- 1Department of Biological Chemistry, School of Medicine, University of California, Irvine, CA, United States
- 2Beckman Laser Institute and Medical Clinic, University of California, Irvine, CA, United States
Since the laser has been invented it has been highly instrumental in ablating different parts of the cell to test their functionality. Through induction of damage in a defined sub-micron region in the cell nucleus, laser microirradiation technique is now established as a powerful real-time and high-resolution methodology to investigate mechanisms of DNA damage response and repair, the fundamental cellular processes for the maintenance of genomic integrity, in mammalian cells. However, irradiation conditions dictate the amounts, types and complexity of DNA damage, leading to different damage signaling responses. Thus, in order to properly interpret the results, it is important to understand the features of laser-induced DNA damage. In this review, we describe different types of DNA damage induced by the use of different laser systems and parameters, and discuss the mechanisms of DNA damage induction. We further summarize recent advances in the application of laser microirradiation to study spatiotemporal dynamics of cellular responses to DNA damage, including factor recruitment, chromatin modulation at damage sites as well as more global damage signaling. Finally, possible future application of laser microirradiation to gain further understanding of DNA damage response will be discussed.
History of Application of Laser Microirradiation in Biology
The application of the microbeam to cell microscopy provided a means for scientists to noninvasively remove subcellular material from living cells. Bessis was the first to modify a part of the cell with this technique by targeting the mitochondria [1]. This provided the foundation for a valuable tool used to mechanically damage subcellular structures without the worry of contamination or membrane rupture inherent with conventional micromanipulation techniques.
The first improvement of micromanipulation of cells with light was a shift in light sources, from the use of ultraviolet (UV) microbeams to classic laser sources. In 1969, Berns and colleagues utilized an argon laser microbeam to successfully irradiate preselected sites on chromosomes [2] and nucleoli [3] sensitized with acridine orange. The intense, coherent nature of the laser resulted in a powerful, focused tool that successfully ablated submicron regions of condensed chromosomes.
Advancement of laser technology provided access to lasers that could provide focal spots with higher energy density. With the development of the Q switched Nd:YAG laser, sensitization of cells was not necessary to successfully irradiate chromosomes [4]. Strahs and Berns then demonstrated via transmission electron microscopy analysis that damage with the Nd:YAG laser at three different wavelengths was identical to damage produced by UV irradiation at 280 nm. Comparison of ultrastructure damage showed that laser ablation of biomolecules was not restricted to specific molecules [5]. Elimination of the need for sensitizing agents was important as their binding to DNA would alter chromatin configuration and skew DNA damage response (DDR).
Short pulsed Nd:YAG lasers with nanosecond pulse durations became a popular and versatile laser source for inducing DNA damage [6–8]. Recently, laser technology has progressed with decreases in pulse duration. Currently the most common laser utilized in the field is the Ti:Sapphire near-infrared (NIR) laser with femtosecond pulse duration [9–14]. König et al described NIR femtosecond laser damage to chromosomes at lower thresholds (not causing membrane rupture) controlled to sub-femtoliter volume and caused by optical breakdown and plasma formation [9]. The most common wavelength used in recent studies vary between 780–800 nm, due to the efficiency of femtosecond pulse width Ti:Sapphire laser and the confinement of the DNA lesion. A comprehensive review by Gassman and Wilson discussed studies using different laser sources (UV, visible, and NIR) both with and without sensitizers [15].
The development of confocal imaging systems brought new potential, where ingenuity of scientists changed the function of imaging lasers to selectively damage nuclear regions by increasing the output power of the lasers. Recently, Gaudreau-Lapierre et al. [16] describes the methodology behind the use of a laser scanning confocal microscope to irradiate regions within the nucleus using the fluorescence-recovery after photobleaching module common with commercially available software for confocal microscopes. Studies utilizing confocal systems to induce DNA damage allowed tracking of the DDR response in 4 dimensions, conventional 3 dimensions (as optical laser can be focused to a specific spot, see below) and the temporal response [16, 17]. Ultraviolet A (UVA) laser wavelength (355–365 nm) is also commonly used for DNA damage induction, including confocal microscope sources [18–21]. The mechanism of DNA damage by UVA laser sources is considered to result from single-photon absorption [22].
Use of lasers to activate compounds is a technique that has grown in recent popularity. Photodynamic therapy in the past has utilized photosensitizers, activated by lasers as clinical treatment for cancer and other diseases. A recent example in this field by Lan et al utilizes the Killer red compound to generate ROS-induced DNA damage at specific sites combining with artificially changing the chromatin states (open and closed) [23]. Yanuk et al. continues the search for photosensitizers with three cholorharmine derivates for photo inducible DNA damage including one that cleanly induces single strand breaks [24]. Efforts to develop tools that can induce specific types of DNA damage with spatiotemporal control continue in the field.
Laser Damage Mechanisms
Laser ablation utilizes energy from photons of light interacting with biomolecules within the targeted cellular structure. A large population of photons of high energy focused to a small beam diameter result in consistent, and targeted regions of damage. Laser induced damage is affected by laser parameters including energy level, pulse duration, number of pulses delivered, and wavelength. Additionally, variation in the physical mechanism of light interaction with biological molecules is also dependent on the laser manufacturer, as well as optimal focusing of the beam through the optical path. The diameter of the induced damage can be controlled to a diffraction-limited spot size defined by 1.22 λ/NA. Damage area can be further extended by repositioning the beam to multiple locations, which in some software is referred to as “zoom factor”.
The combination of laser parameters used dictates the types of DNA damage induced by the laser. We previously utilized multiple laser sources and parameters, such as wavelength, peak irradiance, input power, pulse frequency and duration of exposure, to induce DNA damage, and systematically analyzed the resulting DNA damage by immunofluorescent detection of crosslinking and base damage, recruitment and modification of DDR and pathway-specific repair factors [22]. We proposed four potential mechanisms of laser-induced DNA damage: (i) temperature rise produced by linear or two photon absorption; (ii) generation of large thermo-elastic stresses; (iii) various photochemical processes by linear or two photon absorption including DNA cross-linking damage and production of free radicals and reactive oxygen species; and (iv) optical breakdown (plasma formation) produced by a combination of multiphoton and cascade ionization processes, leading to thermal, mechanical and chemical damage (Figure 1A) [22].
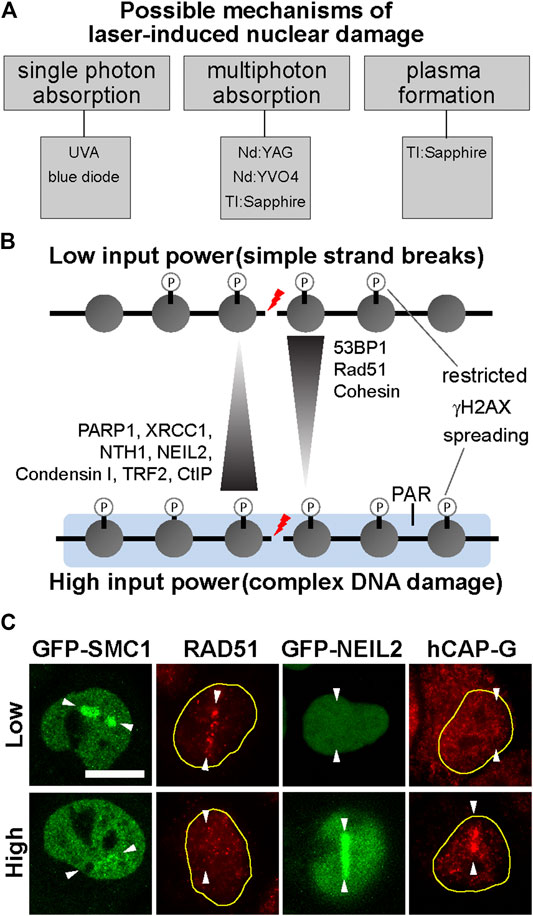
FIGURE 1. Simple strand breaks and complex damage induced by NIR laser microirradiation with low and high input power. By adjusting parameters, laser microirradiation can enrich different types and amounts of DNA damage with the recruitment of different repair factors. (A) Suggested mechanisms of laser induced nuclear damage and corresponding common laser sources for damage induction. Specific wavelength and other laser parameters are summarized in Supplementary Table S1. Modified from [22]. (B) Schematic diagram of differential DNA damage responses to low and high input power NIR laser microirradiation. For relatively simple strand breaks induced by low power laser (top), efficient 53BP1, Rad51 and SMC1 (cohesin) recruitment occurs while no significant PARP activation is observed. In contrast, complex damage containing crosslinking and base damage as well as high-density strand breaks induces robust PARP activation, spreading of H2AX phosphorylation from damage sites (γH2AX, the marker of DSBs), and recruitment of repair factors in NER and BER pathways. Blue box: PAR accumulation [37]. (C) Fluorescent microscope images of human cells damaged with low and high input power laser microirradiation. Cohesin (GFP-SMC1) and Rad51 involved in HR repair are preferentially recruited to low input power damage sites (top) while DNA glycosylase (GFP-NEIL2) and condensin I (hCAP-G) involved in BER/SSB repair are enriched at high input power damage sites (bottom). Damage sites are indicated by white arrowheads. Bar = 10 μm [37, 38].
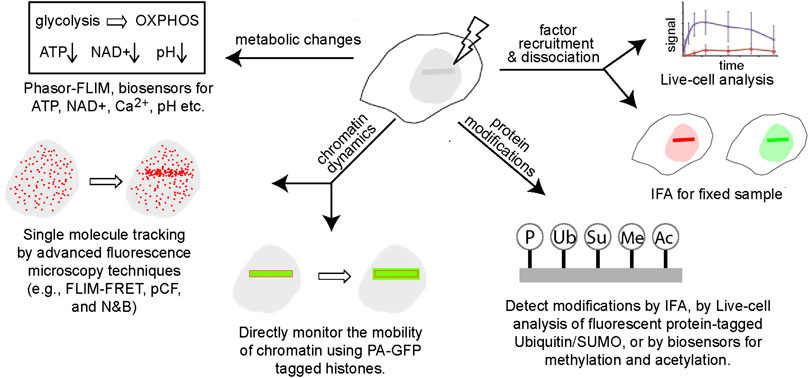
FIGURE 2. Application of laser damage to dissect different aspects of cellular DDRs. Laser microirradiation in combination with a variety of fluorescence dynamics and imaging techniques allows analyses of cellular DDRs with high spatiotemporal resolution, such as repair factor recruitment and dissociation at the damage sites, protein modifications, chromatin dynamics, and metabolic signaling. These techniques include (clockwise) real-time kinetics analysis of fluorescently labeled protein recruitment at laser-induced damage sites, immunofluorescent staining analysis (IFA) of proteins and covalent modifications at damage sites using specific antibodies in fixed cells; damage site chromatin movement using fluorescently labeled histones; high-resolution fluorescence dynamics analyses of molecular movements and oligomerization using fluorescently labeled proteins with advanced fluorescence microscopy techniques, such as FLIM-FRET, pCF and N&B; and analyses of metabolic changes using phasor-FLIM and fluorescence biosensors.
Many studies support the mechanism of multiphoton processes when inducing nuclear damage with short-pulsed lasers. Göppert-Mayer first defined the nonlinear absorption mechanism, which allows DNA and other molecules to absorb multiple photons of lower energy resulting in effects similar to the absorption of a single photon of higher energy [25]. Early studies by Calmettes and Berns demonstrated the phase “paling” of chromosomes and nucleoli suggests this observation was a result of multiphoton processes by 532 nm laser photons and two-photon processes for 266 nm UV light [26]. Phase paling as observed with these multiphoton mechanisms are similar to those resulting from Ti:Sapphire NIR lasers with femtosecond pulse widths [13]. Damage mechanisms of NIR are based on low density plasma formation, and well defined by Vogel and Venugopalan [27, 28]. Damage mechanisms of continuous wave (CW) lasers like the blue diode lasers commonly utilized with confocal imaging systems are based on linear absorption, similar to longer pulse width UV lasers [28].
Induction and Detection of Different Types of DNA Damage and Factor Recruitment
Living cells experience various types of DNA damage that may lead to gene mutations if not corrected. Correspondingly, multiple DNA damage signaling and repair pathways are activated by the damage to maintain genome stability. Photoproducts such as pyrimidine-pyrimidone (6–4) photoproducts (6–4PPs) and cyclobutane pyrimidine dimers (CPDs) caused by UV light are repaired by the nucleotide excision repair (NER) pathway. Base damage, such as oxidation damage 8-oxoguanine (8-oxoG), is repaired via the base excision repair (BER) pathway. DNA double-strand breaks (DSBs) can be repaired by two major pathways, homologous recombination (HR), non-homologous end joining (NHEJ), or alternative/back-up pathways, such as single-strand annealing (SSA) pathway [29]. DNA single-strand breaks (SSB) repair is also the downstream step of BER [30], since base damage is processed by DNA glycosylases and AP endonuclease into a SSB intermediate [22, 31].
As described above, laser microirradiation became a powerful tool to analyze DNA repair in vivo at a single cell resolution. The use of laser ablation with cells expressing fluorescently-tagged DNA repair-related proteins make it possible to study the association and dissociation of repair factors at DNA damage sites with high spatial resolution and tight temporal control. A laser source integrated into a confocal microscope equipped with an incubator for temperature and CO2 control allows time-course analyses of DDR in real time. With advanced fluorescence imaging techniques, it is also possible to investigate cellular responses beyond the repair protein accumulation at damage sites, such as chromatin structural changes and metabolic changes in response to DNA damage [21, 32–35].
The NIR laser-induced damage results in a wide variety of DNA damage types including SSBs, DSBs, and pyrimidine dimers [36]. It was suggested that the use of visible and NIR laser wavelengths may be challenging due to the complexity of induced damage [15]. Complex DNA damage, however, is what is commonly induced by ionizing radiation and genotoxic agents, and thus, it is important to understand the cellular responses to this type of damage. We found that it is possible to control the complexity of DNA lesions by titration of NIR laser input power [37, 38]. Unlike UVC lasers [36], we found that the NIR lasercan generate simple low density SSBs and DSBs at low input power and complex DNA damage (SSBs, DSBs, 6-4PP, CPD, and 8-oxoG) with high input power (Figures 1B,C) [37, 38]. Importantly, complex DNA damage induced by high input-power NIR laser triggers robust poly (ADP-ribose) polymerase 1 (PARP1) recruitment and activation [37]. We found that PARP1 activation at complex damage sites is required for telomeric repeat binding factor 2 (TRF2) recruitment, but inhibits 53BP1 recruitment, having differential effects on DSB repair pathway choice [37, 39] (Figure 1B). PARP1 is a rapid and sensitive DNA nick sensor that catalyzes the synthesis of poly (ADP-ribose) (PAR) chains on itself and target proteins [40]. Local PAR accumulation at damaged lesions was proposed to cause phase separation and recruitment of various factors [41, 42]. 53BP1,which restricts DNA end resection for HR and promotes NHEJ [43, 44], is the first example of repair factor whose recruitment is inhibited by PAR, providing one possible explanation for hyperactivation of NHEJ by PARP inhibitors [37]. Furthermore, we observed accumulation of BER factors, such as DNA glycosylase NEIL1, and condensin I [38, 45] at high power damage sites (Figure 1B). Interestingly, even high-linear energy transfer (LET) α-particles irradiation, which can induce up to 90% complex damage [46] failed to induce robust PARP activation and thus no TRF2 recruitment was observed [47, 48]. This may be due to differences in damage density and induction mechanism. High input power NIR also causes pan-nuclear phosphorylation of H2AX (γH2AX), which disperses MDC1 from damage sites [37]. This is similar to what was reported with high-LET irradiation 49. Because 53BP1 and Rad51 accumulation at the DNA damage sites requires MDC1 50, 51 MDC1 dispersion by pan-nuclear γH2AX also suppresses their clustering at damage sites. Thus, despite the induction of complex DNA damage, the DDR patterns can be different between high-LET IR and high input power NIR. Nevertheless, these studies indicate that with careful titration of laser parameters and characterization of resulting DNA damage by immunofluorescent detection of key damage markers, NIR laser should be a valuable tool to investigate dynamics of DDRs in response to simple strand breaks and complex DNA damage, and is particularly suitable for studying PARP signaling [34, 37–39].
Systematic Studies of Factor Recruitment to Laser-Induced Damage Sites
While laser microirradiation can be used to examine the dynamics of DDR at the single-cell level with high spatiotemporal resolution, it is time-consuming to define laser-irradiated subcellular regions manually and analyze large sets of images. In early DNA damage studies that utilized a laser beam, the number of repair factors tested were limited [36, 52]. The kinetics of individual DNA repair factors at damage sites have been reported [53, 54], but it is difficult to compare these studies because the laser parameters were different, which might have induced different types and amounts of DNA damage [22]. Furthermore, artificial overexpression of the recombinant tagged proteins used for the analyses may cause these proteins to behave differently from the endogenous untagged proteins expressed from their own promoters [37, 55]. To circumvent these potential problems and compare different factor recruitment side by side, bacterial artificial chromosome (BAC)-transduced cell lines expressing EGFP-tagged DNA repair proteins from their endogenous promoters were used for recruitment kinetics study [20]. They systematically measured and mathematically modeled the kinetics of 70 DNA repair proteins to laser-induced DNA damage sites comparing to co-expressed mCherry-tagged PCNA as a control.
In addition to the studies of known repair factor recruitment, laser microirradiation has been employed to identify factors that were not previously known to be involved in DDR and repair. Those include various histone and chromatin remodeling factors that appear to promote efficient DNA repair [17, 56, 58]. However, these previous studies were also limited to investigation of each individual protein factor. Using the lentivirus expression system, Izhar et al. screened hundreds of gene products with potential roles in DNA repair and other nuclear processes, and identified more than 120 proteins that localized to sites of UVA laser-induced DNA damage [59]. Many positive hits were transcription factors that were recruited to DNA damage sites in a PARP-dependent manner. Most of these had not previously been reported to localize to sites of DNA damage.
It is challenging to perform quantitative analyses of factor recruitment kinetics with sufficient statistical power, due to a limited number of cells that can be analyzed at one time. To increase through-put, several approaches have been developed to analyze fluorescence intensity or area under the curve of micro-irradiated regions [54, 60]. Mistrik et al. [61] exposed a bulk of cells simultaneously to a UVA laser beam in a defined pattern of collinear rays. The induced striation pattern was automatically evaluated by custom-coded software, which provides a quantitative assessment of laser-induced phenotypes. Oeck et al. developed an ImageJ-based, high-throughput evaluation tool to standardize and accelerate the quantitative analysis of local protein accumulation at sites of DNA damage [62]. The continuous development of analysis tools will improve high-throughput data analysis of repair factors dynamics at laser induced-DNA damage sites.
Analyses of Secondary Damage Signaling
Chromatin Dynamics
Chromatin dynamics modulates DNA damage site accessibility of repair factors. Damage induction at the define subnuclear region by laser microirradiation allowed tracking of damaged chromatin movement and change of chromatin compaction [17]. Kruhlak et al. [17] tracked mobility and structure of chromatin containing DSBs in living cells by using photoactivatable GFP (PA-GFP)-tagged histone H2B. Initial studies using photo-activatable GFP fused to histone H2B revealed that DNA damage triggers the localized relaxation of chromatin followed by the localized compaction of chromatin, in an ATP and PARP-dependent manner [63, 64]. Several advanced fluorescence microscopy techniques were employed to visualize dynamic chromatin changes at laser induced damaged and undamaged sites [32, 33, 35]. For example, pair correlation function (pCF) analysis of EGFP molecular flow in and out of chromatin before and after damage induction revealed that DNA damage induces a transient decrease in chromatin compaction at the damage site and an increase in compaction to adjacent regions [35]. Dispersion and compaction surrounding the damage site was suggested to facilitate DNA repair factor recruitment to the lesion [35]. Lou et al. were able to directly measure nanometer changes in chromatin compaction at DNA damage sites using a biophysical method based on phasor image-correlation spectroscopy of histone fluorescence lifetime imaging microscopy (FLIM)-Förster resonance energy transfer (FRET) microscopy on live cells coexpressing H2B-eGFP and H2B-mCherry [32]. They found that the fraction of compact chromatin within the NIR laser-induced damage lesion sharply increased in the first 30 min after DSB induction and persisted for up to 3 h, while there was no significant change in the percentage of compact chromatin nucleus-wide [32]. Another group, however, demonstrated that 405 nm laser-induced DNA damage in Hoechst-sensitized cells led to a global compaction of undamaged chromatin through analysis with fluorescence anisotropy imaging of histone H2B-EGFP [33]. The controversial results from different groups may be caused by the different damage conditions (as discussed above). Nevertheless, the combination of laser microirradiation and fluorescence dynamics techniques makes it possible to distinguish changes of chromosome dynamics at damage sites and in the rest of the nucleus (with undamaged chromatin) in response to DNA damage.
Cell-wide Metabolic Response
In addition to studies on DNA damage responses in the nucleus, use of laser microirradiation in conjunction with fluorescence imaging allows investigation of cell-wide DDR because it is possible to distinguish damaged and undamaged cells in the same field under microscope and to track damage-induced changes in realtime. Using fluorescence-based ATP and NAD+ biosensors, pH indicator, and phasor-FLIM capturing autofluorescence of NADH, we systematically measured metabolic dynamics in living cells in response to NIR laser-induced DNA damage with different input power [34]. We observed a rapid cell-wide increase of the bound NADH fraction in response to complex DNA damage, which is triggered by PARP1-dependent transient depletion of NAD+. We found that this change is linked to the increased cellular metabolic reliance to oxidative phosphorylation(oxphos) over glycolysis, which is critical for damaged cell survival [34]. Recent evidence suggests that other cellular processes are also involved in DDR, such as autophagy and immune response [65, 66]. Damage-induced changes in subcellular localization or oligomerization revealed previously unrecognized roles of proteins in DDR and repair processes [67, 68]. Thus, combinatorial use of spatiotemporally defined laser microirradiation and fluorescent imaging will have many future applications in realtime dissection of cellular responses to DNA damage.
Discussion and Future Perspective
With careful parameter setting and characterization of induced damage, laser microirradiation offers valuable opportunities to study in vivo cellular responses to DNA Damage with high spatiotemporal resolution. It was highly instrumental in identifying new factors and modifications involved in DDR, determining the order of factor recruitment and kinetics, chromatin and cellular metabolic changes associated with DNA damage, furthering our knowledge of DNA damage response in human cells (Figure 2). Precise input power titration and parameter setting led to better understanding and control of the types of damage induced, which now allow us to characterize specific DDR signaling associated with simple strand breaks and complex damage. With further technology development and refinement, laser microirradiation will continue to be a powerful tool to study DDR. For example, with CRISPR technology, it is now feasible to generate cell lines that express multiple fluorescently tagged factors from their endogenous loci (to avoid artifactual overexpression) and examine their realtime interplay at laser-induced damage sites using multi-color confocal microscopy. Knowing exactly which cell is damaged, it is also possible to analyze the DDR signaling in cell-cell communication. Advanced fluorescence microscopy techniques, such as number and brightness (N&B) analysis, fluorescence recovery after photobleaching (FRAP), fluorescence localization after photobleaching (FLAP), and FLIM-FRET, may be utilized effectively to further dissect DDR at a single-molecule resolution [69]. Development/improvement of integrated microscopy systems that allow automated laser damage and fluorescent image data acquisition and tracking would allow high-throughput DDR analyses and screening of small molecules, RNAi, or CRISPR libraries to identify critical factors and harness DDR and repair processes, which may be applicable to disease therapy development.
Data Availability Statement
The original contributions presented in the study are included in the article/Supplementary Material, further inquiries can be directed to the corresponding author.
Author Contributions
XK, NW, and KY planned the outline, XK and NW wrote the review under KY’s supervision.
Funding
The work in the Yokomori laboratory was supported in part by NSF MCB-2013798. NW is supported by AFOSR Grant FA9550-20-1-0052.
Conflict of Interest
The authors declare that the research was conducted in the absence of any commercial or financial relationships that could be construed as a potential conflict of interest.
Acknowledgments
We would like to thank Michelle Digman and Enrico Gratton at Laboratory of Fluorescence Dynamics for phasor-FLIM and pCF studies and the support of the Chao Family Comprehensive Cancer Center Optical Biology Core (LAMMP/OBC) Shared Resource for our studies mentioned in this review. We apologize to the researchers whose work we could not cite in this review due to space limitations.
Supplementary Material
The Supplementary Material for this article can be found online at: https://www.frontiersin.org/articles/10.3389/fphy.2020.597866/full#supplementary-material.
References
1. Bessis M, Nomarski G Irradiation ultra-violette des organites cellulaires avec observation continue en contraste de phase. J Cell Biol (1960) 8(3):777–91. doi:10.1083/jcb.8.3.777
2. Berns MW, Olson RS, Rounds DE In vitro production of chromosomal lesions with an argon laser microbeam. Nature (1969) 221(5175):74–5. doi:10.1038/221074a0
3. Berns MW, Olson RS Argon laser micro-irradiation of nucleoli. J Cell Biol (1969) 43(3):621–6. doi:10.1083/jcb.43.3.621
4. Berns MW, Cheng WK, Floyd AD, Ohnuki Y Chromosome lesions produced with an argon laser microbeam without dye sensitization. Science (1971) 171(3974):903–5. doi:10.1126/science.171.3974.903
5. Strahs KR, Berns MW Laser microirradiation of stress fibers and intermediate filaments in non-muscle cells from cultured rat heart. Exp Cell Res (1979) 119(1):31–45. doi:10.1016/0014-4827(79)90332-X
6. Berns MW, Aist J, Edwards J, Strahs K, Girton J, McNeill P, et al. Laser microsurgery in cell and developmental biology. Science (1981) 213(4507):505–13. doi:10.1126/science.7017933
7. Angelov D, Stefanovsky VY, Dimitrov S, Russanova V, Keskinova E, Pashev I Protein-DNA crosslinking in reconstituted nucleohistone, nuclei and whole cells by picosecond UV laser irradiation - PubMed. Nucleic Acids Res (1988) 16(10):4525–38. doi:10.1093/nar/16.10.4525
8. Kim JS, Heale JT, Zeng W, Kong X, Krasieva TB, Ball AR, et al. In Situ analysis of DNA damage response and repair using laser microirradiation. Methods Cell Biol (2007) 82:377–407. doi:10.1016/S0091-679X(06)82013-3
9. König K, Riemann I, Fischer P, Halbhuber KJ Intracellular nanosurgery with near infrared femtosecond laser pulses. Cell Mol Biol (Noisy-le-grand) (1999) 45(2):195–201.
10. Tirlapur UK, König K, Peuckert C, Krieg R, Halbhuber K Femtosecond near-infrared laser pulses elicit generation of reactive oxygen species in mammalian cells leading to apoptosis-like death. Exp Cell Res (2001) 263(1):88–97. doi:10.1006/excr.2000.5082
11. Mari PO, Florea BI, Persengiev SP, Verkaik NS, Brüggenwirth HT, Modesti M, et al. Dynamic assembly of end-joining complexes requires interaction between Ku70/80 and XRCC4. Proc Natl Acad Sci USA (2006) 103(49):18597–602. doi:10.1073/pnas.0609061103
12. Gomez-Godinez V, Wakida NM, Dvornikov AS, Yokomori K, Berns MW Recruitment of DNA damage recognition and repair pathway proteins following near-IR femtosecond laser irradiation of cells. J Biomed Optic (2007) 12(2):020505. doi:10.1117/1.2717684
13. Gomez-Godinez V, Wu T, Sherman AJ, Lee CS, Liaw L-H, Zhongsheng Y, et al. Analysis of DNA double-strand break response and chromatin structure in mitosis using laser microirradiation. Nucleic Acids Res (2010) 38(22):e202. doi:10.1093/nar/gkq836
14. Nadiarnykh O, Thomas G, Van Voskuilen J, Sterenborg HJCM, Gerritsen HC Carcinogenic damage to deoxyribonucleic acid is induced by near-infrared laser pulses in multiphoton microscopy via combination of two- and three-photon absorption. J Biomed Optic (2012) 17(11):116024. doi:10.1117/1.jbo.17.11.116024
15. Gassman NR, Wilson SH Micro-irradiation tools to visualize base excision repair and single-strand break repair. DNA Repair (2015) 31:52–63. doi:10.1016/j.dnarep.2015.05.001
16. Gaudreau-Lapierre A, Garneau D, Djerir B, Coulombe F, Morin T, Marechal A Investigation of protein recruitment to DNA lesions using 405 nm laser micro-irradiation. JoVE (2018) 2018(133). doi:10.3791/57410
17. Kruhlak MJ, Celeste A, Dellaire G, Fernandez-Capetillo O, Müller WG, McNally JG, et al. Changes in chromatin structure and mobility in living cells at sites of DNA double-strand breaks. J Cell Biol (2006) 172(6):823–34. doi:10.1083/jcb.200510015
18. Stixova L, Hruskova T, Sehnalova P, Legartova S, Svidenska S, Kozubek S, et al. Advanced microscopy techniques used for comparison of UVA- and γ-irradiation-induced DNA damage in the cell nucleus and nucleolus. Folia Biol (2014) 60:76–84. doi:10.1039/c1pp05232b
19. Lan L, Nakajima S, Oohata Y, Takao M, Okano S, Masutani M, et al. In situ analysis of repair processes for oxidative DNA damage in mammalian cells. Proc Natl Acad Sci USA (2004) 101(38):13738–43. doi:10.1073/pnas.0406048101
20. Aleksandrov R, Dotchev A, Poser I, Krastev D, Georgiev G, Panova G, et al. Protein dynamics in complex DNA lesions. Mol Cell (2018) 69(6):1046–61. doi:10.1016/j.molcel.2018.02.016
21. Falk M, Lukášová E, Štefančíková L, Baranová E, Falková I, Ježková L, et al. Heterochromatinization associated with cell differentiation as a model to study DNA double strand break induction and repair in the context of higher-order chromatin structure. Appl Radiat Isot (2014) 83:177–85. doi:10.1016/j.apradiso.2013.01.029
22. Kong X, Mohanty SK, Stephens J, Heale JT, Gomez-Godinez V, Shi LZ, et al. Comparative analysis of different laser systems to study cellular responses to DNA damage in mammalian cells. Nucleic Acids Res (2009) 37(9). doi:10.1093/nar/gkp221
23. Wei L, Nakajima S, Levine A, Lan L Novel method for site-specific induction of oxidative DNA damage to study recruitment of repair proteins to heterochromatin and euchromatin. Bio-Protocol (2014) 4(11). doi:10.21769/bioprotoc.1140
24. Yañuk JG, Denofrio MP, Rasse-Suriani FAO, Villarruel FD, Fassetta F, García Einschlag FS, et al. DNA damage photo-induced by chloroharmine isomers: hydrolysis: versus oxidation of nucleobases. Org Biomol Chem (2018) 16(12):2170–84. doi:10.1039/c8ob00162f
25. Göppert-Mayer M Elementary processes with two quantum transitions. Ann Phys (2009) 18(7–8):466–79. doi:10.1002/andp.200910358
26. Calmettes PP, Berns MW Laser-induced multiphoton processes in living cells. Proc Natl Acad Sci USA (1983) 80(23 I):7197–9. doi:10.1073/pnas.80.23.7197
27. Vogel A, Venugopalan V. Mechanisms of pulsed laser ablation of biological tissues. Chem Rev (2003) 103(2):577–644. doi:10.1021/cr010379n
28. Vogel A, Noack J, Hüttman G, Paltauf G. Mechanisms of femtosecond laser nanosurgery of cells and tissues. Appl Phys B Lasers Opt (2005) 81(8):1015–1047. doi:10.1007/s00340-005-2036-6
29. Sallmyr A, Tomkinson AE Repair of DNA double-strand breaks by mammalian alternative end-joining pathways. J Biol Chem (2018) 293(27):10536–49. doi:10.1074/jbc.TM117.000375
30. Mjelle R, Hegre SA, Aas PA, Slupphaug G, Drabløs F, Sætrom P, et al. Cell cycle regulation of human DNA repair and chromatin remodeling genes. DNA Repair (2015) 30:53–67. doi:10.1016/j.dnarep.2015.03.007
31. Abbotts R, Wilson DM Coordination of DNA single strand break repair. Free Radic Biol Med (2017) 107:228–44. doi:10.1016/j.freeradbiomed.2016.11.039
32. Lou J, Scipioni L, Wright BK, Bartolec TK, Zhang J, Pragathi Masamsetti V, et al. Phasor histone FLIM-FRET microscopy quantifies spatiotemporal rearrangement of chromatin architecture during the DNA damage response. Proc Natl Acad Sci USA (2019) 116(15):7323–32. doi:10.1073/pnas.1814965116
33. Kesavan PS, Bohra D, Roy S, Mazumder A Monitoring global changes in chromatin compaction states upon localized DNA damage with tools of fluorescence anisotropy. Mol Biol Cell (2020) 31(13):1403–10. doi:10.1091/mbc.e19-08-0417
34. Murata MM, Kong X, Moncada E, Chen Y, Imamura H, Wang P, et al. NAD+ consumption by PARP1 in response to DNA damage triggers metabolic shift critical for damaged cell survival. Mol Biol Cell (2019) 30(20):2584–97. doi:10.1091/mbc.E18-10-0650
35. Hinde E, Kong X, Yokomori K, Gratton E Chromatin dynamics during DNA repair revealed by pair correlation analysis of molecular flow in the nucleus. Biophys J (2014) 107(1):55–65. doi:10.1016/j.bpj.2014.05.027
36. Dinant C, de Jager M, Essers J, van Cappellen WA, Kanaar R, Houtsmuller AB, et al. Activation of multiple DNA repair pathways by sub-nuclear damage induction methods. J Cell Sci (2007) 120(15):2731–40. doi:10.1242/jcs.004523
37. Cruz GMS, Kong X, Silva BA, Khatibzadeh N, Thai R, Berns MW, et al. Femtosecond near-infrared laser microirradiation reveals a crucial role for PARP signaling on factor assemblies at DNA damage sites. Nucleic Acids Res (2015) 44(3):e27. doi:10.1093/nar/gkv976
38. Kong X, Stephens J, Ball AR, Heale JT, Newkirk DA, Berns MW, et al. Condensin I recruitment to base damage-enriched DNA lesions is modulated by PARP1. PLoS One (2011) 6(8). doi:10.1371/journal.pone.0023548
39. Kong X, Cruz GMS, Silva BA, Wakida NM, Khatibzadeh N, Berns MW, et al. Laser microirradiation to study in vivo cellular responses to simple and complex DNA damage. JoVE (2018) 2018(131):56213. doi:10.3791/56213
40. Ba X, Garg NJ Signaling mechanism of poly(ADP-ribose) polymerase-1 (PARP-1) in inflammatory diseases. Am J Pathol (2011) 178(3):946–55. doi:10.1016/j.ajpath.2010.12.004
41. Smith R, Lebeaupin T, Juhász S, Juhász J, Chapuis C, D’augustin O, et al. Poly(ADP-ribose)-dependent chromatin unfolding facilitates the association of DNA-binding proteins with DNA at sites of damage. Nucleic Acids Res (2019) 47(21):11250–67. doi:10.1093/nar/gkz820
42. Altmeyer M, Neelsen KJ, Teloni F, Pozdnyakova I, Pellegrino S, Grøfte M, et al. Liquid demixing of intrinsically disordered proteins is seeded by poly(ADP-ribose). Nat Commun (2015) 6. doi:10.1038/ncomms9088
43. Bunting SF, Callén E, Wong N, Chen HT, Polato F, Gunn A, et al. 53BP1 inhibits homologous recombination in brca1-deficient cells by blocking resection of DNA breaks. Cell (2010) 141(2):243–54. doi:10.1016/j.cell.2010.03.012
44. Chapman JR, Barral P, Vannier JB, Borel V, Steger M, Tomas-Loba A, et al. RIF1 is essential for 53BP1-dependent nonhomologous end joining and suppression of DNA double-strand break resection. Mol Cell (2013) 49(5):858–71. doi:10.1016/j.molcel.2013.01.002
45. Heale JT, Ball AR, Schmiesing JA, Kim J-S, Kong X, Zhou S, et al. Condensin I interacts with the PARP-1-XRCC1 complex and functions in DNA single-strand break repair. Mol Cell (2006) 21:837–48. doi:10.1016/j.molcel.2006.01.03
46. Carter RJ, Nickson CM, Thompson JM, Kacperek A, Hill MA, Parsons JL Complex DNA damage induced by high linear energy transfer alpha-particles and protons triggers a specific cellular DNA damage response. Int J Radiat Oncol Biol Phys (2018) 100(3):776–84. doi:10.1016/j.ijrobp.2017.11.012
47. Williams ES, Stap J, Essers J, Ponnaiya B, Luijsterburg MS, Krawczyk PM, et al. DNA double-strand breaks are not sufficient to initiate recruitment of TRF2 [4]. Nat Genet (2007) 39(6):696–8. doi:10.1038/ng0607-696
48. Splinter J, Jakob B, Lang M, Yano K, Engelhardt J, Hell SW, et al. Biological dose estimation of UVA laser microirradiation utilizing charged particle-induced protein foci. Mutagenesis (2010) 25(3):289–97. doi:10.1093/mutage/geq005
49. Meyer B, Voss KO, Tobias F, Jakob B, Durante M, Taucher-Scholz G Clustered DNA damage induces pan-nuclear H2AX phosphorylation mediated by ATM and DNA-PK. Nucleic Acids Res (2013) 41(12):6109–6118. doi:10.1093/nar/gkt304
50. Eliezer Y, Argaman L, Rhie A, Doherty AJ, Goldberg M. The direct interaction between 53BP1 and MDC1 Is required for the recruitment of 53BP1 to sites of damage. J Biol Chem (2009) 284(1):426–435. doi:10.1074/jbc.M807375200
51. Zhang J, Ma Z, Treszezamsky A Powell SN. MDC1 interacts with Rad51 and facilitates homologous recombination. Nat Struct Mol Biol (2005) 12(10):902–909. doi:10.1038/nsmb99152
52. Kim JS, Krasieva TB, Kurumizaka H, Chen DJ, Taylor AMR, Yokomori K Independent and sequential recruitment of NHEJ and HR factors to DNA damage sites in mammalian cells. J Cell Biol (2005) 170(3):341–7. doi:10.1083/jcb.200411083
53. Botchway SW, Reynolds P, Parker AW, O’Neill P Use of near infrared femtosecond lasers as sub-micron radiation microbeam for cell DNA damage and repair studies. Mutat Res Rev Mutat Res (2010) 704(1-3):38–44. doi:10.1016/j.mrrev.2010.01.003
54. Mortusewicz O, Evers B, Helleday T PC4 promotes genome stability and DNA repair through binding of ssDNA at DNA damage sites. Oncogene (2016) 35(6):761–70. doi:10.1038/onc.2015.135
55. Muster B, Rapp A, Cristina Cardoso M Systematic analysis of DNA damage induction and DNA repair pathway activation by continuous wave visible light laser micro-irradiation. AIMS Genet (2017) 4(1):47–68. doi:10.3934/genet.2017.1.47
56. Zhu S, Paydar M, Wang F, Li Y, Wang L, Barrette B, et al. Kinesin Kif2C in regulation of DNA double strand break dynamics and repair. Elife (2020) 9. doi:10.7554/eLife.53402
57. Gong F, Clouaire T, Aguirrebengoa M, Legube G, Miller KM Histone demethylase KDM5A regulates the ZMY ND8-NuRD chromatin remodeler to promote DNA repair. J Cell Biol (2017) 216(7):1959–74. doi:10.1083/jcb.201611135
58. Andrin C, McDonald D, Attwood KM, Rodrigue A, Ghosh S, Mirzayans R, et al. A requirement for polymerized actin in DNA double-strand break repair. Nucl (United States) (2012) 3(4):384–95. doi:10.4161/nucl.21055
59. Izhar L, Adamson B, Ciccia A, Lewis J, Pontano-Vaites L, Leng Y, et al. A systematic analysis of factors localized to damaged chromatin reveals PARP-dependent recruitment of transcription factors. Cell Rep (2015) 11(9):1486–500. doi:10.1016/j.celrep.2015.04.053
60. Chen Q, Yu X OGT restrains the expansion of DNA damage signaling—PubMed. Nucleic Acids Res (2016) 2(44):19. doi:10.1093/nar/gkw663
61. Mistrik M, Vesela E, Furst T, Hanzlikova H, Frydrych I, Gursky J, et al. Cells and stripes: a novel quantitative photo-manipulation technique. Sci Rep (2016) 6(1):1–9. doi:10.1038/srep19567
62. Oeck S, Malewicz NM, Krysztofiak A, Turchick A, Jendrossek V, Glazer PM High-throughput evaluation of protein migration and localization after laser micro-irradiation. Sci Rep (2019) 9(1):1–7. doi:10.1038/s41598-019-39760-8
63. Burgess RC, Burman B, Kruhlak MJ, Misteli T Activation of DNA damage response signaling by condensed chromatin. Cell Rep (2014) 9(5):1703–17. doi:10.1016/j.celrep.2014.10.060
64. Khurana S, Kruhlak MJ, Kim J, Tran AD, Liu J, Nyswaner K, et al. A macrohistone variant links dynamic chromatin compaction to BRCA1-dependent genome maintenance. Cell Rep (2014) 8(4):1049–62. doi:10.1016/j.celrep.2014.07.024
65. Galati S, Boni C, Gerra M, Lazzaretti M, Buschini A Autophagy: a player in response to oxidative stress and DNA damage. Oxid Med Cell Longev (2019) 2019. doi:10.1155/2019/5692958
66. Liu H, Zhang H, Wu X, Ma D, Wu J, Wang L, et al. Nuclear cGAS suppresses DNA repair and promotes tumorigenesis. Nature (2018) 563(7729):131–6. doi:10.1038/s41586-018-0629-6
67. Bennetzen MV, Kosar M, Bunkenborg J, Payne MR, Bartkova J, Lindström MS, et al. DNA damage-induced dynamic changes in abundance and cytosol-nuclear translocation of proteins involved in translational processes, metabolism, and autophagy. Cell Cycle (2018) 17(17):2146–63. doi:10.1080/15384101.2018.1515552
68. Hinde E, Yokomori K, Gaus K, Hahn KM, Gratton E Fluctuation-based imaging of nuclear Rac1 activation by protein oligomerisation. Sci Rep (2014) 4(4219):1–9. doi:10.1038/srep04219
Keywords: laser microirradiation, DNA damage response, PARP1, complex DNA damage, chromatin, metabolism
Citation: Kong X, Wakida NM and Yokomori K (2021) Application of Laser Microirradiation in the Investigations of Cellular Responses to DNA Damage. Front. Phys. 8:597866. doi: 10.3389/fphy.2020.597866
Received: 22 August 2020; Accepted: 30 November 2020;
Published: 25 January 2021.
Edited by:
Petra Granitzer, University of Graz, AustriaReviewed by:
Nirmal Mazumder, Manipal Academy of Higher Education, IndiaMartin Falk, Academy of Sciences of the Czech Republic, Czechia
Copyright © 2021 Kong, Wakida and Yokomori. This is an open-access article distributed under the terms of the Creative Commons Attribution License (CC BY). The use, distribution or reproduction in other forums is permitted, provided the original author(s) and the copyright owner(s) are credited and that the original publication in this journal is cited, in accordance with accepted academic practice. No use, distribution or reproduction is permitted which does not comply with these terms.
*Correspondence: Kyoko Yokomori, a3lva29tb3JAdWNpLmVkdQ==
†These authors have contributed equally to this work