Wideband Absorption at Low Microwave Frequencies Assisted by Magnetic Squeezing in Metamaterials
- 1Department of Basic Science, Air Force Engineering University, Xi’an, China
- 2College of Information Counter, Air Force Early Warning Academy, Wuhan, China
- 3School of Electronics and Information Engineering, Xi’an Jiaotong University, Xi’an, China
In this paper, the magnetic squeezing effect in metamaterials is explored and applied to achieve wideband absorption in low-frequency microwave bands. To this end, a metamaterial absorber (MA) is proposed, which consists of a square lattice of a split ring resonator (SRR) placed on top of a magnetic absorbing material (MAM) layer backed by a conducting ground. In the positive resonance region of SRRs, a strong magnetic squeezing effect occurs and more concentrated magnetic field lines are confined within SRRs. This results in significant magnetic field enhancement within the MAM layer, which provides a prerequisite for high-efficiency absorption enhancement at low frequencies. To verify this method, we fabricated a prototype using a 3.0 mm thick silicone MAM sheet. Both the simulation and experiment results show that with the assistance of magnetic squeezing in the SRR array, the absorption at lower frequencies is significantly enhanced and is above 90% in 1.25–2.31 GHz under normal incidence. Furthermore, the MA exhibits satisfactory stability for different polarization states and incident angles due to the square lattice of the SRR array. This design method may find potential applications in fields such as electromagnetic compatibility, wireless communication, and others.
Introduction
Metamaterials provide a series of novel design concepts achieving peculiar physical phenomena and effects that cannot be realized with natural materials, such as negative refractive index [1], electromagnetic (EM) wave cloaking [2, 3], and an inverse Doppler effect [4]. As a kind of special methodology, metamaterials have been widely used not only in the military field, for example, EM stealth [5–7], but also in the civil field, such as solar energy harvesting [8] and biological sensing [9]. Since Landy et al. proposed a late-model perfect metamaterial absorber (MA) which can obtain an absorptivity rate of 99% at a specific frequency [10], MAs with the framework of metal-substrate-metal have been rapidly developed [11–14], which were generally based on electric or magnetic resonances and had the property of flexible adjustable absorption frequency by changing the geometrical parameters of the unit cell. Besides, traditional radar absorbing materials (RAMs) are generally comprised of magnetic metals or ferrite nanocrystalline particles dispersed in a polymer matrix, and always have a high thickness and heavy weight. In contrast, MAs have the advantage of high absorptivity, thin thickness, and a flexible design. However, the bandwidth of this kind of MAs is too narrow. Therefore, one common purpose in this topic is to broaden the bandwidth of the MAs and improve the absorption level simultaneously [15–18]. Thus, a great many research attempts have been implemented, including stacking multiple resonances together in the vertical direction [19], loading with lumped resistors [20], and applying spoof surface plasmon polariton (SSPP) [21, 22].
Nevertheless, the MAs still face two challenges before they can be applied in engineering. One is to resolve the contradiction that enables high-efficiency absorption in low frequency bands with thin thickness and light weight. The other is that the MAs in most literature aims to absorb EM waves with a normal or small incidence angle. But in practice, the incident angle is usually oblique or glancing. In this case, the absorption efficiency of the MAs decreases with the increase of the incident angle, leading to a poor absorption performance. In order to solve these two issues, a series of studies have been conducted. As in Ref. 23, a kind of two-dimensional MA was proposed, which can achieve an absorption peak in the P-band with an incident angle ranging from 0 to 45°. In Ref. [22], two hybrid plasmonic MAs, combining a plasmonic structure covering and various traditional absorbing materials were proposed, achieving high-efficiency absorption in the frequency bands of 4.1–35 and 4.7–31.2 GHz, respectively. In Ref. 24, a two-layer MA consisting of a non-planar metamaterial and a magnetic microwave absorbing material was proposed, and it can realize 90% absorptivity over the whole 2.0–18.0 GHz range. Nevertheless, this research just focused on one single subject. That is, MAs with not only wide-angle absorption, but also obtaining low-frequency absorption with limited thickness are important to be studied and suggest promising prospects in various applications.
In this paper, firstly, the magnetic squeezing effect in metamaterials is explored, which indicates that by virtue of the positive resonance region of the metamaterials, the magnetic field lines will be squeezed, resulting in significant magnetic field strength enhancement. Then, a kind of metamaterial absorber (MA) based on the effect is proposed, featuring wideband absorption in the low-frequency microwave bands with thin thickness and light weight. The MA consists of a square lattice of a split ring resonator (SRR), placed directly on the top of a grounded magnetic absorbing material (MAM) layer. In the positive resonance region of SRRs, a strong magnetic squeezing effect occurs. That is, more concentrated magnetic field lines are confined within the SRR arrays. This results in significant magnetic field enhancement within the MAM layer, which provides a prerequisite for high-efficiency absorption enhancement at low frequencies. To verify this, we fabricated a prototype using a 3.0 mm thick silicone MAM sheet. Both the simulation and experiment results show that under normal incidence, the 10 dB absorption band is 1.25–2.31 GHz. Furthermore, the lattice structure enables the MA to exhibit satisfactory stability for polarization states and incident angles. This design method may find potential applications in fields such as electromagnetic compatibility, wireless communication, and others.
Model Analysis
The schematics and working principles of the proposed MA are shown in Figure 1. The MA consists of three layers: the metamaterial, MAM, and conducting ground layer. When the EM waves impinge on the structure, magnetic resonance occurs. In the positive resonance region, the phenomenon of magnetic squeezing will take place. The magnetic field lines will be confined within the SRR arrays and the magnetic field strength will be enhanced inevitably, as shown on the right side of Figure 1. In addition, by changing the geometrical parameters, the magnetic resonant frequency can be adjusted correspondingly and the positive resonance region can be changed into a low-frequency band. Therefore, derived from the high loss of MAM, low-frequency microwave absorption can be achieved. Also, the lattice structure enables the structure to have satisfactory stability for polarization and wide incident angles.
Low-Frequency Wideband Absorption
Unit Structure Design
As schematically depicted in Figure 2, the unit of the proposed MA is shown. The top layer is the SRR arrays etched on FR4 (εr = 4.3, the loss tangent is 0.025) with the period of P = 8.0 mm, and the gap between adjacent SRRs is s = 1.40 mm. The middle layer is the MAM with the thickness of d = 3.00 mm, and the bottom layer is the conducting ground. The other geometrical parameters are: l1 = 6.60 mm, l2 = 6.95 mm, h = 7.75 mm, r = 0.4 mm, g = 0.3 mm, and t = 0.8 mm.
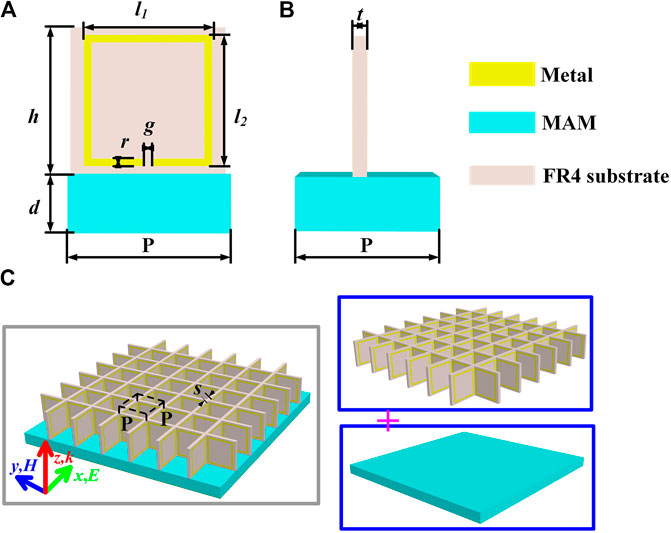
FIGURE 2. Schematic illustration of the unit of proposed MA: (A) front view, and (B) side view. (C) Perspective view of the MA.
Firstly, we simulated the reflectivity spectra of the MAM with different incident angles using CST Microwave Studio 2018. The boundary conditions along the x- and y-directions are the unit cell boundaries, while along the z-direction is open add space. As we all know, the reflectivity R(ω) and transmittance T(ω) can be obtained from the reflection and transmission coefficients S11(ω) and S21(ω). That is, R(ω) = |S11(ω)|2 and T(ω) = |S21(ω)|2. The absorption A(ω) can be calculated by A(ω) = 1 − T(ω) − R(ω) = 1 − |S11(ω)|2 − |S21(ω)|2. Due to the specular reflection of the conducting ground, S21(ω) would be nearly zero in the frequency range. So the absorption can be simplified to A(ω) = 1 − |S11(ω)|2. In other words, we can use reflectivity spectra to represent the absorption performance. Figure 3A shows the simulated reflectivity spectra of the MAM at different angles. It is obvious that there is only a reduction of 9 dB within a very narrow bandwidth and only for the specific incident angles. Self-evidently, only the MAM cannot meet the requirements of practical application.
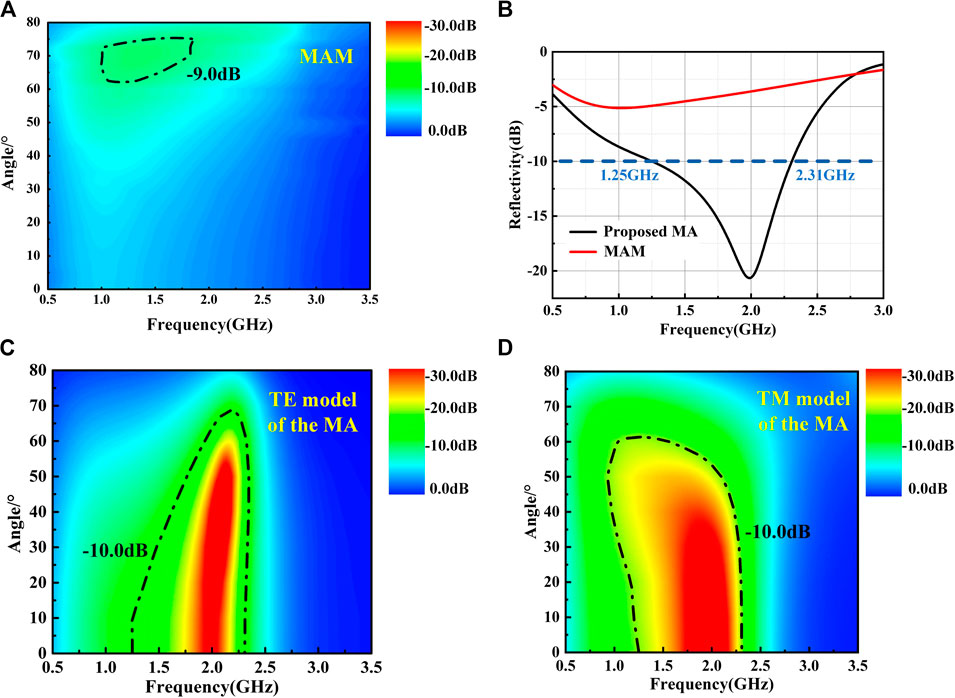
FIGURE 3. (A) The simulated reflectivity spectra of the MAM at different angles. (B) The reflectivity of the comparison between the proposed MA and MAM under normal incidence. The simulated reflectivity spectra of the proposed MA at different angles under (C) TE polarized waves and (D) TM polarized waves.
In consideration of the high magnetic loss of the MAM, the absorption performance will be improved significantly if the magnetic field strength in the MAM can be enhanced greatly. In the previous work [25, 26], the SRRs or their derivatives were widely used to construct left-handed metamaterials by virtue of the negative effective permeability in the negative resonance region. Whereas, in the positive resonance region, the effective permeability will be larger than 1. In other words, the magnetic field strength within or even below the SRR will be enhanced greatly. Placing the MAM beneath the SRR, we can achieve high-efficiency absorption performance in low-frequency microwave bands. Just as Figure 3B shows, under normal incidence, the absorption rate of the proposed MA is enhanced significantly and the –10 dB frequency band is 1.25–2.31 GHz, compared with the MAM.
In addition, the reflectivity spectra of the proposed MA varied with different incident angles for TE and TM polarized waves are shown in Figures 3C,D. Clearly, under TE polarized waves, a 10 dB absorption band can be achieved in 1.25–2.31 GHz with an incident angle ranging from 0 to 70°, and as for the TM polarized waves, the 10 dB absorption band gradually increases as the incident angle increases. Compared with Figure 3A, distinctly, the performances of the absorption bandwidth, absorption level, and angular domain have been improved significantly.
The design steps of our proposed MA can be summarized as follows: firstly, we analyzed and simulated the absorptive performance of the MAM, indicating that there is just a reduction of 9 dB within a very narrow bandwidth, only for the specific incident angles, and only the MAM cannot meet the requirements of practical application. Secondly, in consideration of the high magnetic loss of the MAM, the absorption performance can be improved significantly if the magnetic field strength in the MAM can be enhanced. By utilizing the positive resonance region of the SRRs and the magnetic squeezing effect, the magnetic field strength in the MAM will be greatly enhanced. Finally, the metamaterial absorber (MA) is proposed, which consists of SRR arrays placed on top of the MAM backed by a conducting ground.
Theoretical Analysis
When a kind of loss material is applied as the substrate, both the dielectric loss and the magnetic loss lead to high-efficiency absorption, following the relation: Ptotal = PE + PM=(1/2)ωε″|E|2+(1/2)ωµ″|H|2 [27]. Here, E is the total electric field, ω and ε″ are the angular frequency and imaginary part of the permittivity, respectively. H is the total magnetic field, and µ″ is the imaginary part of the magnetic permeability. As previously analyzed, when the substrate is a MAM with high magnetic loss, by increasing the magnetic field strength in the MAM, the absorption efficiency will be significantly improved. Because the magnetic field is rotational and the magnetic field lines have to be closed to form a circulation, in the positive resonance region, the magnetic field lines tend to be squeezed within the MAM. On the other side, in the positive resonance region, the direction of magnetic field induced by SRR will be in accord with the incident magnetic field. That is to say, the magnetic field strength below the SRR structure will be enhanced greatly by superimposing them together. In Figure 4A, the retrieved effective permeability of the proposed MA is shown. Clearly, in the frequency band from 1.26 to 2.35 GHz, the real component of effective permeability is larger than one and the structure operates in the positive resonance region, which corresponds to the 10 dB absorptive frequency band shown in Figure 3B. Clearly, the frequency band of the positive resonance region is a little different from the absorbing frequency band, after comprehensive analysis, the reason can be concluded that the boundary conditions of the simulation and the retrieving effective permeability MATLAB procedure [28] are not the same, in other words, the conducting ground in the retrieving effective permeability MATLAB procedure is taken out. However, the purpose of retrieving effective permeability of the proposed MA is to verify that in the positive resonance region, the magnetic squeezing effect occurs, and further validating our scheme, in other words, the retrieved effective permeability is for reference only, therefore, the subtle differences between the frequency bands are acceptable.
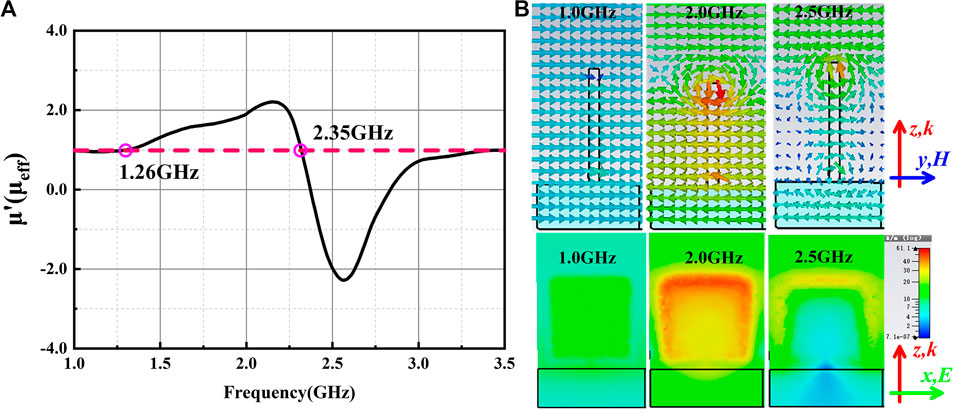
FIGURE 4. (A) The retrieved effective permeability of the proposed MA. (B) Magnetic field distribution of 1.0, 2.0, and 2.5 GHz in the y-z and x-z planes for the normal incidence.
In order to verify the analysis mentioned above, we monitored the magnetic field distribution at 1.0 GHz (the real component of effective permeability is approximately equal to 1), 2.0 GHz (the real component of effective permeability is larger than 1), and 2.5 GHz (the real component of effective permeability is negative) in the y-z and x-z planes with normal incident EM waves, just as Figure 4B shows. Clearly, at 2.0 GHz, which is in the positive resonance region, the magnetic field lines above the MA structure are confined within or below the MA, leading to significant magnetic field enhancement, which provides a prerequisite for low-frequency absorption. While at 2.5 GHz, which belongs to the negative resonance region, in contrast to the direction of the incident magnetic field, the direction of magnetic field induced by SRR will be in the opposite direction. That is, the magnetic field strength in the MAM will be weakened inevitably, as shown in Figure 4B, which will inevitably contribute to weaker absorption.
In addition, simulated results of our proposed MA in contrast to the recent works of broadband MAs are also presented in Table 1.
The comprehensive comparison concludes that our proposed MA achieves an optimal performance of low-frequency microwave absorption with comprehensive consideration of the absorption band and electrical thickness.
Experiment Verification
To verify our design concept, we fabricated and measured a prototype with dimensions of 600 × 600 mm2. The prototype photograph is shown in Figure 5A. The components from top to bottom are: SRR arrays etched on FR4 dielectric, the MAM with 3.0 mm thickness and conducting ground. Firstly, the factory-made samples of SRR arrays are assembled. Then, the samples are glued onto the MAM and a plate of conducting ground. Under normal incidence, the comparison between the simulated and measured reflectivity curves (TE and TM polarized) are shown in Figure 5B. Furthermore, in order to obtain the reflection coefficient of oblique incidence, what we need to do is to adjust the angle disc to get the specific angle like the detail in Figure 5A, while the horn feed stays stationary. Hence, correspondingly, the angle of the incident wave is controlled. The reflectivity spectrum varied with different incident angles for the TE and TM polarized waves are shown in Figures 5C,D, respectively. Compared with Figures 3C,D, it is obvious that the agreements are very good considering the tolerance in the fabrication and assembly, which validates our scheme. Hence, to some extent, we can agree that our proposed MA can achieve wide-angle microwave absorption in a low-frequency band.
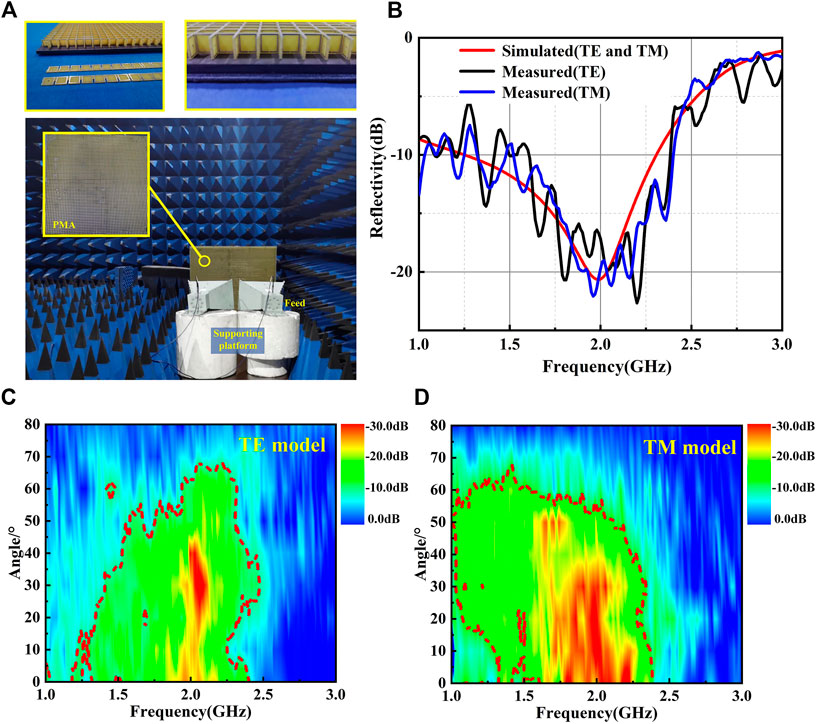
FIGURE 5. (A) The fabricated prototype of the MA and the measured environment of oblique incidence. (B) Comparison between simulated and measured reflectivity spectra under normal incidence. Measured reflectivity spectrum varied with different incident angles under (C) TE and (D) TM polarized waves.
Conclusion
In conclusion, we designed, fabricated, and measured a kind of MA, which included a top layer of SRR, a middle layer of MAM and a bottom layer of conducting ground. The MA can achieve wide-angle microwave absorption in 1.25–2.31 GHz under normal incidence by utilizing the phenomenon of magnetic squeezing. In the positive resonance region of SRRs, a strong magnetic squeezing effect occurs. That is, more concentrated magnetic field lines are confined within the SRR arrays. This results in significant magnetic field enhancement within the MAM layer, which provides a prerequisite for high-efficiency absorption enhancement at low frequencies. Furthermore, the lattice structure enables the MA to have satisfactory stability for polarization and wide incident angles. To verify our design concept, a prototype with dimensions of 600 × 600 mm2 was fabricated and measured, and the good agreement between simulated and measured results validates our scheme. This design method may find potential applications in fields such as electromagnetic compatibility, wireless communication, and others.
Data Availability Statement
All datasets presented in this study are included in the article/supplementary material.
Author Contributions
ZW: Conceptualization, Methodology, Software, Validation, Writing - original draft, Writing - review and editing. JW: Conceptualization, Investigation, Writing - review & editing. YH, YF, XF, YP, MY, YL, HM, ZX, and SQ: Supervision, Writing - review & editing.
Funding
The authors are grateful to support from the National Natural Science Foundation of China (Grant Nos. 61971435, 61801509, 61901508, 61671466, and 61971341), the Shaanxi Province Scientific and Technology Innovation Team Foundation of Shaanxi Province under Grant No. 2019JZ-40, and the National Key Research and Development Program of China (Grant No.: SQ2017YFA0700201).
Conflict of Interest
The authors declare that the research was conducted in the absence of any commercial or financial relationships that could be construed as a potential conflict of interest.
References
1. Smith DR, Padilla WJ, Vier DC, Nemat-Nasser SC, Schultz S. Composite medium with simultaneously negative permeability and permittivity. Phys Rev Lett. (2000). 84:4184. doi:10.1103/physrevlett.84.4184.
2. Chen H, Wu BI, Zhang B, Kong JA. Electromagnetic wave interactions with a metamaterial cloak. Phys Rev Lett. (2007). 99:063903. doi:10.1103/physrevlett.99.149901.
3. Huang C, Yang J, Wu X, Song J, Pu M, Wang C, et al. Reconfigurable metasurface cloak for dynamical electromagnetic illusions. ACS Photonics. (2017). 5:1718–25. doi:10.1021/acsphotonics.7b01114.
4. Seddon N, Bearpark T. Observation of the inverse Doppler effect. Science. (2003). 302:1537–40. doi:10.1126/science.1089342.
5. Hao J, Wang J, Liu X, Padilla WJ, Zhou L, Qiu M. High performance optical absorber based on a plasmonic metamaterial. Appl Phys Lett (2010). 96:251104. doi:10.1063/1.3442904.
6. Reinhard B, Schmitt KM, Wollrab V, Neu J, Beigang R, Rahm M. Metamaterial near-field sensor for deep-subwavelength thickness measurements and sensitive refractometry in the terahertz frequency range. Appl Phys Lett. (2012). 100:221101. doi:10.1063/1.4722801.
7. Narayan S, Sangeetha B, Sruthi TV, Shambulingappa V, Nair RU. Design of low observable antenna using active hybrid-element FSS structure for stealth applications. AEU - Int J Electron Commun. (2017). 80:137–43. doi:10.1016/j.aeue.2017.06.038.
8. Yong Z, Zhang S, Gong C, He S. Narrow band perfect absorber for maximum localized magnetic and electric field enhancement and sensing applications. Sci Rep. (2016). 6:24063. doi:10.1038/srep24063.
9. Wu C, Neuner B, John J, Milder A, Zollars B, Savoy S, et al. Metamaterial-based integrated plasmonic absorber/emitter for solar thermo-photovoltaic systems. J Optic. (2012). 14:024005. doi:10.1088/2040-8978/14/2/024005.
10. Landy NI, Sajuyigbe S, Mock JJ, Smith DR, Padilla WJ. Perfect metamaterial absorber. Phys Rev Lett. (2008). 100:207402. doi:10.1103/physrevlett.100.207402.
11. Cheng Y, Zou Y, Luo H, Chen F, Mao X. Compact ultra-thin seven-band microwave metamaterial absorber based on a single resonator structure. J Electron Mater. (2019). 48:3939–46. doi:10.1007/s11664-019-07156-z.
12. Bakir M, Karaaslan M, Ünal E, Akgöl O, Sabah C. Microwave metamaterial absorber for sensing applications. Opto-Electron Rev (2017). 25:318–25. doi:10.1016/j.opelre.2017.10.002.
13. Kim YJ, Hwang JS, Yoo YJ, Khuyen BX, Rhee JY, Chen X, et al. Ultrathin microwave metamaterial absorber utilizing embedded resistors. J Phys D Appl Phys. (2017). 50:405110. doi:10.1088/1361-6463/aa82f4.
14. Cheng Y, Cheng Z, Mao X, Gong R. Ultra-thin multi-band polarization-insensitive microwave metamaterial absorber based on multiple-order responses using a single resonator structure. Materials. (2017). 10:1241. doi:10.3390/ma10111241.
15. Zhao J, Cheng Y. Ultrabroadband microwave metamaterial absorber based on electric SRR loaded with lumped resistors. J Electron Mater. (2016). 45:5033–9. doi:10.1007/s11664-016-4693-0.
16. Ramya S, Srinivasa Rao I. A compact ultra-thin ultra-wideband microwave metamaterial absorber. Microw Opt Technol Lett. (2017). 59:1837–45. doi:10.1002/mop.30636.
17. Hoa NTQ, Lam PH, Tung PD. Wide‐angle and polarization‐independent broadband microwave metamaterial absorber. Microw Opt Technol Lett. (2017). 59:1157–61. doi:10.1002/mop.30490.
18. Wang Q, Cheng Y. Compact and low-frequency broadband microwave metamaterial absorber based on meander wire structure loaded resistors. AEU Int J Electron Commun. (2020). 120:153198. doi:10.1016/j.aeue.2020.153198.
19. Ding F, Cui Y, Ge X, Jin Y, He S. Ultra-broadband microwave metamaterial absorber. Appl Phys Lett. (2012). 100:103506. doi:10.1063/1.3692178.
20. Nguyen TT, Lim S. Design of metamaterial absorber using eight-resistive-arm cell for simultaneous broadband and wide-incidence-angle absorption. Sci Rep. (2018). 8:6633. doi:10.1038/s41598-018-25074-8.
21. Shen Y, Zhang J, Meng Y, Wang Z, Pang Y, Wang J, et al. Merging absorption bands of plasmonic structures via dispersion engineering. Appl Phys Lett. (2018). 112:254103. doi:10.1063/1.5040067.
22. Shen Y, Zhang J, Wang W, Pang Y, Wang J, Ma H, et al. Integrating absorber with non-planar plasmonic structure for k-vector matching absorption enhancement. J Appl Phys. (2018). 124:225101. doi:10.1063/1.5068717.
23. Fan Y, Zhang HC, Yin JY, Xu L, Nagarkoti DS, Hao Y, et al. . An active wideband and wide-angle electromagnetic absorber at microwave frequencies. Antennas Wirel Propag Lett. (2016). 15:1913–16. doi:10.1109/lawp.2016.2544399.
24. Li W, Wu T, Wang W, Guan J, Zhai P. Integrating non-planar metamaterials with magnetic absorbing materials to yield ultra-broadband microwave hybrid absorbers. Appl Phys Lett. (2014). 104:022903. doi:10.1063/1.4862262.
25. Wang J, Qu S, Yang Y, Ma H, Wu X, Xu Z. Multiband left-handed metamaterials. Appl Phys Lett. (2009). 95:014105. doi:10.1063/1.3170236.
26. Wang J, Qu S, Xu Z, Zhang J, Ma H, Yang Y, et al. Broadband planar left-handed metamaterials using split-ring resonator pairs. Photonics Nanostruct - Fundam Appl. (2009). 7:108–113. doi:10.1016/j.photonics.2009.01.001.
28. Smith DR, Vier DC, Koschny T, Soukoulis CM. Electromagnetic parameter retrieval from inhomogeneous metamaterials. Phys Rev. (2005). 71:036617. doi:10.1103/physreve.71.036617.
29. Tayde Y, Saikia M, Srivastava KV, Ramakrishna SA. Polarization-insensitive broadband multilayered absorber using screen printed patterns of resistive ink. Antennas Wirel Propag Lett. (2018). 17:2489–93. doi:10.1109/lawp.2018.2879274.
30. Kong X, Xu J, Mo J-J, Liu S. Broadband and conformal metamaterial absorber. Front Optoelectron. (2017). 10:124–131. doi:10.1007/s12200-017-0682-z.
31. Bian B, Liu S, Kong X. Three-dimensional microwave broadband metamaterial absorber with broad transmission window based on the coupled symmetric split ring resonators. J Electromagn Waves Appl. (2016). 30:2153–64. doi:10.1080/09205071.2016.1249417.
Keywords: metamaterial absorber, magnetic squeezing effect, low microwave frequency, wideband absorption, metamaterials
Citation: Wang Z, Wang J, Han Y, Fan Y, Fu X, Pang Y, Yan M, Li Y, Ma H, Xu Z and Qu S (2020) Wideband Absorption at Low Microwave Frequencies Assisted by Magnetic Squeezing in Metamaterials. Front. Phys. 8:595642. doi: 10.3389/fphy.2020.595642
Received: 17 August 2020; Accepted: 22 September 2020;
Published: 03 December 2020.
Edited by:
Xufeng Jing, China Jiliang University, ChinaReviewed by:
Xiangkun Kong, Nanjing University of Aeronautics and Astronautics, ChinaMohammad Rashed Iqbal Faruque, National University of Malaysia, Malaysia
Copyright © 2020 Wang, Wang, Han, Fan, Fu, Pang, Yan, Li, Ma, Xu and Qu. This is an open-access article distributed under the terms of the Creative Commons Attribution License (CC BY). The use, distribution or reproduction in other forums is permitted, provided the original author(s) and the copyright owner(s) are credited and that the original publication in this journal is cited, in accordance with accepted academic practice. No use, distribution or reproduction is permitted which does not comply with these terms.
*Correspondence: Jiafu Wang, wangjiafu1981@126.com; Shaobo Qu, qushaobo@mail.xjtu.edu.cn