shin pads may initially appear relatively expensive, with a cost of approximately €100 (USD 116) for a single pad, accounting for 3D printing materials alone. This cost noticeably contrasts with commercially mass-produced shin pads, commonly priced between €5 (USD 6) and €50 (USD 60). However, in comparison to custom-made shin pads, frequently often crafted from carbon fiber and valued at €600 (USD 697), the AM shin pad emerges as a demonstration of substantial cost-effectiveness. Hence, despite the higher initial cost associated with STF-based gear, its inherent capacity to provide prolonged protection, durability, and customization holds the potential for lasting financial savings. This attribute renders it a compelling choice for athletes and teams in search of an ideal equilibrium between safety and economic efficiency.
3.2.3 Additive manufacturing of STG-like materials in sport applications
Additive manufacturing, commonly known as 3D printing, has rapidly narrowed the gap between designing, prototyping, and final production (Mora et al., 2022). This innovative technology is now widely employed to create essential commercial components that significantly contribute to the functionality of various products, from automotive (Abayazid and Ghajari, 2020) to lattice structures designed for energy absorption (Ozdemir et al., 2017; Khosroshahi et al., 2018; Khosroshahi et al., 2019; Hössinger-Kalteis et al., 2023). Example materials used for AM include rubbers, plastics, metals and ceramics despite these latter being more incompatible with sporting equipment (Duncan et al., 2018). Recently, the technology of photopolymer jetting, also known as PolyJet or material jetting, has emerged as one of the swiftest and most promising additive manufacturing methods. Within the PolyJet process, precise deposits of photopolymer resins are applied as voxel-based droplets onto a print bed, subsequently solidified under ultraviolet (UV) light (Abayazid and Ghajari, 2020; Mora et al., 2022). Since resin can be deposited before curing, various materials with different polymerized properties can be deposited from the same nozzles, enabling multi-material part production (Ozdemir et al., 2017; Khosroshahi et al., 2018; Hössinger-Kalteis et al., 2023). Developments in materials science have introduced innovative flexible and shear sensitive elastomers, such as Agilus30 (A30) and Tango+ (T+), to the realm of PolyJet technology (Abayazid and Ghajari, 2020). These groundbreaking photopolymer resins have significantly elevated the mechanical flexibility of PolyJet elastomers, making them invaluable for a range of technological applications (Abayazid and Ghajari, 2020), including soft wearable devices (Han and Lu, 2018), shape memory materials (Kantareddy et al., 2016; Mao et al., 2016; Wu et al., 2016; Akbari et al., 2018), composites (Dimas et al., 2013; Wu et al., 2016), and various types of metamaterials (Che et al., 2018; Dykstra et al., 2019).
A recent work delved into the behavior of A30 and T + PolyJet elastomers, considering a range of loading conditions, strain rates (0.003/s to 0.5/s), and orientations (Abayazid and Ghajari, 2020). Authors introduced a visco-hyperelastic model to effectively anticipate how these materials would respond mechanically. Both elastomers showed nonlinearity and sensitivity to strain rate, with A30 notably exhibiting a 67% increase in tensile stress at 50% strain compared to T+ across varying strain rates (Abayazid and Ghajari, 2020). The relationship between build orientation and strain rates revealed a consistent tension trend, with anisotropy diminishing at higher strain rates (0.3–0.5/s). The visco-hyperelastic model accurately predicted the mechanical behavior of both elastomers, yielding errors between 1.3% and 29.9%, depending on strain rate and orientation (Abayazid and Ghajari, 2020). In another study, the mechanical properties of 3D-printed Digital Material DM40 (Agilus30 and VeroClear) were examined at varying strain rates (Boualleg and Cirkl, 2022). The material exhibited a nonlinear visco-hyperelastic response, influenced by both strain and strain rates (Boualleg and Cirkl, 2022). Researchers developed a visco-hyperelastic material model based on shear test data, used it in finite element analysis to predict compressive stress responses in cylindrical samples under various strains, and found a strong match with experimental results. Further studies have been carried out for characterizing rate-sensitive materials for AM. Liravi et al. developed a model to be used for highly viscous non-Newtonian fluids, such as polysiloxane-based materials, suitable for AM nozzle dispensing systems (Liravi et al., 2017). Their model proved useful in identifying the optimal combination of pressure value, pressure time, and working distance to ensure proper flow over the substrate while preserving the uniformity of the printed feature.
Several efforts have already been done to utilize AM with shear-sensitive materials in the production of sports protective equipment.
Abayazid et al. conducted groundbreaking research on viscoelastic circular cell honeycomb arrays, investigating the influence of curvature in cell walls on their mechanical response during oblique impacts (Abayazid et al., 2022). Using detailed finite element models of Agilus-based honeycombs, they validated their findings with a new testing rig. Their study revealed that adjusting curvature could significantly enhance shear and compressive responses, with concave cell arrays exhibiting up to 300% higher shear stresses during oblique impacts (Abayazid et al., 2022). Additionally, higher impact velocities increased compressive stress and internal energy by as much as 200% (Abayazid et al., 2022), making these honeycombs suitable for applications involving variable impact velocities, such as head collisions.
Boopathy et al. explored the energy-absorbing potential of Vero White and Tango + material combinations under static and dynamic loads using PolyJet 3D printing to create various honeycomb structures (Rajendra Boopathy et al., 2019). They found that a five-layered (5L) sandwich multi-material honeycomb outperformed single materials in absorbing impact loads, with a minimum force of 1,948.17 N compared to 5,055.24 N of single materials (Rajendra Boopathy et al., 2019). These results suggest promising applications for these systems in improving the crash resistance of safety components like helmets, knee guards, and car bumpers. Another study has proved the feasibility of using multi-material 3D printing for shin pad production with a novel sandwich design, featuring two rigid layers and lattice middle made of T+ resin (Cazón-Martín et al., 2019). Impact tests showed that two of the 3D printed shin pads significantly reduced acceleration (42%–68%) and penetration (13%–32%) compared to commercial options, with consistent attenuation and contact times (Cazón-Martín et al., 2019). The most effective lattice structure was employed to create a full-scale shin pad design in Rhinoceros, physically prototyped using a multi-material 3D printer. Results have disclosed the potential of additive manufacturing for designing effective football personal protection gear, mitigating the risk of tibia fractures and addressing player and medical concerns.
4 Conclusion and future perspectives
In the past few years, shear thickening materials (both suspensions and gels) have gained particular interest among scientific community worldwide. The unique physical and chemical properties of these materials have provided considerable opportunities for their application in a broad range of scientific and engineering fields. STSs have been successfully employed in various industrial applications, especially including electronical devices and sport products, showing enhanced levels of protection, flexibility and comfort compared to the standard protective equipment. Nevertheless, the instability of these suspension including their evaporation, sensitivity to humidity, sedimentation or leakage of carrier fluids and reduced air and moisture permeability have significantly limited their use in many of these applications. Hence, in order to overcome these limitations, new smart materials based on more physically and chemically stable crosslinked structures such as polyborosiloxanes gels have been developed for impact protection applications. Such crosslinked gels, have shown great shear thickening, versatile, and multi-impact properties being able to flow and locking together to absorb a great amount of energy in case of a shock event.
Scientific investigations on the shear stiffening effect and mechanism of borosiloxane-based gels have found that a phase change intercurring between viscous-glassy-elastomeric states is present within the borosiloxane polymeric network. This phase change is ascribable to both reversible and irreversible interactions due to the transformation of the B-O bonding across the structure. STG compositions possess a great potential for the design of future shock-proof devices due to their unique features, making these light and adaptable materials even more suitable for personal protection, not only for sport applications purposes. Beyond doubt, still further research is needed in the development of STGs since there is a significant lack of information in the academic literature regarding the structural optimization of STGs. Therefore, it is of fundamental importance to investigate and identify the possible relationships between the chemical structure and formulation of raw materials and the final properties of the resulting composites. Moreover, it is scientifically relevant to develop STG networks with lower density and improved impact protection performances also using different models and simulations software. Under these circumstances, it might be possible to modify and control the properties and main features of STG-based composites starting from the synthetic process. Finally, the shear stiffening mechanism of these gels is for the most part still under investigation. Hence, further research about the influence of chemical composition on the mechanical properties of the STGs are needed. On the whole part, the unique nature of these shear thickening gels involves excellent rate-dependent performances in terms of impact and vibration damping along with high comfort levels. Therefore, the promising and scientific relevant findings open up possibilities for the design of new advanced materials widening the potential use of STGs to the field of smart anti-impact devices for sport protection and other application where safety performance and adequate comfort are particularly needed. In this context, AM technologies emerge as a competitive and viable solution for designing sport protection equipment, offering the capability to withstand even severe impacts thereby reducing the risk of sport-associated traumas. Indeed, new advanced solutions are under the corner and could be available for the next XXV winter Olympic Games of Milano-Cortina 2026 (Italy).
Author contributions
MP: Writing–original draft, Writing–review and editing. GL: Writing–review and editing. NP: Writing–review and editing. MC: Writing–review and editing, Supervision.
Funding
The author(s) declare that no financial support was received for the research, authorship, and/or publication of this article.
Conflict of interest
Author MC was employed by RE-SPORT srl.
The remaining authors declare that the research was conducted in the absence of any commercial or financial relationships that could be construed as a potential conflict of interest.
The author(s) declared that they were an editorial board member of Frontiers, at the time of submission. This had no impact on the peer review process and the final decision
Publisher’s note
All claims expressed in this article are solely those of the authors and do not necessarily represent those of their affiliated organizations, or those of the publisher, the editors and the reviewers. Any product that may be evaluated in this article, or claim that may be made by its manufacturer, is not guaranteed or endorsed by the publisher.
References
Abayazid, F. F., Carpanen, D., and Ghajari, M. (2022). New viscoelastic circular cell honeycombs for controlling shear and compressive responses in oblique impacts. Int. J. Mech. Sci. 222 (January), 107262. Available from. doi:10.1016/j.ijmecsci.2022.107262
Abayazid, F. F., and Ghajari, M. (2020). Material characterisation of additively manufactured elastomers at different strain rates and build orientations. Addit. Manuf. 33 (February), 101160. doi:10.1016/j.addma.2020.101160
Akbari, S., Sakhaei, A. H., Kowsari, K., Yang, B., Serjouei, A., Yuanfang, Z., et al. (2018). Enhanced multimaterial 4D printing with active hinges. Smart Mater Struct. 27 (6), 065027. doi:10.1088/1361-665x/aabe63
Alpina (2023). Alpina prolan - Alpina sports. Available from: https://www.alpina-sports.com/en/prolan/.
Ashokkumar, M., Thanikaivelan, P., Murali, R. C. B., and Chandrasekaran, B. (2010). Preparation and characterization of composite sheets from collagenous and chromium-collagen complex wastes using polyvinylpyrrolidone: two Problems, One Solution. Waste Biomass Valor 1 (3), 347–355. doi:10.1007/s12649-010-9030-x
ASTM F2040-02 (2002). Standard specification for helmets used for recreational snow sports. PA, USA: ASTM International West Conshohocken. Materials AS for T and. ASTM F2040-02.
Baharvandi, H. R., Alebooyeh, M., Alizadeh, M., Heydari, M. S., Kordani, N., and Khaksari, P. (2016). The influences of particle–particle interaction and viscosity of carrier fluid on characteristics of silica and calcium carbonate suspensions-coated Twaron® composite. J. Exp. Nanosci. 11 (7), 550–563. doi:10.1080/17458080.2015.1094190
Barlaz, D. (2010). Prevention of hip fractures in elderly patients using a protective garment incorporating a shear thickening fluid. United States University of Delaware.
Barnes, H. A. (1989). ShearThickening (“ dilatancy ”) in suspensions of nonaggregating solid particles dispersed in Newtonian liquids shear-thickening (" dilatancy ") in suspensions of nonaggregating solid particles dispersed in Newtonian liquids shear thickening is defined in. 2009;329.
Beiderbeck, D., Krüger, H., and Minshall, T. (2020). 21st century sport how technol will chang sport digit age, 111–132.The future of additive manufacturing in sports
Bhandalkar, S., and Deshmukh, R. (2019). Sport protective equipment market. Available from: https://www.alliedmarketresearch.com/sports-protective-equipment-market.
Bossis, G., and Brady, J. F. (1989). The rheology of Brownian suspensions. J. Chem. Phys. 91 (3), 1866–1874. doi:10.1063/1.457091
Brunner, R., Friesenbichler, B., Casartelli, N. C., Bizzini, M., Maffiuletti, N. A., and Niedermann, K. (2019). Effectiveness of multicomponent lower extremity injury prevention programmes in team-sport athletes: an umbrella review Br. J. Sports Med. 53 (5), 282–288. doi:10.1136/bjsports-2017-098944
Caine, D. J., Harmer, P. A., and Schiff, M. A. (2009). Epidemiology of injury in olympic sports. John Wiley and Sons.
Caswell, S. V., Gould, T. E., and Wiggins, J. S. (2007). “4 - protective helmets in sports,” in Materials in sports equipment. Editor A. Subic Cambridge, England (Woodhead Publishing Limited), 87–126. Available from: https://www.sciencedirect.com/science/article/pii/B9781845691318500040.
Cazón-Martín, A., Iturrizaga-Campelo, M., Matey-Muñoz, L., Rodríguez-Ferradas, M. I., Morer-Camo, P., and Ausejo-Muñoz, S. (2019). Design and manufacturing of shin pads with multi-material additive manufactured features for football players: a comparison with commercial shin pads. Proc. Inst. Mech. Eng. Part P J. Sport Eng. Technol. 233 (1), 160–169. doi:10.1177/1754337118811266
Charkarska, L., Goshev, I., Idakeva, K., Todinova, S., and Apostolov, G. (2008). Cross-linking phosphoric acid hydrolysates of collagen with cyanuric chloride. J. Soc. Leather Technol. Chem. 92, 81–84.
Che, K., Yuan, C., Qi, H. J., and Meaud, J. (2018). Viscoelastic multistable architected materials with temperature-dependent snapping sequence. Soft Matter 14 (13), 2492–2499. doi:10.1039/c8sm00217g
Chen, Y., Buggy, C., and Kelly, S. (2019). Winning at all costs: a review of risk-taking behaviour and sporting injury from an occupational safety and health perspective. Sport Med. 5 (1), 15–21. doi:10.1186/s40798-019-0189-9
Colonna, M., Parisi, M., and La Fauci, G. (2021). Device for absorbing shocks on helmets and corresponding helmet. International patent, WO2023017552A1, 19.
COLPHARMA Srl (2023). Prodotti. Available from: https://www.noene.it/prodotti/(Accessed October 21, 2023).
Cross, M. M. (1965). Rheology of non-Newtonian fluids: a new flow equation for pseudoplastic systems. J. Colloid Sci. 20 (5), 417–437. doi:10.1016/0095-8522(65)90022-x
Cross, R. (2012a). Elastic and viscous properties of silly putty. Am. J. Phys. 80 (10), 870–875. doi:10.1119/1.4732086
Cross, R. (2012b). Elastic properties of plasticine, silly putty, and tennis strings. Phys. Teach. 50 (9), 527–529. doi:10.1119/1.4767481
D3O (2023a). Discover D3O. Available from: https://www.d3o.com/discover-d3o/.
D3O (2023b). Our products. Available from: https://www.d3o.com/our-products/(Accessed October 21, 2023).
Dainese (2023). Flexagon pl Waistcoat - back - dainese. Available from: https://www.dainese.com/gb/en/ski-safety/back.
Dawson, M. A., McKinley, G. H., and Gibson, L. J. (2009). The dynamic compressive response of an open-cell foam impregnated with a non-Newtonian fluid. J. Appl. Mech. Trans. ASME 76 (6), 1–8. doi:10.1115/1.3130825
DeHass, D. M. (2009). 82-2007-2008 NCAA sports sponsorship and participation rates report. Indianapolis, IN: NCAA. Available from: http://www.ncaapublications.com/%0Ap-4124-participation-rates-1981-82-2006-07-ncaa-sportssponsorship-%0Aand-participation-rates-report.aspx.
Deshpande, A. P., Krishnan, J. M., and Kumar, P. B. S. (2010). Rheology of complex fluids. Rheology Complex Fluids, 1–257.
Dickson, T. J., and Terwiel, F. A. (2021). Injury trends in alpine skiing and a snowboarding over the decade 2008–09 to 2017–18. J. Sci. Med. Sport 24 (10), 1055–1060. doi:10.1016/j.jsams.2020.12.001
Digital Journal (2023). This report provides a comprehensive analysis of the Sports Medicine market’s size, market share, and future growth rate, as well as revenue and sales trends expected from 2023 - 2030, with a projected 9% CAGR. Available from: https://www.digitaljournal.com/pr/news/this-report-provides-a-comprehensive-analysis-of-the-sports-medicine-market-s-size-market-share-and-future-growth-rate-as-well-as-revenue-and-sales-trends-expected-from-2023-2030-with-a-projected-9-cagr- (Accessed October 21, 2023).
Dimas, L. S., Bratzel, G. H., Eylon, I., and Buehler, M. J. (2013). Tough composites inspired by mineralized natural materials: computation, 3D printing, and testing. Adv. Funct. Mater 23 (36), 4629–4638. doi:10.1002/adfm.201300215
Ding, J., Tracey, P. J., Li, W., Peng, G., Whitten, P. G., and Wallace, G. G. (2013). Review on shear thickening fluids and applications.
Dlugosch, S., Hu, H., and Chan, A. C. K. (2012). Impact protective clothing in sport: areas of application and level of utilization. Res. J. Text. Appar. 16 (3), 18–28. doi:10.1108/rjta-16-03-2012-b002
Dotti, F., Ferri, A., Moncalero, M., and Colonna, M. (2016). Thermo-physiological comfort of soft-shell back protectors under controlled environmental conditions. Appl. Ergon. 56, 144–152. doi:10.1016/j.apergo.2016.04.002
Duncan, O., Shepherd, T., Moroney, C., Foster, L., Venkatraman, P. D., Winwood, K., et al. (2018). Review of auxetic materials for sports applications: expanding options in comfort and protection. Appl. Sci. 8 (6), 941. doi:10.3390/app8060941
Dykstra, D. M. J., Busink, J., Ennis, B., and Coulais, C. (2019). Viscoelastic snapping metamaterials. J. Appl. Mech. 86 (11), 111012. doi:10.1115/1.4044036
Eather, N., Wade, L., Pankowiak, A., and Eime, R. (2023). The impact of sports participation on mental health and social outcomes in adults: a systematic review and the “Mental Health through Sport”conceptual model. Syst. Rev. 12 (1), 102–127. doi:10.1186/s13643-023-02264-8
Egres, R. G., and Wagner, N. J. (2005). The rheology and microstructure of acicular precipitated calcium carbonate colloidal suspensions through the shear thickening transition. J. Rheol. (N Y N Y) 49 (3), 719–746. doi:10.1122/1.1895800
Emery, C. A. (2023). Injury prevention research to reduce youth-sport related injuries. Open Access Gov. 39 (1), 40–41. doi:10.56367/oag-039-10903
Emery, C. A., and Pasanen, K. (2019). Current trends in sport injury prevention. Best. Pract. Res. Clin. Rheumatol. 33 (1), 3–15. doi:10.1016/j.berh.2019.02.009
Fall, A., Huang, N., Bertrand, F., Ovarlez, G., and Bonn, D. (2008). Shear thickening of cornstarch suspensions as a reentrant jamming transition. Phys. Rev. Lett. 100 (1), 018301. Available from: https://link.aps.org/doi/10.1103/PhysRevLett.100.018301.
Fan, X., Wang, S., Zhang, S., Wang, Y., and Gong, X. (2019). Magnetically sensitive nanocomposites based on the conductive shear-stiffening gel. J. Mater Sci. 54 (9), 6971–6981. Available from. doi:10.1007/s10853-019-03360-8
Fester, V., Slatter, P., and Alderman, N. (2012). Resistance coefficients for non-Newtonian flows in pipe fittings.
Flörke, O. W., Graetsch, H. A., Brunk, F., Benda, L., Paschen, S., Bergna, H. E., et al. (2000). Silica. Ullmann’s Encycl. Ind. Chem.
Fluid Inside. (2023) Fluid inside. Available from: www.fluidinside.com.
Fox Racing (2023). Fox racing. Available from: https://www.foxracing.com/.
Frias, C. F., Serra, A. C., Ramalho, A., Coelho, J. F. J., and Fonseca, A. C. (2017). Preparation of fully biobased epoxy resins from soybean oil based amine hardeners. Ind. Crops Prod. 109 (August), 434–444. Available from. doi:10.1016/j.indcrop.2017.08.041
Galindo-Rosales, F. J., Rubio-Hernández, F. J., Sevilla, A., and Ewoldt, R. H. (2011). How Dr. Malcom M. Cross may have tackled the development of “An apparent viscosity function for shear thickening fluids“. J. Nonnewt. Fluid Mech. 166 (23–24), 1421–1424.
Ganly, M., and McMahon, J. M. (2018). New generation of headgear for rugby: impact reduction of linear and rotational forces by a viscoelastic material-based rugby head guard. BMJ Open Sport Exerc Med. 4 (1), e000464–e000468. doi:10.1136/bmjsem-2018-000464
Garrett, P. R. (2017). Defoaming: theory and industrial applications. Boca Raton, Florida, United States CRC Press.
Giustini, M., Fondi, G., Bejko, D., and Bauer, R.Huib Valkenberg AP for the E-IW, Group. European Injury DataBase (EU-IDB): data analysis 2020. 2023 [Accessed 2023 October 20]. p. 41. Available from: https://www.iss.it/-/rapporto-istisan-23/12-european-injury-database-eu-idb-data-analysis-2020-marco-giustini-gianni-fondi-dritan-bejko-robert-bauer-huib-valkenberg-alessio-pitidis-for-the-eu-idb-working-group.
Goertz, M. P., Zhu, X., and Houston, J. E. (2009). Temperature dependent relaxation of a “solid–liquid.”. J. Polym. Sci. Part B Polym. Phys. 47 (13), 1285–1290. doi:10.1002/polb.21721
Going, R. E., Loehman, R. E., and Chan, M. S. (1974). Mouthguard materials: their physical and mechanical properties. J. Am. Dent. Assoc. 89 (1), 132–138. doi:10.14219/jada.archive.1974.0354
Golinelli, N., Spaggiari, A., and Dragoni, E. (2015). Mechanical behaviour of magnetic Silly Putty: viscoelastic and magnetorheological properties. J. Intell. Mater Syst. Struct. 28 (8), 953–960. Available from. doi:10.1177/1045389X15591655
Goral, V. N., Hsieh, Y.-C., Petzold, O. N., Clark, J. S., Yuen, P. K., and Faris, R. A. (2010). Perfusion-based microfluidic device for three-dimensional dynamic primary human hepatocyte cell culture in the absence of biological or synthetic matrices or coagulants. Lab. Chip 10 (24), 3380–3386. doi:10.1039/c0lc00135j
Group, B. S. I. (2000). British standard, protective clothing–protective jackets, body and shoulder protectors for horse riders–requirements and test methods. BS En. 13158, 2000.
Gürgen, S., Kuşhan, M. C., and Li, W. (2017). Shear thickening fluids in protective applications: a review. Prog. Polym. Sci. 75, 48–72. doi:10.1016/j.progpolymsci.2017.07.003
Guskiewicz, K. M., Marshall, S. W., Bailes, J., McCrea, M., Harding, H. P., Matthews, A., et al. (2007). Recurrent concussion and risk of depression in retired professional football players. Med. Sci. Sports Exerc 39 (6), 903–909. doi:10.1249/mss.0b013e3180383da5
Hady, F. M. (1995). Mixed convection boundary-layer flow of non-Newtonian fluids on a horizontal plate. Appl. Math. Comput. 68 (2–3), 105–112. doi:10.1016/0096-3003(94)00084-h
Haid, D., Foster, L., Hart, J., Greenwald, R., Allen, T., Sareh, P., et al. (2023). Mechanical metamaterials for sports helmets: structural mechanics, design optimisation, and performance. Smart Mater Struct. 32, 113001. doi:10.1088/1361-665x/acfddf
Han, Y., and Lu, W. F. (2018). Structural design of wearable electronics suitable for highly-stretched joint areas. Smart Mater Struct. 27 (10), 105042. doi:10.1088/1361-665x/aadf05
Harmon, K. G., Clugston, J. R., Dec, K., Hainline, B., Herring, S., Kane, S. F., et al. (2019). American Medical Society for Sports Medicine position statement on concussion in sport. Br. J. Sports Med. 53 (4), 213–225. doi:10.1136/bjsports-2018-100338
Hoffman, R. L. (1972). Discontinuous and dilatant viscosity behavior in concentrated suspensions. I. Observation of a flow instability. Trans. Soc. Rheol. 16 (1), 155–173. doi:10.1122/1.549250
Hoffman, R. L. (1974). Discontinuous and dilatant viscosity behavior in concentrated suspensions. II. Theory and experimental tests. J. Colloid Interface Sci. 46 (3), 491–506. doi:10.1016/0021-9797(74)90059-9
Hoffman, R. L. (1998). Explanations for the cause of shear thickening in concentrated colloidal suspensions. J. Rheol. (N Y N Y). 42 (1), 111–123. doi:10.1122/1.550884
Holt, S. E., and Perez, M. P. (2015). Impact resistant, torsion-reducing protective athletic gear using shear thickening fluid. United States Patent, US9193890B2, 8. Available from: https://patentimages.storage.googleapis.com/9c/52/bd/2ef19dcf9cfc67/US9193890.pdf.
Hössinger-Kalteis, A., Pitz, E., and Major, Z. (2023). Sensitivity study of multi-stable metamaterials applied in helmet liners. Available SSRN 4327810.
International A (2008). Standard specification for helmets used in skateboarding and trick roller skating.
Jachowicz, M., and Owczarek, G. (2020). Analysis of selected mechanical parameters for foamed materials with non-Newtonian liquid characteristics in terms of their use in aspects of protective helmets. 1–8.
Jiang, W., Gong, X., Wang, S., Chen, Q., Zhou, H., Jiang, W., et al. (2014). Strain rate-induced phase transitions in an impact-hardening polymer composite. Appl. Phys. Lett. 104 (12), 121915. doi:10.1063/1.4870044
Kaewpradit, P. (2021). Impact responses of an open-cell natural rubber foam impregnated with shear thickening fluid, 217–223.2020
Kantareddy, S. N. R., Simpson, T. W., Ounaies, Z., and Frecker, M. (2016). “3D printing of shape changing polymer structures: design and characterization of materials,”in 2016 International Solid Freeform Fabrication Symposium. University of Texas at Austin.
Khandavalli, S., and Rothstein, J. P. (2014). Extensional rheology of shear-thickening fumed silica nanoparticles dispersed in an aqueous polyethylene oxide solution. J. Rheol. (N Y N Y) 58 (2), 411–431. doi:10.1122/1.4864620
Khosroshahi, S. F., Duckworth, H., Galvanetto, U., and Ghajari, M. (2019). The effects of topology and relative density of lattice liners on traumatic brain injury mitigation. J. Biomech. 97, 109376. doi:10.1016/j.jbiomech.2019.109376
Khosroshahi, S. F., Tsampas, S. A., and Galvanetto, U. (2018). Feasibility study on the use of a hierarchical lattice architecture for helmet liners. Mater Today Commun. 14, 312–323. doi:10.1016/j.mtcomm.2018.02.002
Knapik, J. J., Hoedebecke, B. L., Rogers, G. G., Sharp, M. A., and Marshall, S. W. (2019). Effectiveness of Mouthguards for the prevention of orofacial injuries and concussions in sports: systematic review and meta-analysis. Sport Med. 49, 1217–1232. doi:10.1007/s40279-019-01121-w
La Fauci, G., Parisi, M., Nanni, A., Crosetta, L., Pugno, N. M., and Colonna, M. (2023). Design and proof-of-concept of an advanced protective system for the dissipation of tangential impact energy in helmets, based on non-Newtonian fluids. Smart Mater Struct. 32 (4), 044004. doi:10.1088/1361-665x/acc148
Lammer, H., Rosenkranz, H., Kotze, J., and Schwenger, R. (2012). System and method of using shear thickening materials in sports products. US Patent, US8105184B2.
LEATT Christopher, J., Meyer, C. S., Keevy, P. A., and Steffens, J. P. (2015). Helmet. International Patent, WO2015121787A, 11. Available from: https://worldwide.espacenet.com/patent/search?q=pn%3DWO2015121787A1.
LEATT (2020). What is 360° turbine technology? Available from: https://leatt.com/int/what-is-360-turbine-technology.
Lee, B.-W., Kim, I.-J., and Kim, C.-G. (2009). The influence of the particle size of silica on the ballistic performance of fabrics impregnated with silica colloidal suspension. J. Compos Mater 43 (23), 2679–2698. doi:10.1177/0021998309345292
Li, S., Wang, J., Zhao, S., Cai, W., Wang, Z., and Wang, S. (2017). Giant rheological effect of shear thickening suspension comprising silica nanoparticles with No aggregation. J. Mater Sci. Technol. 33 (3), 261–265. doi:10.1016/j.jmst.2016.06.008
Li, X., Zhang, D., Xiang, K., and Huang, G. (2014). Synthesis of polyborosiloxane and its reversible physical crosslinks. RSC Adv. 4 (62), 32894–32901. doi:10.1039/c4ra01877j
Liang, J., and Zhang, X.-H. (2015). Rheological properties of SP in shock transmission application. J. Mater Civ. Eng. 27 (9), 4014250. doi:10.1061/(asce)mt.1943-5533.0001227
Lin, X.-G., Guo, F., Du, C.-B., and Yu, G.-J. (2018). The mechanical properties of a novel STMR damper based on magnetorheological silly putty. Adv. Mater Sci. Eng. 2018, 1–15. doi:10.1155/2018/2681461
Lionberger, R. A., and Russel, W. B. (1997). Effectiveness of nonequilibrium closures for the many body forces in concentrated colloidal dispersions. J. Chem. Phys. 106 (1), 402–416. doi:10.1063/1.473029
Liravi, F., Darleux, R., and Toyserkani, E. (2017). Additive manufacturing of 3D structures with non-Newtonian highly viscous fluids: finite element modeling and experimental validation. Addit. Manuf. 13, 113–123. Available from. doi:10.1016/j.addma.2016.10.008
Liu, B., Du, C., Deng, H., Fu, Y., Guo, F., Song, L., et al. (2022b). Study on the shear thickening mechanism of multifunctional shear thickening gel and its energy dissipation under impact load. Polym. Guildf. 247 (January), 124800. Available from. doi:10.1016/j.polymer.2022.124800
Liu, M., Jiang, W., Chen, Q., Wang, S., Mao, Y., Gong, X., et al. (2016). A facile one-step method to synthesize SiO 2@ polydopamine core–shell nanospheres for shear thickening fluid. RSC Adv. 6 (35), 29279–29287. doi:10.1039/c5ra25759j
Liu, Y., Zhou, C., Lv, J., Bai, Y., Xu, K., Zhang, H., et al. (2022a). Dual network poly (dimethyl siloxane)–impact hardening polymer composite with autonomous self-healing and soft–stiffness switch abilities. Mater Today Commun. 33, 104489. doi:10.1016/j.mtcomm.2022.104489
Liu, Z., Picken, S. J., and Besseling, N. A. M. (2014). Polyborosiloxanes (PBSs), synthetic kinetics, and characterization.
Manske, R., Magee, D., Zachazewski, J., and Quillen, W. (2011). Athletic and sport issues in musculoskeletal rehabilitation.
Mao, Y., Ding, Z., Yuan, C., Ai, S., Isakov, M., Wu, J., et al. (2016). 3D printed reversible shape changing components with stimuli responsive materials. Sci. Rep. 6 (1), 24761. doi:10.1038/srep24761
Martin, R., Rekondo, A., de Luzuriaga, A. R., Santamaria, A., and Odriozola, I. (2015). Mixing the immiscible: blends of dynamic polymer networks. RSC Adv. 5 (23), 17514–17518. doi:10.1039/c5ra00628g
Mashchenko, V. I., Sitnikov, N. N., Khabibullina, I. A., Kuzmin, M. K., and Chausov, D. N. (2020). Structure and properties of self-healing materials based on hydroxyl terminated polydimethylsiloxane and boric acid. J. Phys. Conf. Ser. 1560 (1), 012037. doi:10.1088/1742-6596/1560/1/012037
Metts, D. M., and Gower, R. M. (2021). Non-Newtonian materials for the prevention of mild traumatic brain injury. USA: espacenet; an improved design for an athletic helmet incorporating non-Newtonian fluid shock absorbing structures, 18. Available from: https://worldwide.espacenet.com/patent/search?q=pn%3DUS11045710B2.
Mora, S., Pugno, N. M., and Misseroni, D. (2022). 3D printed architected lattice structures by material jetting. Mater Today 59, 107–132. doi:10.1016/j.mattod.2022.05.008
Moreland, G., and Barkley, L. C. (2021). Concussion in sport. Curr. Sports Med. Rep. 20 (4), 181–182. doi:10.1249/jsr.0000000000000825
Morgan, J. T., and Morgan, G. E. (2022). Helmet with non-Newtonian fluid liner system. US patent, US11219263B2, 18. Available from: https://worldwide.espacenet.com/patent/search?q=pn%3DUS11219263B2.
Moriana, A. D., Tian, T., Sencadas, V., and Li, W. (2016). Comparison of rheological behaviors with fumed silica-based shear thickening fluids. Korea-Australia Rheol. J. 28, 197–205. doi:10.1007/s13367-016-0020-9
National Institute of Health (2021). Sports injuries basics. Available from: https://www.niams.nih.gov/health-topics/sports-injuries/basics/symptoms-causes (Accessed October 20, 2023).
National Operating Committee on Standards for Athletic Equipment (NOCSAE) (2012). Standard performance specification for newly manufactured football helmets. Paper No. ND001-11m12. NOCSAE Stand (November), 1–6.
National Safety Council (2023). Sports and recreational injuries. Available from: https://injuryfacts.nsc.org/home-and-community/safety-topics/sports-and-recreational-injuries/(Accessed October 20, 2023).
Nickel, A., and Edgecombe, B. D. (2012). in 4.30 - industrial applications of ROMP. Editors K. Matyjaszewski, and M. B. T.-PSACR Möller (Amsterdam: Elsevier), 749–759. Available from: https://www.sciencedirect.com/science/article/pii/B9780444533494001060.
Nicotra, M., Moncalero, M., Messori, M., Fabbri, E., Fiorini, M., and Colonna, M. (2014). Thermo-mechanical and impact properties of polymeric foams used for snow sports protective equipment. Procedia Eng. 72, 678–683. doi:10.1016/j.proeng.2014.06.115
NOCSAE (2015). Standard performance specification for newly manufactured lacrosse helmets with faceguard - nd041-11m15. (June).
NORSOREX® (2023). Our products and product development service. Available from: https://www.norsorex.com/Our-products-and-services/(Accessed October 21, 2023).
OECD (2021). International regulatory Co-operation and international organisations. The Case of ASTM International. Available from: https://www.oecd.org/gov/regulatory-policy/irc-astm-case-study.pdf.
OpenPR (2023). Sports medicine market is anticipated to create a value opportunity of around USD 10.8 billion by 2031 | stryker corporation, arthrex, wright medical technology. Available from: https://www.openpr.com/news/3208998/sports-medicine-market-is-anticipated-to-create-a-value (Accessed October 21, 2023).
Ozdemir, Z., Tyas, A., Goodall, R., and Askes, H. (2017). Energy absorption in lattice structures in dynamics: nonlinear FE simulations. Int. J. Impact Eng. 102, 1–15. doi:10.1016/j.ijimpeng.2016.11.016
Pan, Z., Huang, B., Zhu, L., and Zeng, K. (2020). Synthesis of a boron-containing adhesion promoter for improving the adhesion of addition-cure silicone rubber to PPA. J. Adhes. Sci. Technol. 34 (7), 792–805. Available from. doi:10.1080/01694243.2019.1685758
Pannikottu, A., and Abraham, A. (2017). Football helmet liner to reduce concussions and traumatic brain injuries. US Patent, US 20150033453A1.
Parisi, M., Allen, T., Colonna, M., Pugno, N., and Duncan, O. (2023). Indentation and impact response of conventional, auxetic, and shear thickening gel infused auxetic closed cell foam. Smart Mater Struct. 32 (7), 074004. doi:10.1088/1361-665x/acd91c
Part, C. F. R., Standard, S., This, B. H., Part, C. F. R., and Part, C. F. R. (2023). Title 16 —commercial practices chapter II —consumer product safety commission subchapter B —consumer product safety act regulations 16, 1203.
Peterson, C., Xu, L., and Florence, C. (2021). Average medical cost of fatal and non-fatal injuries by type in the USA. Inj. Prev. 27 (1), 1.1–10. doi:10.1136/injuryprev-2019-043544
Pfriem, S. D. (2016). Standards-based regulation of athletic protective headgear - policy background, mechanisms and evaluation. J. Law Heal 29 (1), 55–84. Available from: https://www.proquest.com/scholarly-journals/standards-based-regulation-athletic-protective/docview/1826920650/se-2?accountid=46437.
Phung, T. N., Brady, J. F., and Bossis, G. (1996). Stokesian dynamics simulation of Brownian suspensions. J. Fluid Mech. 313, 181–207. doi:10.1017/s0022112096002170
Physiopedia. Protective Sports Equipments (2022). Available from: https://www.physio-pedia.com/Protective_Sports_Equipments.
Plant, D. J. (2009). Flexible energy absorbing material and methods of manufacture thereof. US Patent, US7608314B2.
POC Sports (2023). Spin system. Available from: https://www.pocsports.com/blogs/press-release/poc-presents-spin-and-clarity-brand-new-innovations.
Polmann, H., Melo, G., Conti Réus, J., Domingos, F. L., de Souza, B. D. M., Padilha, A. C., et al. (2020). Prevalence of dentofacial injuries among combat sports practitioners: a systematic review and meta-analysis. Dent. Traumatol. 36 (2), 124–140. doi:10.1111/edt.12508
Polyanswer (2023a). Polyanswer - the future of high-impact technology. Available from: http://polyanswer.com/#.
Polyanswer (2023b). Products DILATANT FLUID and COMPOUNDED SYSTEMS. Available from: http://polyanswer.com/#s-products (Accessed October 21, 2023).
Qiu, Z. (2020). The influence of the design and manufacture of sports equipment on sports. J. Phys. Conf. Ser. 1549 (3), 032039. doi:10.1088/1742-6596/1549/3/032039
Rajendra Boopathy, V., Sriraman, A., and Arumaikkannu, G. (2019). Energy absorbing capability of additive manufactured multi-material honeycomb structure. Rapid Prototyp. J. 25 (3), 623–629. doi:10.1108/rpj-03-2018-0066
Rheon (2023). RHEONTM technology. Available from: https://rheonlabs.com/rheon-technology-how-it-works/.
RHEON Labs (2023). Rheon technology products. Available from: https://rheonlabs.com/rheon-technology-products/(Accessed October 21, 2023).
Rogers Corporation (2023). Elastomeric material solutions. Available from: https://www.rogerscorp.com/elastomeric-material-solutions (Accessed October 21, 2023).
Rudolf, M., Boutelier, D., Rosenau, M., Schreurs, G., and Oncken, O. (2016). Rheological benchmark of silicone oils used for analog modeling of short- and long-term lithospheric deformation. Tectonophysics 684, 12–22. doi:10.1016/j.tecto.2015.11.028
Sang, M., Wu, Y., Liu, S., Bai, L., Wang, S., Jiang, W., et al. (2021). Flexible and lightweight melamine sponge/MXene/polyborosiloxane (MSMP) hybrid structure for high-performance electromagnetic interference shielding and anti-impact safe-guarding. Compos Part B Eng., 211, 108669. Available from: https://www.sciencedirect.com/science/article/pii/S1359836821000639.
Schwank, J., Thomas, V., and Akervall, J. (2017). Adaptive mouth guard and method of use. International Patent, WO2015175192A1, 30. Available from: https://worldwide.espacenet.com/patent/search?q=pn%3DEP3142756A1.
Sema, YILDIZ. SYNTHESIS AND RHEOLOGICAL BEHAVIOR OF SHEAR THICKENING FLUIDS (STFs) FOR LIQUID ARMOR APPLICATIONS. 2013;(December):1–102.
Serra, G. F., Fernandes, F. A. O., de Sousa Rja, , Noronha, E., and Ptak, M. (2022). New hybrid cork-STF (Shear thickening fluid) polymeric composites to enhance head safety in micro-mobility accidents. Compos Struct. 301, 116138. doi:10.1016/j.compstruct.2022.116138
Seto, R., Mari, R., Morris, J. F., and Denn, M. M. (2013). Discontinuous shear thickening of frictional hard-sphere suspensions. Phys. Rev. Lett. 111 (21), 218301. doi:10.1103/physrevlett.111.218301
Shargawi, A. A., Amick, R. Z., Jorgensen, M. J., and Asmatulu, R. (2022). Experimental investigation of energy absorbency and dampening characteristics of D3O ® material during low velocity static impacts.
Shargawi, A. A., Jorgensen, M. J., Hakansson, N. A., Amick, R. Z., and Asmatulu, R. (2023). Energy absorbency and impact resistance of D3O® materials under dynamic impact loadings. Am. J. Aerosp. Eng. 10 (1), 1–10.
Shenoy, S. S., and Wagner, N. J. (2005). Influence of medium viscosity and adsorbed polymer on the reversible shear thickening transition in concentrated colloidal dispersions. Rheol. acta 44, 360–371. doi:10.1007/s00397-004-0418-z
Siegkas, P., Sharp, D. J., and Ghajari, M. (2019). The traumatic brain injury mitigation effects of a new viscoelastic add-on liner. Sci. Rep. 9 (1), 3471. doi:10.1038/s41598-019-39953-1
Signetti, S., Nicotra, M., Colonna, M., and Pugno, N. M. (2019). Modeling and simulation of the impact behavior of soft polymeric-foam-based back protectors for winter sports. J. Sci. Med. Sport 22, S65–S70. doi:10.1016/j.jsams.2018.10.007
Sochi, T. (2010). Non-Newtonian flow in porous media. Polym. Guildf. 51 (22), 5007–5023. Available from. doi:10.1016/j.polymer.2010.07.047
Sorbothane (2023b). Sorbothane overview. Available from: https://www.sorbothane.com/material-properties.aspx.
Soutrenon, M., and Michaud, V. (2014). Impact properties of shear thickening fluid impregnated foams. Smart Mater Struct. 23 (3), 035022. doi:10.1088/0964-1726/23/3/035022
Spinelli, D. J., Plaisted, T. A., and Wetzel, E. D. (2018). Adaptive head impact protection via a rate-activated helmet suspension. Mater Des. 154, 153–169. doi:10.1016/j.matdes.2018.04.083
Stitt, D., Kabaliuk, N., Alexander, K., and Draper, N. (2022). Potential of soft-shell rugby headgear to mitigate linear and rotational peak accelerations. Ann. Biomed. Eng. 50 (11), 1546–1564. doi:10.1007/s10439-022-02912-5
Tang, M., Huang, G., Zhang, H., Liu, Y., Chang, H., Song, H., et al. (2017). Dependences of rheological and compression mechanical properties on cellular structures for impact-protective materials.
Tang, M., Wang, W., Xu, D., and Wang, Z. (2016). Synthesis of structure-controlled polyborosiloxanes and investigation on their viscoelastic response to molecular mass of polydimethylsiloxane triggered by both chemical and physical interactions. Ind. Eng. Chem. Res. 55 (49), 12582–12589. doi:10.1021/acs.iecr.6b03823
The Evolution of Sports Equipment (2016). Available from: https://urbannaturale.com/the-evolution-of-sports-equipment/.
Tran, D., Cooke, M. S., and Newsome, P. R. H. (2001). Laboratory evaluation of mouthguard material. Dent. Traumatol. 17 (6), 260–265. doi:10.1034/j.1600-9657.2001.170604.x
Venkatraman, P., and Tyler, D. J. (2016). A critical review of impact resistant materials used in sportswear clothing.
Vickers, N. J. (2017). Animal communication: when i’m calling you, will you answer too? Curr. Biol. 27 (14), R713–R715. doi:10.1016/j.cub.2017.05.064
Wagner, N. J., and Russel, W. B. (1989). Nonequilibrium statistical mechanics of concentrated colloidal dispersions: hard spheres in weak flows with many-body thermodynamic interactions. Phys. A Stat. Mech. its Appl. 155 (3), 475–518. doi:10.1016/0378-4371(89)90003-4
Wang, S., Gong, L., Shang, Z., Ding, L., Yin, G., Jiang, W., et al. (2018b). Novel safeguarding tactile e-skins for monitoring human motion based on SST/PDMS-AgNW-PET hybrid structures. Adv. Funct. Mater 28, 1707538. doi:10.1002/adfm.201707538
Wang, S., Jiang, W., Jiang, W., Ye, F., Mao, Y., Xuan, S., et al. (2014). Multifunctional polymer composite with excellent shear stiffening performance and magnetorheological effect. J. Mater Chem. C 2, 7133–7140. doi:10.1039/c4tc00903g
Wang, S., Xuan, S., Jiang, W., Jiang, W., Yan, L., Mao, Y., et al. (2015). Rate-dependent and self-healing conductive shear stiffening nanocomposite: a novel safe-guarding material with force sensitivity. J. Mater Chem. A 3 (39), 19790–19799. –9. Available from. doi:10.1039/C5TA06169E
Wang, S., Xuan, S., Liu, M., Bai, L., Zhang, S., Sang, M., et al. (2017). Smart wearable Kevlar-based safeguarding electronic textile with excellent sensing performance. Soft Matter 13 (13), 2483–2491. doi:10.1039/c7sm00095b
Wang, S., Xuan, S., Wang, Y., Xu, C., Mao, Y., Liu, M., et al. (2016b). Stretchable polyurethane sponge scaffold strengthened shear stiffening polymer and its enhanced safeguarding performance. ACS Appl. Mater Interfaces 8 (7), 4946–4954. doi:10.1021/acsami.5b12083
Wang, Y., Ding, L., Zhao, C., Wang, S., Xuan, S., Jiang, H., et al. (2018a). A novel magnetorheological shear-stiffening elastomer with self-healing ability. Compos Sci. Technol. 168, 303–311. doi:10.1016/j.compscitech.2018.10.019
Wang, Y., Wang, S., Xu, C., Xuan, S., Jiang, W., and Gong, X. (2016a). Dynamic behavior of magnetically responsive shear-stiffening gel under high strain rate. Compos Sci. Technol. 127, 169–176. doi:10.1016/j.compscitech.2016.03.009
Wang, Z. G., Sun, Y. Y., Yu, Z. W., and Li, Q. Y. (2013). Characterization and application of shear thickening fluids. Appl. Mech. Mater 405–408, 2503–2506. doi:10.4028/www.scientific.net/amm.405-408.2503
Wei, M., Lin, K., and Sun, L. (2022). Shear thickening fluids and their applications. Mater Des. 216, 110570. Available from: https://www.sciencedirect.com/science/article/pii/S0264127522001915.
Wetzel, E. D., Lee, Y. S., Egres, R. G., Kirkwood, K. M., Kirkwood, J. E., and Wagner, N. J. (2004). “The effect of rheological parameters on the ballistic properties of shear thickening fluid (STF)-kevlar composites,” in AIP conference proceedings (American Institute of Physics), 288–293.
Witek, D. M., Dombrowski, R., and Wagner, N. J. (2020). Movement-reactive athletic apparel and methods of making the same. US Patent, US10716338B2, 27. Available from: https://worldwide.espacenet.com/patent/search?q=pn%3DUS10716338B2.
Wu, J., Yuan, C., Ding, Z., Isakov, M., Mao, Y., Wang, T., et al. (2016). Multi-shape active composites by 3D printing of digital shape memory polymers. Sci. Rep. 6 (1), 24224. doi:10.1038/srep24224
Wu, Q., Xiong, H., Peng, Y., Yang, Y., Kang, J., Huang, G., et al. (2019). Highly stretchable and self-healing “solid–liquid” elastomer with strain-rate sensing capability. ACS Appl. Mater Interfaces 11 (21), 19534–19540. –40. Available from. doi:10.1021/acsami.9b05230
Wyart, M., and Cates, M. E. (2014). Discontinuous shear thickening without inertia in dense non-Brownian suspensions. Phys. Rev. Lett. 112 (9), 098302. doi:10.1103/physrevlett.112.098302
Yang, H.-G., Li, C.-Z., Gu, H.-C., and Fang, T.-N. (2001). Rheological behavior of titanium dioxide suspensions. J. Colloid Interface Sci. 236 (1), 96–103. doi:10.1006/jcis.2000.7373
Yudong, M. (2021). Football goalkeeper glove based on non-Newtonian fluid. Chinese Patent, CN213407689U, 9. Available from: https://worldwide.espacenet.com/patent/search?q=pn%3DCN213407689U.
Zarei, M., and Aalaie, J. (2020). Application of shear thickening fluids in material development. J. Mater Res. Technol. 9 (5), 10411–10433. Available from. doi:10.1016/j.jmrt.2020.07.049
Zhang, S., Wang, S., Hu, T., Xuan, S., Jiang, H., and Gong, X. (2020). Study the safeguarding performance of shear thickening gel by the mechanoluminescence method. Compos Part B Eng. 180, 107564, 107564. Available from. doi:10.1016/j.compositesb.2019.107564
Zhang, S., Wang, S., Wang, Y., Fan, X., Ding, L., Xuan, S., et al. (2018). Conductive shear thickening gel/polyurethane sponge: a flexible human motion detection sensor with excellent safeguarding performance. Compos Part A Appl. Sci. Manuf. 112, 197–206. doi:10.1016/j.compositesa.2018.06.007
Zhao, C., Gong, X., Wang, S., Jiang, W., and Xuan, S. (2020). Shear stiffening gels for intelligent anti-impact applications. Cell Rep. Phys. Sci. 1 (12), 100266. Available from. doi:10.1016/j.xcrp.2020.100266
Zhou, Z., Solomon, M. J., Scales, P. J., and Boger, D. V. (1999). The yield stress of concentrated flocculated suspensions of size distributed particles. J. Rheol. (N Y N Y). 43 (3), 651–671. doi:10.1122/1.551029
Keywords: Non-Newtonian fluids, sport protection, shear thickening, polyborosiloxane, energy absorption
Citation: Parisi M, La Fauci G, Pugno NM and Colonna M (2023) Use of shear thickening fluids in sport protection applications: a review. Front. Mater. 10:1285995. doi: 10.3389/fmats.2023.1285995
Received: 30 August 2023; Accepted: 30 October 2023;
Published: 17 November 2023.
Edited by:
Yang Yu, University of New South Wales, AustraliaReviewed by:
Mazdak Ghajari, Imperial College London, United KingdomShaoqi Li, Nanjing Tech University, China
Copyright © 2023 Parisi, La Fauci, Pugno and Colonna. This is an open-access article distributed under the terms of the Creative Commons Attribution License (CC BY). The use, distribution or reproduction in other forums is permitted, provided the original author(s) and the copyright owner(s) are credited and that the original publication in this journal is cited, in accordance with accepted academic practice. No use, distribution or reproduction is permitted which does not comply with these terms.
*Correspondence: Mariafederica Parisi, mariafederica.paris2@unibo.it