Thermodynamic and Mechanical Properties of DMPC/Cholesterol Mixed Monolayers at Physiological Conditions
- Biological Physics Laboratory, Physics Institute, Universidad Autónoma de San Luis Potosí, San Luis Potosí, Mexico
One of the main known effects of cholesterol is to rigidify the cell membrane throughout the so-called condensing effect. Although many studies have been done in mixtures of cholesterol with different membrane lipids, there are not many studies in a wide concentration range of cholesterol or at physiological conditions. In this work, we studied mixtures of DMPC/Cholesterol monolayers to determine the effect of cholesterol, from very low to physiological concentrations and two pHs. We use a Langmuir balance and Brewster angle microscopy to study their thermodynamic behavior at 37.0 ± 0.1°C at the air/solution interface. From the analysis of the (π−A) isotherms, we determined the excess area and the compressibility elastic modulus to determine the monolayers mechanical properties. Surprisingly, we found three main effects of cholesterol: The first one is a fluidization effect of the monolayer at all cholesterol concentrations. The second effect is the so-called condensing effect that appears due to the non-ideality of the mixture. The third effect is a stiffness of the monolayer as the cholesterol concentration increases. These effects are stronger in pure water, pH ≈ 6.6, than on buffer at physiological pH = 7.4. We also found that all mixtures are thermodynamically stable at all concentrations at a surface pressure of 30.1 ± 1.6 and 27.4 ± 3.2 mN/m in pure water and buffer, respectively. Furthermore, we compared this stability with a fatty acid monolayer that shows a much lower surface pressure equilibrium value that DMPC or its mixtures with cholesterol, indicating a possibly reason why double chain lipids are better than single chain lipids to made up the cell membrane.
Introduction
Cholesterol is a very important component in all membranes of mammalian cells and it is critical to human health: It is known that cholesterol is responsible for the modulation of physical properties of cell membranes, because the bulky molecular structure of cholesterol interferes with the movement of the phospholipid tails [1]. It constitutes up to 40% of the plasma membrane in some type of cells [2] and the cholesterol concentration seems to be involved in the regulation of microphase separation (lipid rafts), rigidity, membrane thickness and permeability [3–5].
One effect of adding cholesterol is to reduce the Lβ/Lα phase transition temperature, and it removes completely the transition at 50% of cholesterol concentration [6], by inducing the formation of an intermediate phase known as the liquid crystalline ordered or liquid ordered phase [5, 7]. The transition temperatures are correlated with the chain melting temperature (0°C for some glycerophospholipids and 37°C for sphingolipids). Other effects of adding cholesterol include changes in the lipid molecule cross sectional area, the thickness of the bilayer, the orientational order of the lipids and the motion of the hydrocarbon chains [1, 3, 5].
Cholesterol mixed with phospholipids can form oligomeric chemical complexes with a fundamental stoichiometry 3:2 and 6:1 phospholipids per cholesterol molecule [8, 9]. The formation of these phospholipid/cholesterol complexes produce the so-called cholesterol condensing effect [4, 7, 10, 11] where the area occupied by the molecules is decreased. As stated by the umbrella model, the lipid acyl chains and the nonpolar cholesterol part become densely packed as they share the limited space below the phospholipid head groups. At a particular concentration, the head groups cannot protect additional cholesterol molecules from contact with water and they form a separated and immiscible monohydrated cholesterol phase [12–14]. The solubility limit for cholesterol in phosphatidilcholines (PC) bilayers is known to be around 66% [7, 10, 12].
Recent monolayer studies of the interaction of phospholipids and cholesterol have shown that the molecular area of the mixture is typically smaller than the weighted molecular areas of the pure components [4, 15, 16]. It was found that cholesterol interacts preferentially with phospholipids containing fully saturated chains and this interaction decreases significantly with unsaturated chains [1, 5, 15, 17]. Cholesterol interacts more strongly with sphingolipids than with phosphatidylcholines of similar chain length [8, 11, 17, 18]. Monolayers mixtures of phosphatidilcholines and cholesterol have a higher collapse pressure (πc) than monolayers of single components, indicating that a mixed monolayer is more stable [16, 19, 20].
Model systems with only a few components have been extensively used to study the properties of biological membranes [8, 17, 19]. Giant unilamellar vesicles have been used to study mechanical properties and interactions between lipids and DNA, peptide and proteins in a simple model system composed of a single phospholipid bilayer [21, 22]. Langmuir monolayers have also been used as 2D model systems to study interactions present in biomembranes [3, 18], since the physicochemical and mechanical characterization of the monolayers can be obtained from surface pressure-area (π−A) measurements [17, 23, 24]. Furthermore, phase transitions, morphologies and textures can be obtained by combining additional characterization techniques such as neutron and X-ray scattering, polarized fluorescence, Brewster angle microscopy (BAM) or atomic force microscopy (AFM) [12, 18, 25].
Phosphatidylcholines play an important role in cell membranes since they represent more than 50% of the lipids of the plasma membrane in most eukaryotic cells [26]. The interaction of DMPC and other phosphatidylcholines of different acyl chain length and saturation degree with cholesterol has been studied at pH 6.6 and 24°C, and found that cholesterol cannot condense in the same way unsaturated lipids as it does saturated lipids, due to the kinks of the double bonds on the acyl chains [27]. In a similar study, at different temperatures from 10–30°C, it was found that acyl chain asymmetry modifies the interfacial elasticity of the lipid monolayers [28]. The condensation effect in DMPC and DPPC induced by different sterols at 23°C has been determined by mean of the analysis of the excess free energy; it was found that the mixture of phosphatidylcholines with cholesterol produced the most stable monolayers in comparison when cholesterol is replaced by ergosterol or lanosterol [29]. The effect of the subphase pH on the condensation effect in mixed monolayers of DPPC/cholesterol in a wide range of cholesterol fraction (10–90%) has been studied by Gong et al, at 25°C. They found that the monolayer is more stable at neutral pH and at 60% of cholesterol fraction [11]. Kim et al. observed that at 23°C a very low fraction of cholesterol (≈0.2%) modifies dramatically the morphology and the dynamic properties of a DPPC monolayer by reducing the surface viscosity due to the formation of 6:1 phospholipid/cholesterol complexes. This complexes decorates the boundaries of the DPPC lipid domains [9].
In this work, we study the interaction between DMPC and cholesterol from very low to physiological cholesterol mole fractions (0.01–0.40) and at physiological conditions of temperature, 37 ± 0.1°C and pH, ≈ 6.6 and 7.4. We use the Langmuir balance technique to study the model membrane monolayers, and we obtain the mechanical properties of the membranes from the isotherms. We show that cholesterol have three effects i) It fluidizes the monolayer at low surface pressures and at all concentrations studied, ii) When the pure condensed phase appears, it shows the so-called condensing effect, and iii) Upon increasing the concentration of cholesterol the monolayers stiffens.
Experimental
Materials
DMPC (1,2-Dimyristoyl-sn-glycero-3-phosphocholine, > 99%, Sigma-Aldrich, United States), Cholesterol (>99% Sigma- Aldrich, United States) and Arachidic acid (≥99% Sigma- Aldrich, United States) were used without further purification. DMPC, Cholesterol and Arachidic acid were dissolved in HPLC grade chloroform (>99%, Fermont, Mexico). Then DMPC and Cholesterol were mixed in different molar ratios (0, 0.01, 0.02, 0.03, 0.04, 0.10, 0.15, 0.20, 0.30, 0.35, 0.40, and 1.0) of cholesterol and stored at −20°C.
Methods
Langmuir-Blodgett Trough
A Langmuir-Blodgett trough (model 611, NIMA Technology LTD., Coventry, England) was used to measure the pressure-area isotherms, using a filter paper as the Wilhelmy plate for the surface pressure determination (with a precision of ±0.1 mN/m). The trough was filled with deionized water (bioresearch grade water, >18.0 MΩ-cm of resistivity, Barnstead/Thermolyne, Dubuque, Iowa, United States) and deionized water was used to prepare the pH 7.4 phosphate buffer solution. The temperature was kept at 37.0 ± 0.1°C, during the experiments in order to have human physiological temperature conditions, using a water recirculator bath (Neslab, United States). Before starting each experiment, the subphase and trough cleanliness were tested by closing the barriers and checking that the pressure sensor readings were less than 0.1 mN/m when the barriers of the Langmuir through were fully closed (and by the presence of a dark background only, observed by Brewster angle microscopy, see below). Using a 50 µl Hamilton glass microsyringe, the lipid/Cholesterol mixtures were gently deposited on the air/water interface and waited at least 30 min to allow for the evaporation of the solvent before starting each experiment. The monolayer was then compressed at 20 cm2/min. The average area per molecule was calculated by the NIMA software based upon the average molecular weight, concentration and volume of the deposited sample.
Brewster Angle Microscope
During the compression, images of the monolayer were acquired using a Brewster Angle Microscope BAM (Nanofilm EP4, Accurion GmbH, Germany) in order to see morphologies of the monolayer and phase transitions, along the obtained isotherms in the whole surface pressure range.
Mixed Monolayer Stability Study
We studied the stability of the DMPC/cholesterol mixed monolayers by slowly compressing them up to 35 mN/m and maintaining the area per molecule constant by using the area control function of the NIMA trough software. The surface pressure was recorded for approximately 300 min, in order to determine changes in surface pressure as consequence of the monolayer relaxation until its equilibrium value. The experiments were done both in ultrapure water (pH ≈ 6.6) and in a buffer subphase at pH 7.4.
Results and Discussion
Isotherms
The addition of even a very small amount of cholesterol produces a considerable shift in the take-off pressure area [12]. Considering an ideal mixture behavior between DMPC and cholesterol, each isotherm should give a take-off pressure area equal to A = XDMPC ADMPC + XChol AChol, where Ai and Xi are the molecular take-off area and the mole fraction of the “i” component, respectively. Taking a 0.01 cholesterol fraction gives A = 0.99 (142) +0.01 (40) = 141.0 Å2/molecule, which is much larger than the experimental value of 115.3 ± 0.1 Å2/molecule obtained. In fact, this is reflected at all cholesterol concentrations studied here, as it is shown in Figure 1. This difference implies that cholesterol interacts strongly with the liquid expanded (LE) DMPC phase, disrupting its formation and making the gas phase to disappear at much lower areas per molecule. Here, we are taking the take-off pressure as a reference for the “disappearing” of the gas phase, although in a mixture this is not completely correct, especially when the concentration of cholesterol becomes high. However, at the higher concentration of cholesterol in the mixture, we notice that the gas phase disappeared at about 5–8 mN/m and at lower cholesterol concentrations the gas phase disappear at even lower surface pressure; therefore we are taking the take-off pressure area as a reference for this case.
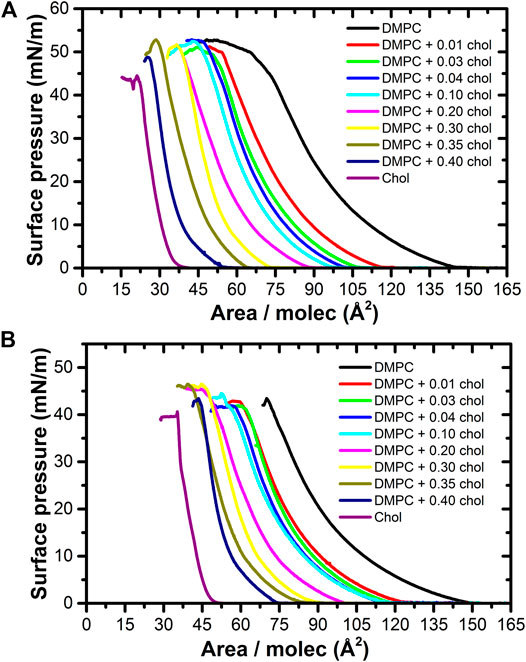
FIGURE 1. Pressure-area isotherms of the DMPC/Cholesterol mixtures at 37.0 ± 0.1°C, on (A) ultrapure water at pH ≈ 6.6 and (B) PBS Buffer at pH 7.4 subphases. As the cholesterol mole fraction increases, the take-off pressure moves to lower areas per molecule, extending the presence of the gas phase.
So, taking the take-off pressure as a reference of the condensed phase of pure DMPC, the molecules are arranged with a particular tilt azimuthal order parameter [30]. However, the bulky cholesterol molecule disrupts this order, shifting the appearance of the pure condense phase to lower areas per molecule, as denoted by a smaller take-off area of the surface pressure, as shown in Figure 2. A cholesterol molecule changes the tilt angle of the DMPC molecules around it, making them more vertical with respect to the surface, so that they occupy a smaller effective area. The LE − G coexistence region is thus extended, decreasing the take-off pressure area by more than just the difference in areas of the individual components [16, 17, 31]. Cholesterol have therefore the effect of fluidizing the monolayer, preventing the appearance of the pure condensed phase.
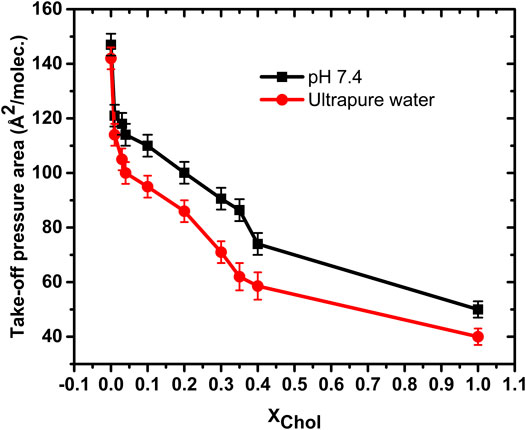
FIGURE 2. Take-off pressure area of the studied isotherms on ultrapure water at pH ≈ 6.6 and on PBS Buffer pH 7.4 subphases. In both cases, the cholesterol delays the appearance of the pure condensed phase to lower areas/molec.
Figure 3 shows representative BAM images along various isotherms. The images show the coexistence of the gaseous (G) phase (darker regions) and the more condensed (LE) phase (brighter regions) at relatively low surface pressures (Figures 3A–D). In the pure DMPC isotherm, the G phase disappears at the take-off surface pressure, as it is noticeable absent at a pressure of 5 mN/m (Figure 3E), something typical for a pure component system [18, 32, 33]. As we increase the amount of cholesterol there is a residual amount of gaseous phase at the same pressure of 5 mN/m, see Figures 3F–H [17, 34]. But at the surface pressure of 15 mN/m, the gaseous phase disappears at all mixture concentrations but in fact it disappears even a lower surface pressures, rendering a homogenous monolayer in the condensed phase (Figures 3I–L). At an even higher pressure value of 32.5 mN/m, we notice the presence of small 3D crystals which become more noticeable at higher pressures particularly close to the collapse pressure (Пc). The amount and size of the 3D crystals increase with the cholesterol concentration. It has been proposed [35] that the properties of a lipid monolayer can be correlated to those of a bilayer around a surface pressure of 32–35 mN/m.
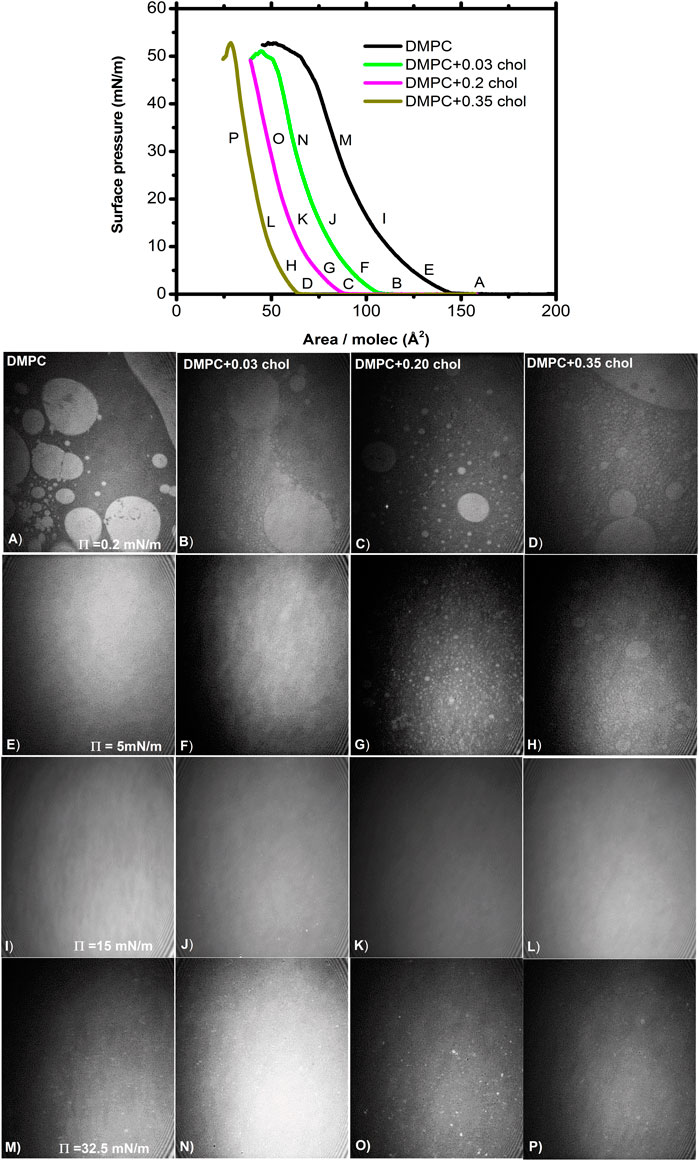
FIGURE 3. BAM images of DMPC/chol mixed monolayer at different surface pressures at 37.0°C. The images are 462 × 564 µm2. All the images in a row (column) correspond to the surface pressure (cholesterol fraction) indicated in the first image.
Excess Area Analysis
A way to estimate the miscibility and the interactions between molecules present in a two-component monolayer mixture is by the determination of the excess area [11, 16]:
where A12 is the average area per molecule of the mixture and Ai and Xi as defined above. A negative excess area indicates attractive forces between the two kind of molecules of the mixture [11, 20, 23, 31]. Figure 4 shows the excess area determined at different surface pressures in the cholesterol range studied. The excess area is negative in all cases, indicating attractive interactions in the condensed phase between DMPC and cholesterol. At a given pressure the excess area is fairly constant at all cholesterol fractions; showing a clear effect that is noticeable even at the smaller amount of cholesterol studied. The effect is more evident at lower surface pressures, possible due to there is more space between the phospholipid molecules where cholesterol can be intercalated. As the surface pressure increases, this space is reduce making the presence of cholesterol between the lipids more difficult, until it is expelled at even higher surface pressures. The strongest attractive interaction (most negative excess area) occurs in the range of 0.30–0.40 M fraction of cholesterol. It is worth noting that this range of cholesterol concentration coincides with the physiological value in most cell membranes [11, 17, 26].
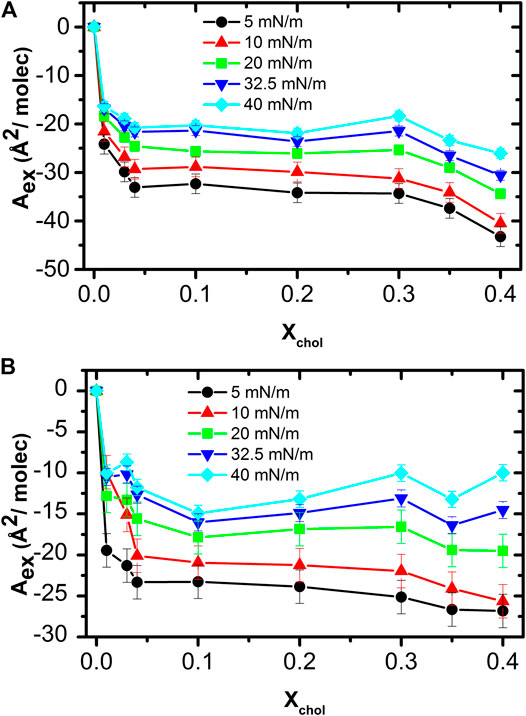
FIGURE 4. Excess area as function of cholesterol concentration at different surface pressures in ultrapure water (A) and in pH 7.4 subphase (B).
Thermodynamic Properties
Thermodynamic stability of mixed monolayers can be obtained by comparing the pure monolayer using the excess Gibbs free energy [23],
It can be noticed from Figure 5 that the excess Gibbs-free energy for all the mixtures is negative, therefore it can be concluded that the DMPC and cholesterol molecules form a stable mixed monolayer at all conditions. The lowest energy happens again at a concentration between 30–40% of cholesterol concentration and at surface pressures of 30–40 mN/m that correspond to the values present in mammalian cell membranes [2, 26, 35].
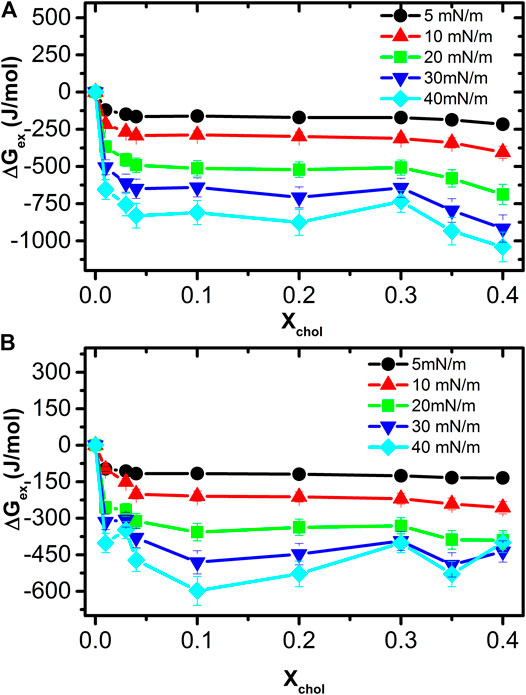
FIGURE 5. Excess Gibbs free energy for the mixed monolayer at different cholesterol concentrations and surface pressures in ultrapure water (A) and in pH 7.4subphase (B). For all the isotherms, the ΔGex is negative, indicating that the molecules mix spontaneously.
Mechanical Properties and Equilibrium Spreading Pressure
Monolayer mechanical properties can be analyzed by calculating the isothermal compressibility or rather its inverse, the area compressibility elastic modulus given by [15, 23, 24],
Figure 6 shows a plot of
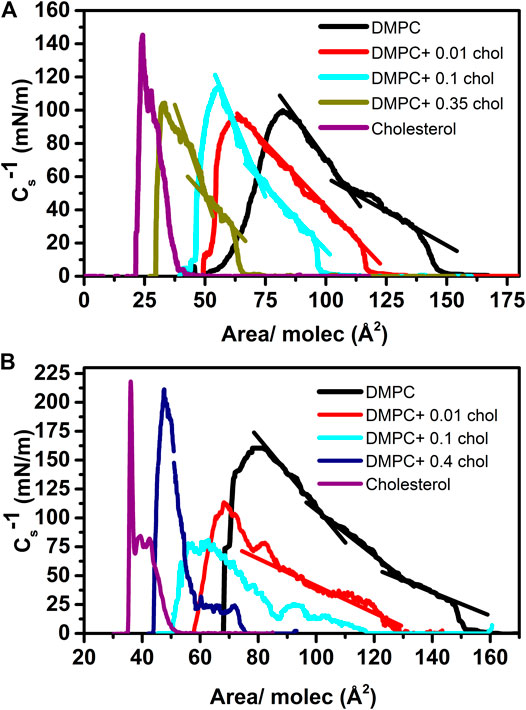
FIGURE 6. Area compressibility elastic modulus at different cholesterol fractions at 37.0 ± 0.1°C on ultrapure water subphase (A) and on pH 7.4 (B).
On the other hand, the behavior of both type isotherms, and therefore
It is worth noticing that the maximum of the
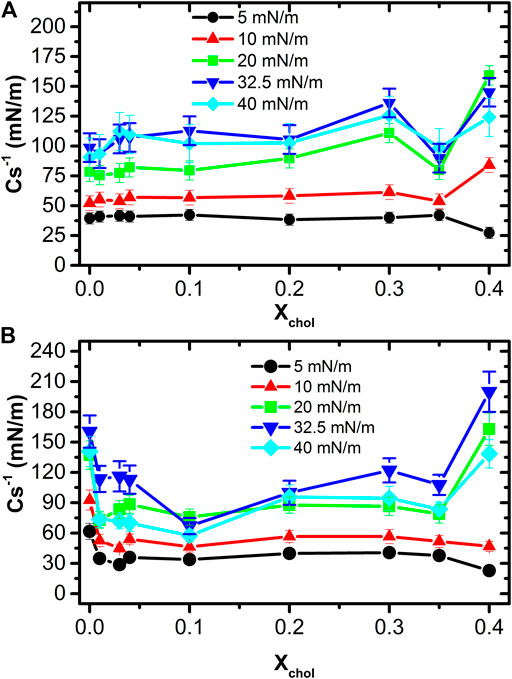
FIGURE 7. Comparison between area compressibility elastic modulus at different surface pressures on ultrapure water subphase (A) and pH 7.4 subphase (B).
Figure 8 shows a monolayer stability analysis as a function of time at pH ≈ 6.6 (Figure 8A) and physiological pH (Figure 8B). In this study, we prepare again Langmuir monolayers with different concentrations of cholesterol and compared their relaxation with that of pure DMPC. We also include for comparison, the relaxation behavior of pure arachidic acid and pure cholesterol. It has been proposed [13, 34] that the behavior of a monolayer in a surface pressure range of 32–35 mN/m is equivalent to the behavior of a bilayer in a cell membrane at 20°C. To test this hypothesis, the monolayers were slowly compressed (20 cm2/min) up to 35 mN/m and allowed to relax to its equilibrium surface pressure.
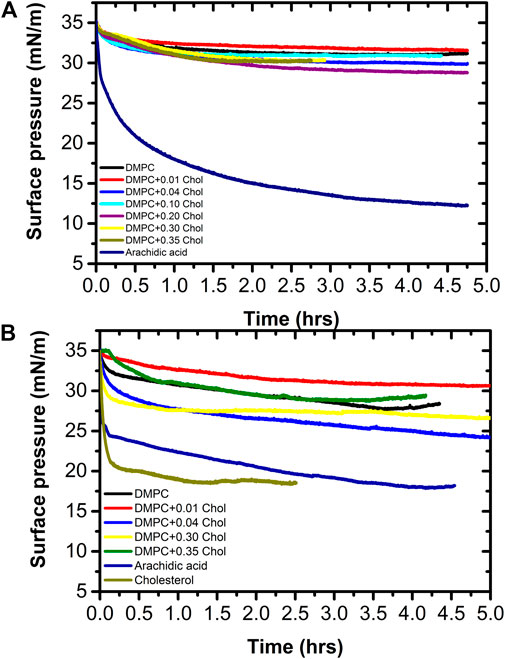
FIGURE 8. Monolayer equilibrium surface pressure comparison as a function of the cholesterol concentration at (A) pH 6.6 and (B) pH 7.4 determined at 37.0 ± 0.1°C. In addition, the equilibrium surface pressure of arachidic acid is shown at both conditions.
Our relaxation studies indicate that all monolayers have a pressure drop as a function of time. However, both DMPC and DMPC + cholesterol relax to an equilibrium surface pressure of about 30.2 ± 1.4 and 27.4 ± 3.2 mN/m in pure water (pH ≈ 6.6) and buffer at pH 7.4, respectively. It is surprising that at pH ≈ 6.6 the equilibrium surface pressure in quite similar for all the cholesterol concentration range, the surface pressure drop was between 3.8 and 6.2 mN/m, while at pH 7.4 the surface pressure drop is somewhat larger, between 4.4 and 11.5 mN/m. Therefore, the equilibrium surface pressure values at pH 6.6 are not very different for all DPPC-Cholesterol mixtures, but at pH 7.4 the equilibrium surface pressure values are not as homogeneous. The pressure drop indicates that the monolayer is slowly collapsing; that is, forming three-dimensional structures, but it is worth noting that the equilibrium surface pressure at pH 7.4 of pure DMPC and its mixture with a cholesterol mole fraction of 0.35 falls in the range of the equilibrium surface pressure at pH 6.6. In addition, we can state that at 37.0°C and physiological pH 7.4 that any of these monolayers could be equivalent to a bilayer below 27 mN/m, in terms of their equilibrium properties. Remarkably, pure cholesterol has a quick and very large surface pressure drop at physiological pH, to about 17 mN/m, as shown in Figure 8B). This indicates that the equilibrium surface pressure of the mixture is mostly due to the DMPC. Moreover, the surface pressure of arachidic acid shows two behaviors at pH 7.4; first, a rapid decay of about 11 mN/m in a short time, followed by a much slower decay of about 6 mN/m more toward the final equilibrium spreading pressure of about 18 mN/m. This is, the equilibrium spreading pressure value of arachidic acid is similar to that of pure cholesterol and almost half the initial surface pressure value. Even more, at pH 6.6, the equilibrium pressure of arachidic acid decays more slowly that at pH 7.4, but it decays even to a lower value of about 12.5 mN/m. This give us a good indication that monolayers formed by single chain lipids have a much lower equilibrium surface pressure than monolayers formed by double chain lipids, such as phospholipids. This also might indicate why nature chose double chain lipids, such as phospholipids, to be the main lipid components in cell membranes. Due to energetic considerations, single chain lipids tend to form micelles while double chain lipids tend to form vesicles that are associated with the formation of cell membranes [39]. However, even if single chain lipids could form vesicles, they could not form thermodynamically stable unilamellar vesicles but rather multiwall vesicles; for the case of arachidic acid they will be 4 to 5 bilayers thick [32].
Figure 9 shows a series of representative Brewster angle microscopy images of the equilibrium spreading pressure experiments of the monolayers. First at a surface pressure of 35 mN/m (left) and then at the equilibrium surface pressure (right) of the corresponding sample. The first row corresponds to the DMPC monolayer, at 35 mN/m where a homogeneous monolayer can be observed. While at 27 mN/m a few 3D structures can be noted over the homogeneous DMPC monolayer, this is due to the relaxation of the monolayer. In the second row, a homogeneous Cholesterol monolayer can be noted at 35 mN/m and then when the equilibrium surface pressure is reached (17 mN/m), after 2.5 hours, some 3D structures can be noted floating on a homogeneous monolayer. In the third row the DMPC+0.3 cholesterol mixed monolayer is shown. At the start of the experiment at 35 mN/m, separated 3D structures can be noted due to the condensing effect induced by the cholesterol molecules. While at the equilibrium surface pressure (27 mN/m), 3D structure domains can be noted as well, forming a foam-like structure. Finally, the fourth row shows the images corresponding to the arachidic acid monolayer. At the beginning of the surface pressure relaxation experiment (35 mN/m) some 3D structures can be observed. But at the equilibrium surface pressure (17 mN/m), a large amount of arachidic acid crystals can be noticed due to the collapse of the monolayer by the relaxation process. It is important to notice that the arachidic acid monolayer achieves stability at a surface pressure value 37 % lower than the DMPC monolayer and its mixtures with cholesterol. Therefore, the phospholipid monolayer has a stable surface pressure much higher than the single-chain fatty acid.
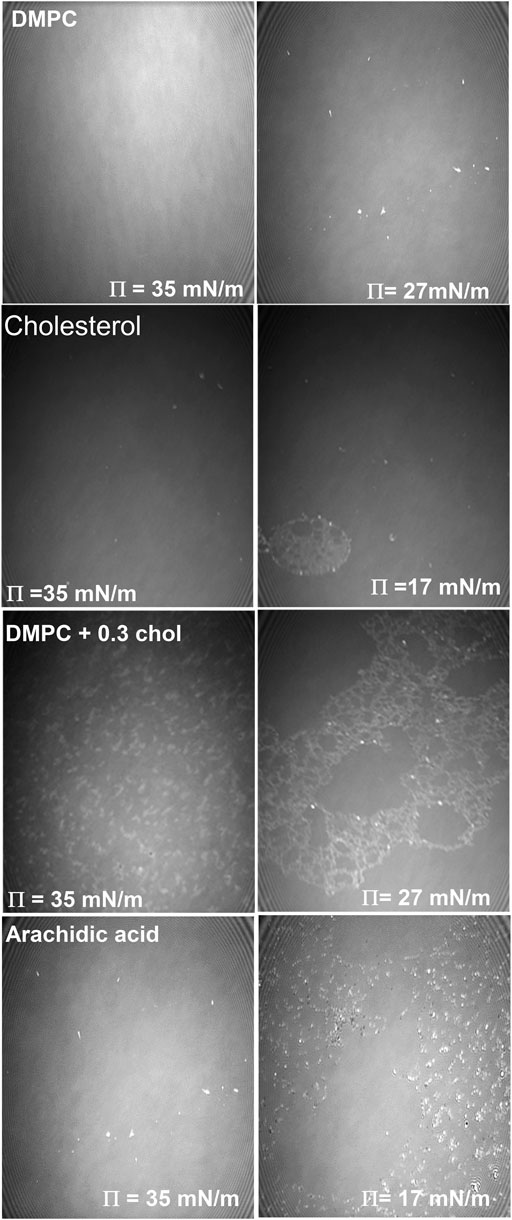
FIGURE 9. Relaxation of lipid monolayers for pure DMPC (first row) and arachidic acid (second row) monolayers at the beginning of the experiment (left column) and at the end of the experiment (right column) at pH 7.4 subphase at 37.0 ± 0.1°C. The images are 462 × 564 μm2.
Conclusion
The analysis of the thermodynamics and mechanical properties of Langmuir monolayers of DMPC/cholesterol as a function of the concentration of cholesterol and at two pH values at physiological temperature gave interesting results. For example, we found that the isotherms of DMPC/cholesterol monolayers mixtures show an increase in the monolayer fluidity at all cholesterol concentrations. Cholesterol is a bulky molecule that makes the take-off surface pressure of the monolayer appears at lower areas per molecule. This means that the presence of the gas phase remains at much lower area per molecule compared to that of the pure DMPC monolayer due to the effect of cholesterol, thus fluidizing the monolayer. However, this effect is much less pronounced as the concentration of cholesterol increases, and near physiological cholesterol concentration the monolayer is less compressible, as observed by an increased in slope of the compression modulus,
On the other hand, it is important to notice that the excess free energy is the lowest at physiological concentrations of cholesterol at both pH and surface pressure, this is, it becomes more negative not only as the concentration of cholesterol increases but also as the surface pressure increases as well, close to the equilibrium surface pressure determined at both pH. This indicates that DMPC and cholesterol mix better at higher cholesterol concentrations as well as at higher surface pressures. In general, in agreement to the excess area and Gibbs free energy analysis, this results showed the presence of attractive interactions that form thermodynamically stable monolayers in the whole cholesterol fraction range studied [17], and where more stable monolayers were observed when the mixture contained about the average physiological cholesterol fraction (≈0.35) that resulted to be energetically favored and gives more stable monolayers [11]. Furthermore, we show that monolayers formed by either pure DMPC or a mixture of DMPC with cholesterol relax to about the same equilibrium surface pressure, although the final surface pressure relaxation value is different depending on the pH. Furthermore, we also show that a monolayer formed by a single chain lipid, such as arachidic acid, is much less stable than the pure DMPC or the DMPC/cholesterol mixture; this results clearly indicates a possible reason on why nature uses double chain lipids as its mayor component in cell membranes.
Data Availability Statement
The raw data supporting the conclusions of this article will be made available by the authors, without undue reservation.
Author Contributions
AB-F, VMC-M, ERA-P, and EAV-M performed the experiments and data analysis. AB-F, EG, and JR-G wrote and discussed the paper funding acquisition. All authors discussed the data, conclusions and proof read the manuscript. EG and JR-G were responsible for the funding acquisition JR-G directed the project and experiments. All authors contributed in the revision of the manuscript.
Funding
This work was supported by CONACYT through grants FC-341, FC-157, CB-254981, CB-237439 and CB-254460. Part of the work was also supported by the UASLP through the Fondos Concurrentes program.
Conflict of Interest
The authors declare that the research was conducted in the absence of any commercial or financial relationships that could be construed as a potential conflict of interest.
References
1. McMullen TPW, Lewis RNAH, McElhaney RN. Cholesterol-phospholipid interactions, the liquid-ordered phase and lipid rafts in model and biological membranes. Curr Opin Colloid Interf Sci (2004) 8(6):459–68. doi:10.1016/j.cocis.2004.01.007
2. van Meer G. Lipid traffic in animal cells. Annu Rev Cell Biol (1989) 5(1):247–75. doi:10.1146/annurev.cb.05.110189.001335
3. Simons K, Toomre D. Lipid rafts and signal transduction. Nat Rev Mol Cell Biol (2000) 1:31–9. doi:10.1038/35036052
4. Bacia K, Schwille P, Kurzchalia T, Bacia K, Schwille P, Kurzchalia T. Sterol structure determines the separation of phases and the curvature of the liquid-ordered phase in model membranes. Proc Natl Acad Sci USA (2005) 102(9):3272–7. doi:10.1073/pnas.0408215102
5. Stottrup BL, Hernandez-Balderrama LH, Kunz JC, Nguyen AH, Sonquist BJ. Comparison of cholesterol and 25-hydroxycholesterol in phase-separated Langmuir monolayers at the air-water interface. J Phys Chem B (2014) 118(38):11231–7. doi:10.1021/jp506592k
6. McMullen TP, Lewis RN, McElhaney RN. Comparative differential scanning calorimetric and FTIR and 31P-NMR spectroscopic studies of the effects of cholesterol and androstenol on the thermotropic phase behavior and organization of phosphatidylcholine bilayers. Biophys J (1994) 66(3):741–52. doi:10.1016/S0006-3495(94)80850-1
7. Simons K, Vaz WL. Model systems, lipid rafts, and cell membranes. Annu Rev Biophys Biomol Struct (2004) 33:269–95. doi:10.1146/annurev.biophys.32.110601.141803
8. McConnell HM, Radhakrishnan A. Condensed complexes of cholesterol and phospholipids. Biochim Biophys Acta (2003) 1610:159–73. doi:10.1016/S0005-2736(03)00015-4
9. Kim K, Choi SQ, Zell ZA, Squires TM, Zasadzinski JA. Effect of cholesterol nanodomains on monolayer morphology and dynamics. Proc Natl Acad Sci USA (2013) 110:E3054–60. doi:10.1073/pnas.1303304110
10. Tierney KJ, Block DE, Longo ML. Elasticity and phase behavior of DPPC membrane modulated by cholesterol, ergosterol, and ethanol. Biophys J (2005) 89:2481–93. doi:10.1529/biophysj.104.057943
11. Gong K, Feng S-S, Go ML, Soew PH. Effects of pH on the stability and compressibility of DPPC/cholesterol monolayers at the air-water interface. Colloids Surf A: Physicochem Eng Aspects (2002) 207:113–25. doi:10.1016/S0927-7757(02)00043-2
12. Huang J, Feigenson GW. A microscopic interaction model of maximum solubility of cholesterol in lipid bilayers. Biophys J (1999) 76:2142–57. doi:10.1016/S0006-3495(99)77369-8
13. Brezesinski G, Möhwald H. Langmuir monolayers to study interactions at model membrane surfaces. Adv Colloid Interf Sci (2003) 100-102:563–84. doi:10.1016/s0001-8686(02)00071-4
14. Barrett MA, Zheng S, Toppozini LA, Alsop RJ, Dies H, Wang A, et al. Solubility of cholesterol in lipid membranes and the formation of immiscible cholesterol plaques at high cholesterol concentrations. Soft Matter (2013) 9:9342–51. doi:10.1039/C3SM50700A
15. de Meyer F, Smit B. Effect of cholesterol on the structure of a phospholipid bilayer. Proc Natl Acad Sci (2009) 106:3654–8. doi:10.1073/pnas.0809959106
16. Ohvo-Rekilä H, Ramstedt B, Leppimäki P, Slotte JP. Cholesterol interactions with phospholipids in membranes. Prog Lipid Res (2002) 41:66–97. doi:10.1016/S0163-7827(01)00020-0
17. Wydro P. The magnitude of condensation induced by cholesterol on the mixtures of sphingomyelin with phosphatidylcholines-study on ternary and quaternary systems. Colloids Surf B Biointerfaces (2011) 82:594–601. doi:10.1016/j.colsurfb.2010.10.023
18. Wydro P, Flasiński M, Broniatowski M. Does cholesterol preferentially pack in lipid domains with saturated sphingomyelin over phosphatidylcholine? A comprehensive monolayer study combined with grazing incidence X-ray diffraction and Brewster angle microscopy experiments. J Colloid Interf Sci (2013) 397:122–30. doi:10.1016/j.jcis.2013.01.060
19. Silvius JR, del Giudice D, Lafleur M. Cholesterol at different bilayer concentrations can promote or antagonize lateral segregation of phospholipids of differing acyl chain length. Biochemistry (1996) 35:15198–208. doi:10.1021/bi9615506
20. Dynarowicz-Łątka P, Hąc-Wydro K. Interactions between phosphatidylcholines and cholesterol in monolayers at the air/water interface. Colloids Surf B: Biointerfaces (2004) 37:21–5. doi:10.1016/j.colsurfb.2004.06.007
21. Montes LR, Alonso A, Goñi FM, Bagatolli LA. Giant unilamellar vesicles electroformed from native membranes and organic lipid mixtures under physiological conditions. Biophys J (2007) 93:3548–54. doi:10.1529/biophysj.107.116228
22. Menger FM, Keiper JS. Chemistry and physics of giant vesicles as biomembrane models. Curr Opin Chem Biol (1998) 2:726–32. doi:10.1016/S1367-5931(98)80110-5
23. Nichols-Smith S, Teh SY, Kuhl TL. Thermodynamic and mechanical properties of model mitochondrial membranes. Biochim Biophys Acta (2004) 1663:82–8. doi:10.1016/j.bbamem.2004.02.002
24. Wydro P. Sphingomyelin/phosphatidylcholine/cholesterol monolayers--analysis of the interactions in model membranes and Brewster Angle Microscopy experiments. Colloids Surf B Biointerfaces (2012) 93:174–9. doi:10.1016/j.colsurfb.2011.12.035
25. Qiu X, Ruiz-Garcia J, Stine KJ, Knobler CM, Selinger JV. Direct observation of domain structure in condensed monolayer phases. Phys Rev Lett (1991) 67:703–6. doi:10.1103/PhysRevLett.67.703
26. Van Meer G, Voelker DR, Feigenson GW. Membrane lipids: where they are and how they behave. Nat Rev Mol Cell Biol (2008) 9:112–24. doi:10.1038/nrm2330
27. Smaby JM, Momsen MM, Brockman HL, Brown RE. Phosphatidylcholine acyl unsaturation modulates the decrease in interfacial elasticity induced by cholesterol. Biophys J (1997) 73:1492–505. doi:10.1016/S0006-3495(97)78181-5
28. Ali S, Smaby JM, Momsen MM, Brockman HL, Brown RE. Acyl chain-length asymmetry alters the interfacial elastic interactions of phosphatidylcholines. Biophys J (1998) 74:338–48. doi:10.1016/S0006-3495(98)77791-4
29. Sabatini K, Mattila JP, Kinnunen PK. Interfacial behavior of cholesterol, ergosterol, and lanosterol in mixtures with DPPC and DMPC. Biophys J (2008) 95:2340–55. doi:10.1529/biophysj.108.132076
30. Kaganer VM, Peterson IR, Kenn RM, Shih MC, Durbin M, Dutta P. Tilted phases of fatty acid monolayers. J Chem Phys (1995) 102:9412–22. doi:10.1063/1.468809
31. Dynarowicz-Łątka P, Kita K. Molecular interaction in mixed monolayers at the air/water interface. Adv Colloid Interf Sci (1999) 79:1–17. doi:10.1016/S0001-8686(98)00064-5
32. Valdes-Covarrubias MA, Cadena-Nava RD, Vásquez-Martínez E, Valdez-Pérez D, Ruiz-García J. Crystallite structure formation at the collapse pressure of fatty acid Langmuir films. J Phys Condens Matter (2004) 16:S2097–107. doi:10.1088/0953-8984/16/22/008
33. Kubo I, Adachi S, Maeda H, Seki A. Phosphatidylcholine monolayers observed with Brewster angle microscopy and π-A isotherms. Thin Solid Films (2001) 393:80–5. doi:10.1016/S0040-6090(01)01101-4
34. Subramaniam S, McConnell HM. Critical mixing in monolayer mixtures of phospholipid and cholesterol. J Phys Chem (1987) 91:1715–8. doi:10.1021/j100291a010
35. Marsh D. Lateral pressure in membranes. Biochim Biophys Acta (1996) 1286:183–223. doi:10.1016/s0304-4157(96)00009-3
36. Ege C, Ratajczak MK, Majewski J, Kjaer K, Lee KY. Evidence for lipid/cholesterol ordering in model lipid membranes. Biophys J (2006) 91:L01–3. doi:10.1529/biophysj.106.085134
37. Cadena-Nava RD, Martin-Mirones JM, Vázquez-Martínez EA, Roca JA, Ruiz-Garcia J. Direct observations of phase changes in Langmuir films of cholesterol. Rev Mexi Fís (2006) 52:32–40.
38. Castillo-Santaella D, Maldonado-Valderrama J, Faraudo J, Martín-Molina A. Specific ion effects in cholesterol monolayers. Materials (2016) 9:340–54. doi:10.3390/ma9050340
Keywords: cholesterol, DMPC, model membranes, brewster angle microscopy, Langmuir monolayers, isotherms, mechanical properties
Citation: Bañuelos-Frias A, Castañeda-Montiel VM, Alvizo-Paez ER, Vazquez-Martinez EA, Gomez E and Ruiz-Garcia J (2021) Thermodynamic and Mechanical Properties of DMPC/Cholesterol Mixed Monolayers at Physiological Conditions. Front. Phys. 9:636149. doi: 10.3389/fphy.2021.636149
Received: 01 December 2020; Accepted: 05 February 2021;
Published: 17 March 2021.
Edited by:
Enrique Hernandez-Lemus, Instituto Nacional de Medicina Genómica (INMEGEN), MexicoReviewed by:
Luciano Caseli, Federal University of São Paulo, BrazilAmir Darío Maldonado, Universidad Tecnológica del Sur de Sonora, Mexico
Copyright © 2021 Bañuelos-Frias, Castañeda-Montiel, Alvizo-Paez, Vazquez-Martinez, Gomez and Ruiz-Garcia. This is an open-access article distributed under the terms of the Creative Commons Attribution License (CC BY). The use, distribution or reproduction in other forums is permitted, provided the original author(s) and the copyright owner(s) are credited and that the original publication in this journal is cited, in accordance with accepted academic practice. No use, distribution or reproduction is permitted which does not comply with these terms.
*Correspondence: Eduardo Gomez, egomez@mail.ifisica.uaslp.mx; Jaime Ruiz-Garcia, jaime@mail.ifisica.uaslp.mx