- 1Prokhorov General Physics Institute of the Russian Academy of Sciences, Moscow, Russia
- 2V. M. Gorbatov Federal Research Center for Food Systems of Russian Academy of Sciences, Moscow, Russia
The paper investigates the effect of gases dissolved in water on the processes occurring during the laser breakdown of colloidal solutions of nanoparticles. The dynamics of the dependences of the plasma luminosity and acoustic signals on the concentration of nanoparticles under irradiation of colloids of nanoparticles saturated with air, argon, and molecular hydrogen has been studied. It is shown that irradiation of colloids saturated with molecular hydrogen and argon leads to an increase in the integral luminosity and integral acoustic signals in comparison with the control sample saturated with atmospheric gases, which indicates the obvious presence of the influence of gases dissolved in the liquid on the optical breakdown process. The most intense acoustic signals, as well as the brightest breakdowns, were observed when the colloidal solution was saturated with molecular hydrogen.
Introduction
More than a century ago it became clear that the chemical properties of liquids are partly determined by the gases dissolved in them. In physical processes, the role of gases dissolved in liquids has been neglected for a long time. Everything changed after the discovery of the oxygen effect in tumor radiotherapy [1]. The oxygen effect is the property of molecular oxygen present in cells and tissues to enhance the biological effect of ionizing radiation [2]. It is known that the radiolysis of water produces hydroxyl radicals, protons, and electrons [3]. In the absence of oxygen, a reactive hydroxyl radical can indirectly damage biological molecules. Protons and electrons have a lesser effect on biological molecules [4]. Molecular oxygen is an effective electron acceptor, while a superoxide anion radical is formed from molecular oxygen, which can effectively damage biological molecules [5]. In this case, the electron concentration decreases significantly. Later, the oxygen effect was discovered when aqueous solutions were exposed to elevated temperatures [6], ultraviolet radiation [7], visible and infrared [8] radiation.
During the process of optical breakdown, the formation of plasma is observed, in which free electrons play an important role in the absorption of optical radiation [9]. The basis for optical breakdown is the effect of the development of an avalanche of electrons [10]. In this case, seed electrons appear as a result of multiphoton ionization of molecules or gas atoms [11]. When laser radiation interacts with an atom, several photons are absorbed. The ionization of matter occurs, accompanied by the appearance of a free electron. The electron is accelerated in the field of a laser wave and, when it collides with other atoms, ionizes them, giving rise to another electron. Then, two electrons are accelerated by the field and, in collisions with atoms, two more electrons are generated. Thus, an avalanche-like increase in the number of free electrons occurs [12]. It is assumed that, upon the optical breakdown of aqueous solutions, gases dissolved in water can significantly affect the process of an avalanche-like increase in the number of electrons. For example, it is supposed that oxygen, as an electron acceptor, can significantly slow down the development of an electron avalanche. Inert gases should not significantly affect the process, and gases capable of donating an electron should accelerate the development of an avalanche of electrons. This assumption was experimentally verified in work using aqueous colloidal solutions of nanoparticles saturated with atmospheric gases, argon, and molecular hydrogen.
Methods
Cu nanoparticles were obtained using the technique of laser ablation of solid metal targets in liquid. Milli-Q water in an amount of 15 ml was used as a working liquid for laser ablation. A detailed description of this technique can be found in ref. 13. The resulting colloids of nanoparticles were analyzed using a DC24000 analytical disk centrifuge (CPS Instruments). The obtained Cu nanoparticles had a spherical shape and a unimodal size distribution with an average diameter of d = 82 ± 11 nm.
Atmospheric gases, molecular hydrogen, and argon were used in the experiments. Argon under pressure in cylinders, purity 99.987% (Experimental Technological Plant, Protvina, Russia). A GVCh-6 molecular hydrogen generator was used as a source of molecular hydrogen (the purity of the hydrogen produced using the generator was 99.995%).
Before the experiment, the Milli-Q water in which the air was initially dissolved was saturated with gases using bubbling. In this case, the selected gas was bubbled through a thinned perforated capillary [14]. Water in an amount of 20 ml was placed in an experimental cuvette and bubbled until complete saturation. The saturation was monitored by the concentration of molecular oxygen. Initially, there is about 270 μM molecular oxygen in water at room temperature and atmospheric pressure. It should be noted that it was assumed in the experiment that the concentration of molecular oxygen in the cuvette did not change during the entire experiment. However, this assumption is not entirely correct, since it is known that additional concentrations of dissolved oxygen can arise both with an increase in the temperature of the liquid [15] and the presence of cavitation gas bubbles [16]. When bubbling through a solution of molecular hydrogen and argon, the concentration of molecular oxygen decreases to 25–30 μM. The solution was considered saturated with argon and molecular oxygen after no decrease in the concentration of molecular oxygen for half an hour. Saturated solutions contained 618 μM molecular hydrogen and ∼1,450 μM argon. In the case of molecular hydrogen, the concentration was monitored directly, and the argon concentration was estimated. A schematic view of the experimental setup is shown in Figure 1.
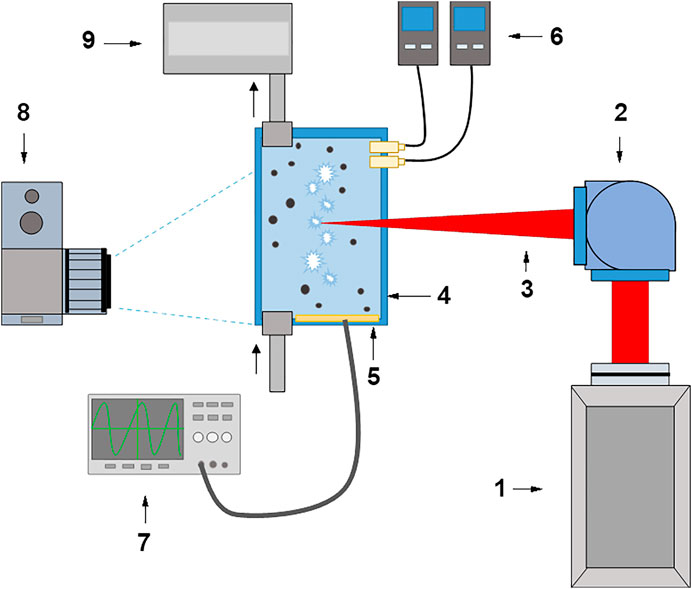
FIGURE 1. Scheme of the experimental setup. 1 - Nd: YAG laser; 2 - Galvano-mirror system; 3 - Laser radiation; 4 - Cuvette with colloidal solution; 5 - Film piezoelectric sensor; 6 - H2 and O2 analyzers; 7 - Digital oscilloscope; 8 - Digital camera; 9 –Gases supply system.
Cu nanoparticles (seeds for optical breakdown) were added to a liquid already saturated with molecular hydrogen or argon to avoid oxidation or reduction. The experiments were carried out in the concentration range of 105–1011 nanoparticles/cuveml. The obtained colloidal solution of nanoparticles in a gas-saturated liquid was then irradiated by a Nd: YAG laser with λ = 1,064 nm, τ = 10 ns, ν = 10 kHz, Ep = 2 mJ. The laser beam was focused using a galvano-mirror system in the center of a glass cuvette filled with an aqueous solution of Fe nanoparticles and could move with speed 3,000 mm/s along a line 10 mm long inside the cuvette. The movement of the laser beam is required to initiate optical breakdown in an unperturbed liquid. It is known from preliminary experiments that changes in the parameters of the laser beam movement can lead to changes in the measured breakdown parameters. The values of the beam movement speed and the length of the movement line are chosen so as to maximize the measured parameters. After irradiation for 1 min, the colloidal solution was removed from the cuvette, after which a solution of nanoparticles of a different concentration was prepared.
As a result of experiments on irradiation of a solution of copper nanoparticles in water saturated with gases, characteristic acoustic spectra of optical breakdown and images of optical breakdown plasma were obtained, Figure 2. Figure 2A depicts the acoustic spectrum observed after the optical breakdown. Acoustic vibrations caused by the optical breakdown of the colloidal solution were recorded using film piezoelectric sensors, the signal from which was fed to a digital oscilloscope (GW Instek GDS-72204E). The method for recording acoustic pulses was described earlier [17]. The acoustic spectrum (Figure 2A left) shows two peaks (0 and 90 µs). The first peak corresponds to optical breakdown followed by the formation of a breakdown plasma. After the breakdown, a cavitation area is formed in the colloidal solution of nanoparticles, then a cavitation bubble is formed, and its expansion is observed. This process takes approximately 90 microseconds. The second peak corresponds to the bubble collapse. In the experiments, the amplitudes of these peaks, their area, and half-width were estimated (Figure 2A, right). The amplitude, half-width and area under the peak were chosen as the measured parameters of the acoustic signal in order to obtain the most detailed information on how the acoustic signal changes depending on the influence and what effect the dissolved gases have on this dependence. The most obvious in terms of physical meaning is the amplitude of the cavitation signal. The magnitude of the amplitude determines both the bubble lifetime and the amplitude of the collapse amplitude. In turn, the signal of the magnitude of the cavitation amplitude is determined by the magnitude of the cavitating region. The acoustic spectrum shown in the figure has negative signal values, since the initial atmospheric pressure was selected as zero pressure. The acoustic signal recorded during optical breakdown is associated with an increased pressure wave, and negative values are associated with the re-reflection of the pressure wave from the wall of the cell to which the sensor is attached. However, in the spectrum, the amplitude of the acoustic signal was measured from the level at a distance of 10–15 μs from the peak. Also, the integral area of acoustic signals in the spectrum was measured. Multiple breakdowns were recorded at oscilloscope sweep times of 200 μs. Integration was performed over the entire resulting oscilloscope sweep with several acoustic spectra.
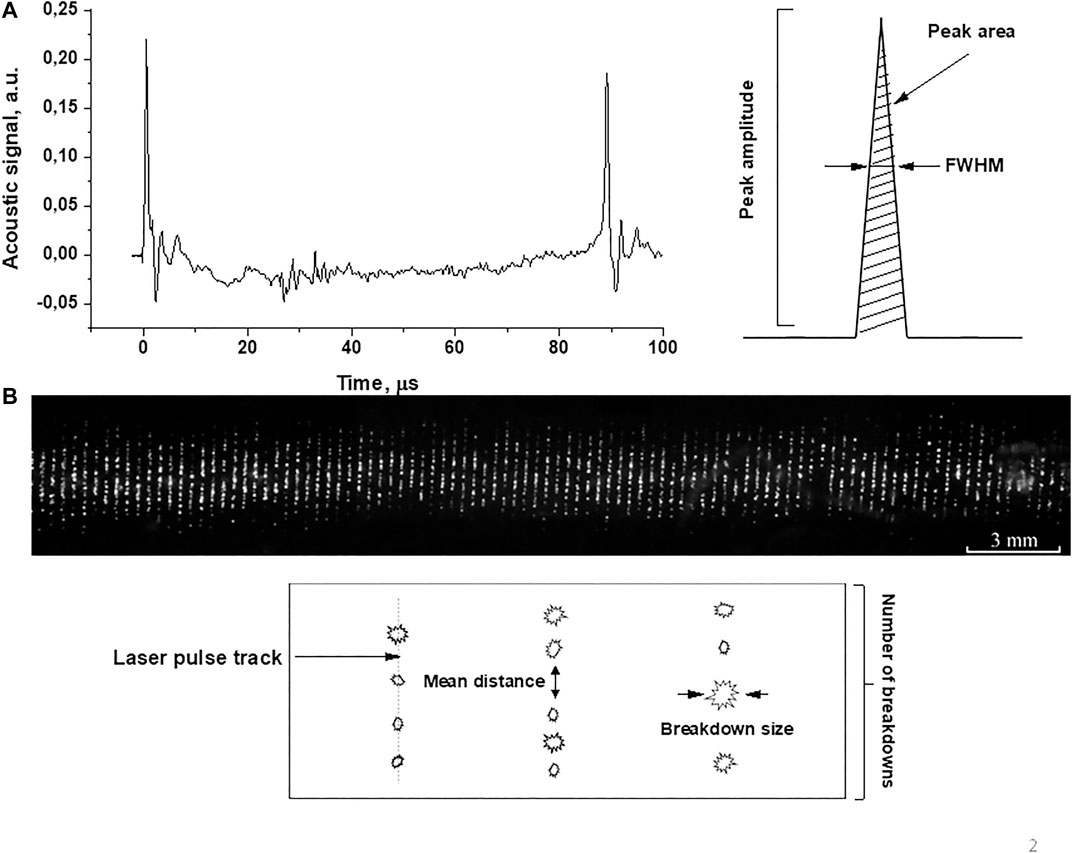
FIGURE 2. (A) Acoustic spectrum and (B) image of laser breakdown plasma upon irradiation of a colloidal solution of Cu nanoparticles unsaturated with gases (n = 1010 pcs/ml). The parameters measured in experiments are shown schematically in the figure.
Figure 1 demonstrates the tracks of laser pulses (vertical lines). Each line consists of separate breakdowns (Figure 1 below). In the experiments, the distance between breakdowns in one track, the size of individual breakdowns, and the glow intensity were estimated. Breakdown plasma images were recorded using a 75D digital video camera (Canon, Japan). The analysis of the obtained images was carried out using the LaserImage program, a detailed description of which can be found in ref. 17. To download the LaserImage program see ref. 18.
Results and Discussion
In the experiments, the influence of the concentration of copper nanoparticles on the acoustic signals and light signals of an optical breakdown plasma under irradiation of aqueous solutions saturated with molecular hydrogen and argon was studied. The results of the study of acoustic signals are shown in Figure 3. The influence of argon and hydrogen dissolved in the colloid is noticeable in the example of the dependencies of the amplitude and area of the acoustic signal on the concentration of nanoparticles, Figures 3A,C. It can be seen from the presented results that acoustic signals in colloids with dissolved argon and hydrogen become more intense over the entire studied concentration range. It should be noted that the half-width of the acoustic signals changes non-monotonically with an increase in the concentration of nanoparticles, but the effect of dissolved gases on the half-width of the signal remains insignificant, Figure 3B.
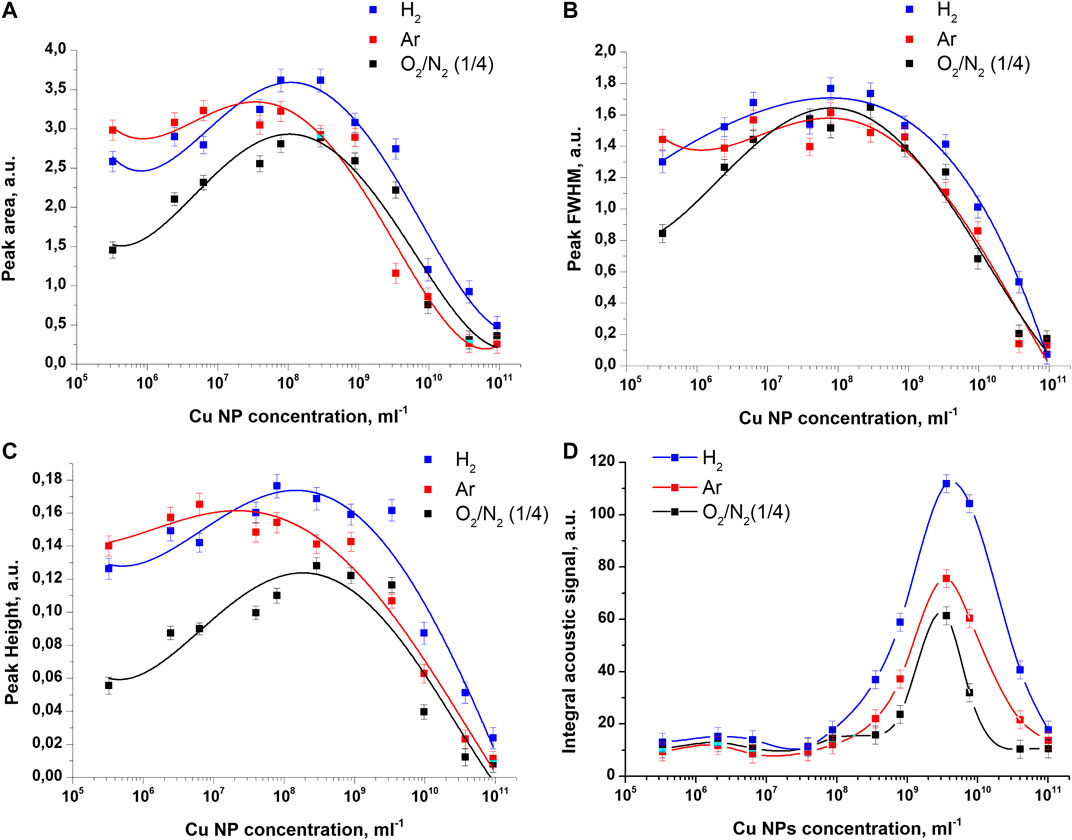
FIGURE 3. Influence of dissolved gases on the characteristics of acoustic signals recorded during the optical breakdown of colloidal solutions of copper nanoparticles. Dependence of the (A) area under the acoustic signal (B) half-width (C) amplitude and (D) integral area of the acoustic signal on the concentration of Cu nanoparticles upon irradiation of colloidal solutions of nanoparticles with dissolved air, argon, and molecular hydrogen.
Figure 2 shows the effect of gases dissolved in colloidal solutions on the intensity of integral acoustic signals at various concentrations of nanoparticles. It was found that irradiation of a colloid of copper nanoparticles saturated with molecular hydrogen leads to the most intense acoustic signals during the optical breakdown. This is evidenced by the magnitude of the peak of acoustic signals at a nanoparticle concentration of 3 × 109 pcs/ml. The maximum value of the integral signal in the case of hydrogen turns out to be 80% higher than the value of the analogous maximum in the case of dissolved air. Irradiation of colloids saturated with argon also leads to an increase in the maximum value of the integral acoustic signals by about 20% in comparison with air. It should be emphasized that when measuring integral acoustic signals the effect of the dissolution of gases in a colloid is observed only at high concentrations of nanoparticles in the range from 2 × 108 pcs/ml to 1011 pcs/ml.
The shift in the position of the maxima of the amplitude, half-width, and area of the acoustic signal from the position of the maximum of the integral acoustic signal can be directly related to the number of breakdowns observed in the experiment. Since the integral acoustic signal is proportional not only to the amplitude of the acoustic signals, but also to the number of breakdowns, which grows exponentially up to high concentrations of nanoparticles (1011 pcs/ml), as a result, the value of the maximum is shifted toward high concentrations.
The results of studying the light signals of the optical breakdown plasma are shown in Figure 4. It was found that the total number of breakdowns increases exponentially with an increase in the concentration of nanoparticles (Figure 4A). In colloids saturated with argon and hydrogen, the recorded number of breakdowns turns out to be greater than in a colloid saturated with atmospheric gases. The effect of dissolved gases becomes especially noticeable when using high concentrations of nanoparticles 1010–1011 pcs/ml. So, for example, at a nanoparticle concentration of 4 × 1010 pcs/ml, the number of recorded breakdowns for the case with hydrogen, argon, and air is 1,800, 1,300, and 900 pcs, respectively.
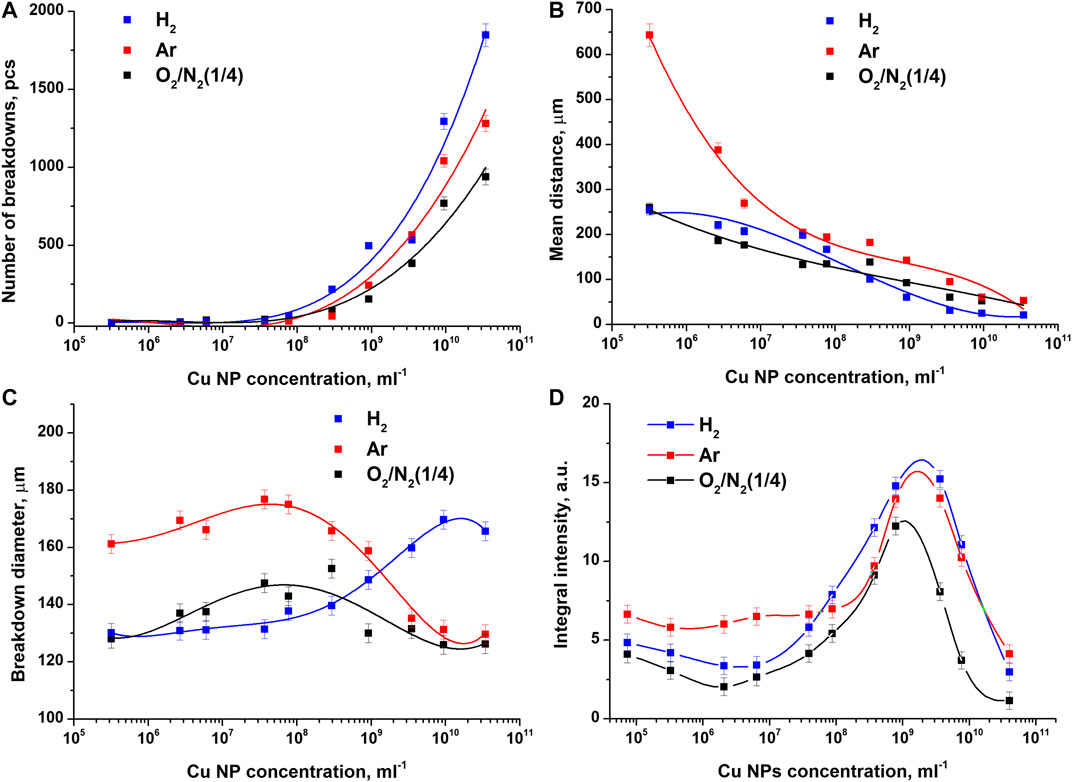
FIGURE 4. Influence of dissolved gases on the characteristics of plasma luminescence recorded during the optical breakdown of colloidal solutions of copper nanoparticles. Dependence of (A) the number of breakdowns (B) the average distance between breakdowns (C) the intensity of an individual breakdown, and (D) the total flash intensity on the concentration of Cu nanoparticles upon irradiation of colloidal solutions of nanoparticles saturated with atmospheric gases, argon, and molecular hydrogen.
As the concentration of nanoparticles increases, the average distance between breakdowns monotonically decreases in all irradiated samples of colloidal solutions (Figure 4B). It should be noted that at low concentrations of nanoparticles (105–107 pcs/ml), the largest average distance between adjacent breakdowns in one track is observed in samples saturated with argon.
The average sizes of individual breakdowns change non monotonically with an increase in the concentration of nanoparticles and turn out to be approximately 20% larger when a colloid saturated with argon is irradiated over a sufficiently large concentration range n = 105 pcs/ml–109 pcs/ml, Figure 4C. With a subsequent increase in the concentration of nanoparticles, the average size of individual breakdowns in a colloid saturated with hydrogen turns out to be the largest, while the size of breakdowns in other samples decreases. It follows from Figure 4C that, starting with nanoparticle concentrations of 4 × 109 pcs/ml and higher, breakdowns in a colloid saturated with hydrogen have the largest average size. The diameter of such breakdowns turns out to be 20–25% larger than the diameter of breakdowns in the case of argon and air.
Figure 3 shows that the saturation of a colloidal solution of copper nanoparticles with argon or hydrogen significantly affects the integral luminosity of optical breakdown plasma. It has been established that, regardless of the type of gas, the maximum values of the integral luminosity of the plasma are observed at a nanoparticle concentration of 109–1010 pcs/ml. With an increase or decrease in the concentration of nanoparticles, the luminosity intensity noticeably decreases. The most intense integral plasma luminosity at a nanoparticle concentration of 109–1010 pcs/ml is observed when a colloidal solution of copper nanoparticles is saturated with molecular hydrogen. The value of the integral intensity turns out to be 20% higher than the intensity when the control colloid with dissolved air is irradiated. A less intense breakdown in luminosity is observed upon irradiation of a colloidal solution saturated with argon. In this case, the differences in the values of the maximum integral intensity are about 12%. It is important to note that during the optical breakdown of colloidal solutions of nanoparticles at concentrations of 105–108 pcs/ml, the highest plasma luminosity is observed in the presence of argon.
It follows from the above results that, upon the optical breakdown of colloids of copper nanoparticles, the process of plasma formation and the form of the acoustic spectrum of shock waves both are influenced by gases dissolved in the irradiated colloid. The obtained experimental data indicate the following general tendency upon irradiation of colloids of nanoparticles with dissolved gases. At low concentrations of nanoparticles in the range from 105 to 107 pcs/ml, the presence of argon or molecular hydrogen in the colloid leads to an increase in the overall plasma brightness and intensification of acoustic signals in comparison with solutions saturated with atmospheric gases. At the same time, solutions saturated with argon at these concentrations demonstrate the highest brightness and the largest amplitude and area of acoustic signals, Figures 3, 4. At the peak values of luminosity and acoustics at concentrations from 109 to 1010 pcs/ml, a different picture is observed. In this case, colloids with dissolved argon and molecular hydrogen also demonstrate an increase in plasma brightness and acoustics compared to air, except that now colloids with molecular hydrogen demonstrate the highest values of the studied parameters. With a further increase in concentration to 1011 pcs/ml, a general tendency is observed in all samples, characterized by a sharp decrease in plasma luminosity and the intensity of acoustic signals.
It is known that the optical breakdown of aqueous solutions can occur on nanosized gas bubbles - bubstones [19]. It was shown that this process depends on the gas concentration [20]. Can the effects observed in the above experiments be explained using this concept? Probably not, since the saturation concentrations of argon, molecular hydrogen, and oxygen in water do not qualitatively correspond to the effects observed in the work. Perhaps this mechanism plays a role, but not the primary one.
It is known that the formation of a breakdown plasma in gases is directly related to the concentration of electrons capable of participating in the formation of an electron avalanche [21]. In the case of optical breakdown in liquids, one should take into account the influence of the environment on the development of an electron avalanche [22]. From the experimental results presented above, it follows that optical breakdown occurs in three fundamentally different cases, depending on which gases are dissolved in the liquid.
In the case of air, a certain concentration of oxygen molecules is present in a colloidal solution, which is a strong oxidizing agent and an electron acceptor. Oxygen molecules can inhibit the development of an avalanche of electrons by capturing electrons and forming a superoxide anion radical. This interaction ultimately leads to a decrease in the concentration and energy of electrons in the breakdown plasma, which, in turn, will lead to a decrease in the brightness of the plasma flash and the fraction of energy converted into acoustic oscillations. Irradiation of colloidal solutions saturated with argon, in turn, leads to the opposite effect since there are almost no oxygen molecules in such a solution, which prevent the development of breakdown.
In the case of colloidal solutions saturated with molecular hydrogen, the following scenario is likely to occur. Hydrogen molecules, possessing a reduction potential in this case, are a source of additional electrons, thus contributing to the development of an electron avalanche. That is why, during the optical breakdown of colloidal solutions saturated with hydrogen, the highest values of both the plasma luminosity and the intensity of acoustic signals are observed (Figures 3, 4), which is especially noticeable at high particle concentrations.
Since water itself is a multicomponent heterogeneous system containing, among other things, different amounts of dissolved gases and nanobubbles, the course of processes in aqueous solutions can be influenced by the composition of water, as well as external conditions affecting its composition.
Conclusion
The paper investigates the effect of the influence of dissolved gases on the processes occurring during the optical breakdown of colloidal solutions of copper nanoparticles. It is shown that the presence of various gases in a colloidal solution, which can appear, for example, upon intense stirring of solutions, leads to a significant change in the acoustic and optical parameters that characterize the laser breakdown plasma. It has been found that molecular hydrogen dissolved in a colloidal solution has the strongest effect on the breakdown process at high concentrations of nanoparticles (108–1011 pcs/ml). Thus, when analyzing the properties of solutions, it is necessary to take into account the presence of gas bubbles in them, as well as take into account the importance of processes leading to the formation of gas bubbles in a solution (for example, shaking, mixing, other processes leading to saturation of solutions with gases), since they can significantly change the physicochemical characteristics of the resulting solutions.
Data Availability Statement
The raw data supporting the conclusions of this article will be made available by the authors, without undue reservation.
Author Contributions
IB and SG conducted experiments. IB, AL, and SG participated in the processing of the results and their discussion. IB and SG participated in writing the text of the manuscript.
Funding
This work was supported by a grant of the Ministry of Science and Higher Education of the Russian Federation for large scientific projects in priority areas of scientific and technological development (grant number 075-15-2020-775). Part of the research related to the production of nanoparticles was supported by the Russian Foundation for Basic Research (19-02-00061).
Conflict of Interest
The authors declare that the research was conducted in the absence of any commercial or financial relationships that could be construed as a potential conflict of interest.
Acknowledgments
The authors are grateful to the Center for Collective Use of the GPI RAS for the equipment provided.
References
1. Gray LH, Conger AD, Ebert M, Hornsey S, Scott OC. The concentration of oxygen dissolved in tissues at the time of irradiation as a factor in radiotherapy. Br. J. Radiol (1953) 312:638–48. doi:10.1259/0007-1285-26-312-638
2. Thoday J, Read J. Effect of oxygen on the frequency of chromosome aberrations produced by X-rays. Nature (1947) 160:608. doi:10.1038/160608a0
3. Ward JF. DNA damage produced by ionizing radiation in mammalian cells: identities, mechanisms of formation, and reparability. Prog. Nucleic Acid Res. Mol. Biol (1988) 35:95–125. doi:10.1016/S0079-6603(08)60611-X
4. Hutchinson F. Chemical changes induced in DNA by ionizing radiation. Prog. Nucleic Acid Res. Mol. Biol (1985) 32:115–54. doi:10.1016/s0079-6603(08)60347-5
5. McCord JM, Fridovich I. The biology and pathology of oxygen radicals. Ann. Intern. Med (1978) 89(1):122–7. doi:10.7326/0003-4819-89-1-122
6. Shtarkman IN, Gudkov SV, Chernikov AV, Bruskov VI. Formation of hydrogen peroxide and hydroxyl radicals in aqueous solutions of L-amino acids by the action of X-rays and heat. Biofizika (2008) 53(1):5–13.
7. Wlaschek M, Briviba K, Stricklin GP, Sies H, Scharffetter-Kochanek K. Singlet oxygen may mediate the ultraviolet A-induced synthesis of interstitial collagenase. J. Invest. Dermatol (1995) 104(2):194–8. doi:10.1111/1523-1747.ep12612751
8. Zakharov SD, Ivanov AV. Light-oxygen effect in cells and its potential applications in tumour therapy. Quant. Electron (1999) 29(12):1031. doi:10.1070/QE1999v029n12ABEH001629
9. Vogel A, Nahen K, Theisen D, Noack J. Plasma formation in water by picosecond and nanosecond Nd:YAG laser pulses. I. optical breakdown at threshold and superthreshold irradiance. IEEE J. Sel. Top Quant. Electron (1996) 2-4:847–60. doi:10.1109/2944.577307
10. Fan CH, Sun J, Longtin JP. Breakdown threshold and localized electron density in water induced by ultrashort laser pulses. J. Appl. Phys (2002) 91:2530. doi:10.1063/1.1433929
11. Alberti A, Munafò A, Koll M, Nishihara M, Pantano C, Freund JB, et al. Laser-induced non-equilibrium plasma kernel dynamics. J. Phys. D Appl. Phys (2019) 53:025201. doi:10.1088/1361-6463/ab492a
12. DellʼAglio M, Gaudiuso R, De Pascale O, and De Giacomo A. Mechanisms and processes of pulsed laser ablation in liquids during nanoparticle production. Appl. Surf. Sci (2015) 4:348–9. doi:10.1016/j.apsusc.2015.01.082
13. Baimler I, Simakin A, Uvarov O, Volkov M, Gudkov S. Generation of hydroxyl radicals during laser breakdown of aqueous solutions in the presence of Fe and Cu nanoparticles of different sizes. Phys. Wave Phenom (2020) 107:28–110. doi:10.3103/S1541308X20020028
14. Baimler IV, Gudkov SV, Sarimov RM, Simakin AV, Shcherbakov IA. Concentration dependences of molecular oxygen and hydrogen in aqueous solutions. Dokl. Phys (2020) 65:5–7. doi:10.1134/S1028335820010085
15. Kell GS. Effects of isotopic composition, temperature, pressure, and dissolved gases on the density of liquid water. J. Phys. Chem. Ref. Data (1977) 6(4):1109–31. doi:10.1063/1.555561
16. Prosperetti A. The speed of sound in a gas–vapour bubbly liquid. Interface focus (2015) 5(5):20150024. doi:10.1098/rsfs.2015.0024
17. Simakin A, Astashev M, Baimler I, Uvarov O, Voronov V, Vedunova M, et al. The effect of gold nanoparticle concentration and laser fluence on the laser-induced water decomposition. J. Phys. Chem. B (2019) 123(8):1869–80. doi:10.1021/acs.jpcb.8b11087
18. Astashev ME, Gudkov SV. Prokhorov General Physics Institute of the Russian Academy of Sciences. Program for investivating plasma luminescence during optical breakdown of medium on individual nanoparticles. Available from: https://drive.google.com/drive/folders/1YRNF2p7qpejlGP55QBiqM108LSGAseaE (Accessed October 20, 2020).
19. Bunkin NF, Bakum SI. Role of a dissolved gas in the optical breakdown of water. Quant. Electron 36(2):117. doi:10.1070/QE2006v036n02ABEH013113
20. Bunkin NF, Bunkin FV. Bubston structure of water and electrolyte aqueous solutions. Phys. Usp (2016) 59(9):846. doi:10.3103/S1541308X13020015
21. Barcikowski S, Plech A, Suslick KS, Vogel A. Materials synthesis in a bubble. MRS Bull (2019) 44(5):382–91. doi:10.1557/mrs.2019.107
Keywords: laser radiation, nanoparticles, dissolved gases, argon, molecular hydrogen, optical breakdown
Citation: Baimler IV, Lisitsyn AB and Gudkov SV (2020) Influence of Gases Dissolved in Water on the Process of Optical Breakdown of Aqueous Solutions of Cu Nanoparticles. Front. Phys. 8:622775. doi: 10.3389/fphy.2020.622775
Received: 29 October 2020; Accepted: 24 November 2020;
Published: 17 December 2020.
Edited by:
Nikolai F. Bunkin, Bauman Moscow State Technical University, RussiaReviewed by:
Nikita Penkov, Institute of Cell Biophysics, RussiaVladimir Sivakov, Leibniz Institute of Photonic Technology (IPHT), Germany
Copyright © 2020 Baimler, Lisitsyn and Gudkov. This is an open-access article distributed under the terms of the Creative Commons Attribution License (CC BY). The use, distribution or reproduction in other forums is permitted, provided the original author(s) and the copyright owner(s) are credited and that the original publication in this journal is cited, in accordance with accepted academic practice. No use, distribution or reproduction is permitted which does not comply with these terms.
*Correspondence: Sergey V. Gudkov, c19tYWthcml5QHJhbWJsZXIucnU=