Photodynamic Therapy Using Cerenkov and Radioluminescence Light
- 1Experimental Imaging Centre, San Raffaele Scientific Institute, Milan, Italy
- 2Department of Computer Science, University of Verona, Verona, Italy
In this short review the potential use of Cerenkov radiation and radioluminescence as internal sources for Photodynamic therapy (PDT) is discussed. PDT has been developed over the course of more than 100 years and is based on the induced photo conversion of a drug called photosensitizer (PS) that triggers the production of cytotoxic reactive oxygen species (ROS) leading to the killing of the cells. In order to overcome the problem of light penetration in the tissues, different solutions were proposed in the past. The use of radioisotopes like: 18F, 64Cu, 90Y, 177Lu as internal light sources increase the light fluence at the PS compared to an external source, resulting in a larger cytotoxic effect.
1 Introduction
Photodynamic therapy (PDT) has been developed over the course of more than 100 years as will described in more details in section 2 of this review. The basic idea behind PDT is rather simple and is based on the induced photo conversion of a drug called photosensitizer (PS) that triggers the production of cytotoxic reactive oxygen species (ROS), leading to the killing of the cells as will be described in details in section 3. The clinical use of PDT is mainly focused on treating different types of cancers; however other disease like herpes acne can be treated [1], showing a wide range of possible applications. The first PS approved for the clinical use to treat cancer was an optimized hematoporphyrin derivative (HPD) known with the commercial name of Photofrin®, described in details by [2]. Since the introduction of HPD in the seventies there has been significant research focused on the development of second and third generation PS in order to increase the yield of cytotoxic ROS and introduce targeting strategies such as antibody conjuration or nanoparticles (NPs) [3]. A detailed description of the different PS is well beyond the scope of this review and more details can be found for example in [4, 5]. The main difficulties related to the use of PDT for clinical applications are due to the intrinsic processes (absorption and scattering) of the light in penetrating tissues, the low concentration of the PS at the tumor site and the lack of oxygen in hypoxic tumor regions. In order to overcome the problem of light penetration in the tissues, different solutions have been proposed in the past. In this review we will focus on the recent use of Cerenkov radiation (CR) (section 4) and radioluminescence (section 5) as internal light sources to excite the PS molecules and, thus, to trigger ROS production inside the tumor region as shown in Figure 1. We would like to point out that we did not make any attempt in writing an exhaustive description on PDT and its clinical applications, for this please refer also to these reviews [6, 7].
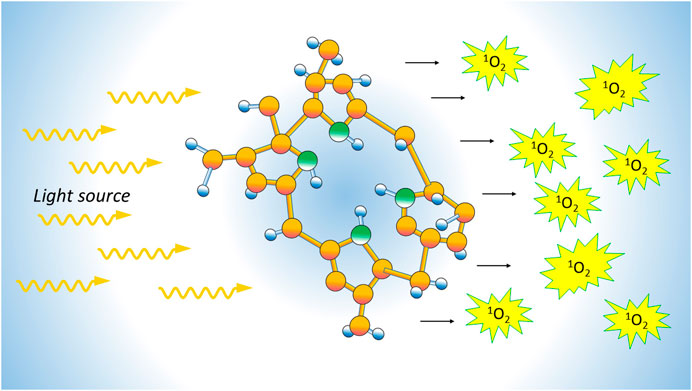
FIGURE 1. The figure describes the basic steps of PDT that is based on the induced photo conversion of a PS that trigger the production of cytotoxic reactive oxygen species leading to the killing of tumor cells.
2 Historical Overview of PDT
The use of light to treat diseases can be dated back to antiquity. For example, the ancient Greek physicians suggested the use of sunlight to treat diseases [8]. The use of light was then abandoned for many centuries until the beginning of the 20th century. The modern era of PDT begin in the early 1900s with the important contribution of the Danish physician, Niels Finsen. In his work he showed that it was possible to treat pustules or cutaneous tuberculosis using red or UV light. In 1903 Finsen was awarded the Nobel Prize for his discovery [9]. Almost at the same time, the possibility of using light in combination with a drug to kill cells was suggested by Oscar Raab, a medical student at the time. During a thunderstorm, Raab observed that the combination of acridine and light was lethal to Infusoria. Starting from this findings his supervisor Herman von Tappeiner showed the important role of oxygen in the reaction, and in 1904, he introduced the term photodynamic action [10]. The use of the term dynamic did not convinced von Tappeiner himself and there has been some attempts (with no success) to modify it into photochemotherapy. A very important advancement in PDT was that hematoporphyrin and its derivative (HPD) was a powerful PS with a strong affinity with neoplastic tissues [11]. Based on this result a clinical trial was performed at Roswell Park Cancer Institute in 1978 [2, 12]. The properties of HPD as PS were improved by purifying the compound to extract the more active monomers, currently known with the commercial name of Photofrin® [2]. Photofrin absorbs light at 400 nm (Soret band) and 630 nm (Q-band) [13]. Contemporary PS are designed with an absorption range of 650–800 nm, longer wavelength range reduces the absorption and scattering properties of the tissues. Wavelengths longer than 800 nm can not be used because in this case the photon energy is too small to induce the generation of singlet oxygen [6]. As will be discussed in sections 4 and 5 the problem of tissue absorption and scattering can be reduced using CR or radioluminescence as internal light sources.
3 PDT Mechanisms
The starting step of PDT is the absorption of an optical photon by the PS leading to the molecule transition from the singlet state
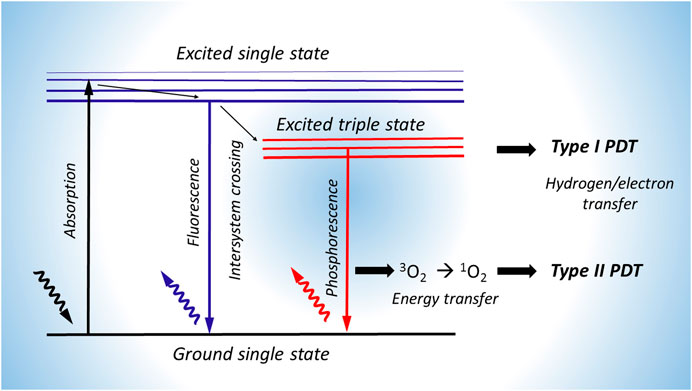
FIGURE 2. Scheme of the different photosensitization processes after the absorption of a photon by the PS. Two different types of reactions can take place, in Type I process an electron of the PS is transfer by reacting with a nearby substrate forming ROS. The type II reaction involves an energy transferred from the PS triplet state
4 PDT Using Cerenkov Light Sources
4.1 Cerenkov Radiation Production
Before addressing the use of Cerenkov light as a source for PDT it is useful to describe how CR radiation is produced considering its peculiar nature [17–19]. CR is produced when a charged particle travels through a dielectric medium inducing a local polarization, more precisely the atoms behave like elementary dipoles as shown in Figure 3. If the particle speed v exceeds the speed of light c in a medium with a refracting index n, or more precisely when
where
where
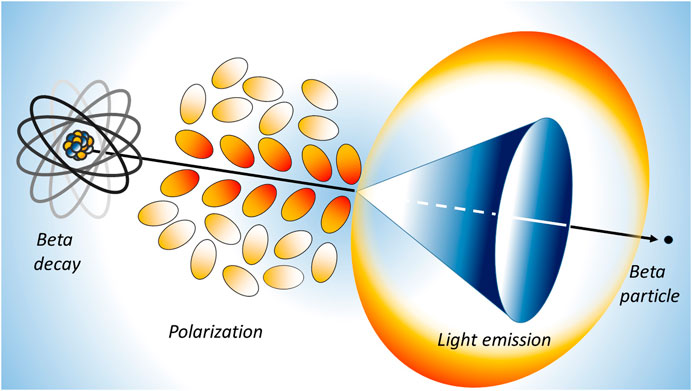
FIGURE 3. Schematic representation of the Cerenkov light emission process. A beta particle, emitted by a radionuclide, travels in a medium faster than the light speed in the medium itself. The fast de-polarization of the molecules produces a cone of light known as Cerenkov radiation.
4.2 PDT Using Isotopes Emitting Cerenkov Radiation
As mentioned in section 1, one of the main problems of PDT is the delivery of light to the PS due to the intrinsic absorption and scattering properties of the tissues. One solution is to use CR created within the tumor as the light source. The main advantage of using Cerenkov sources is the possibility of having in the same location (e.g., tumor site) both the PS and the light source for PDT. The local light emission feature of Cerenkov sources has been exploited also in endoscopic imaging as shown by [21]. Another important feature of CR is its spectrum, which is weighted to the UV and blue regions where some PS absorb strongly. In a preclinical proof-of-principle study with a breast cancer animal model expressing luciferase [22] investigated the possible role of 18FDG, a widely used positron emission tomography (PET) tracer, as a internal light source to photoactivate caged luciferin. No PDT was performed in this case. In particular they used luciferin 1-(4,5-dimethoxy-2-nitrophenyl) ethyl ester (DMNP-luciferin) to demonstrate in vitro and in vitro the effect of the CR emitted by 18FDG as a possible source to activate luciferin. In vitro results obtained using breast adenocarcinoma cells (MDA-MB-231-luc-D3H1) showed that the CR emitted by 18FDG induced similar photoactivation to UV light at 365 nm. As described in [23] transferrin-coated TiO2 nanoparticles in combination with PET radionuclides like 18F and 64Cu can be used to perform PDT in vitro and in vivo. Cell viability was reduced significantly in vitro with respect to control groups when combining radionuclides with the PS. Similar results were observed in vivo with an aggressive HT1080 subcutaneous mouse tumor model. After 12 days from the start of the treatment, the tumor volume dropped to almost zero while the tumor volumes of the untreated groups were in the 400–600 mm3 range. Histological analysis confirmed extensive tumor necrotic regions [24]. developed an analytical model with the goal of combining the physics of Cerenkov light production, scattering, and the mechanisms of PDT reaction when using TiO2 NPs as PS. Their model has been validated using the experimental data found in [23, 25]. The main result of [24] was the establishment of a mechanistic description based on the Frank–Tamm theory of CR production (seeEq. 2) and the photocatalytic generation of free radicals. It has been found that the ROS production depends on the isotope activity (MBq), the NPs concetration (mol/L) and diameter (in this case 25 nm). The model agrees well with the experimental data found in the literature and possibly can be applied to other types of radionuclides, PS, or can be used to optimize the NPs size. Recently [26] proposed the combination of Doxorubicin (DOX), a chemotherapeutic agent that has been used to treat different types of haematological and solid cancers, with the CR emitted by 18F. Unfortunately DOX has severe side effects like liver damage or cardiotoxicity, however its alternative use as PS was proposed by [27] after a strong reduction of the injected dose. The interesting feature of DOX is its excitation band centered at 480 nm that matches well the spectrum of the CR as described in section 4.1. The cytotoxic effect of sutch combination was evaluated in vitro using a breast cancer cell line (T47D). In was shown that cell viability was reduced up to 85% by increasing 18F activity (=140 μCi) in combination with DOX. In order to increase the Cerenkov light yield [13] developed mesoporous silica nanoparticles (HMSNs) able to encapsulate a second-generation PS like chlorin e6 (Ce6) [28] and 89Zr. The Chlorin e6 (Ce6) shows interesting PS proprieties as high efficiency and low dark toxicity, and, at the same time, the use of 89Zr allows a greater Cerenkov light yield production having an higher beta endpoint energy (909 keV) and also a longer half life (78.4 h) compared to 18F and 64Cu. In their work [13] found with in vitro experiments a strong dose-dependent cell viability reduction depending on the concentration of Ce6 and 89Zr. In particular cell viability was reduced to 10% when using an 89Zr activity of 1 MBq and a concentration of HMSN-Ce6 equal to 40 μM. An in vivo study was performed using 4T1 tumors in Balb/c mice with a size of =200 mm3 and showed a strong tumor control for 14 days after a single dose of [89Zr]HMSN-Ce6 (15 MBq, 50 μl). The damage to the tumor tissue was also confirmed by H&E stained tumor slices taken 7 days after treatment. In [29] a novel hybrid tetramodal imaging nanotheranostics tool based on 89Zr for photothermal and PDT was described. More precisely they developed a nanoconstruct by assembling copper sulfide (CuS) nanoparticles on the surface of 89Zr–labeled hollow mesoporous silica nanoshells filled with porphyrin molecules. In order to investigate in vivo the tetramodal properties of [89Zr]CSNC-PEG10k its biodistribution was imaged using: PET, fluorescence, Cerenkov luminescence and CR energy transfer imaging. It has been found for a subcutaneous 4T1 murine breast tumor model using these imaging techniques, that [89Zr]CSNC-PEG10k showed a specific uptake even 15 days after intravenous injection. Recently [30] investigated the use of 131I-bovine serum albumin loaded into chlorin-lipid nanovesicle cavity. The main goal was to combine the anti-tumor effect of 131I radiotherapy with PDT induced by Cerenkov light emitted by 131I. Their results showed a reduced lung tumor growth in mice. In particular the survival curve was significantly prolonged and, at the same time, histopathology on different organs found no strong side effects. The use of 131I as both radiotherapy and PDT Cerenkov light source was also investigated by [31] using a Zn based nanoplatform. Both in vitro and in vivo experiments were carried out using 4T1 cell line. Cellular viability was measured and it has been found equal to 29.2% using an activity of 40 μCi. The same cell line has been used to establish a tumor model with a subcutaneous injection of 5 × 106 cells. After 14 days from the treatment the average tumor volume of the treated mice was about 180 mm3 while the volume of the untreated group was 850 mm3. Ex vivo analysis using hematoxylin and eosin staining was performed and showed more tumor cells with shrinked nuclei and, at the same time no particular damage to other organs like: heart, liver, spleen and kidney. Using the same 4T1 cell line [25] investigated the use of 68Ga (endpoint energy 1.899 MeV) as a more intense CR source to activate a dextran-modified TiO2 PS. A comparison between 18F-FDG, and 68Ga-labeled bovine serum albumin was performed, and it has been found a stronger inhibitor of the growth of 4T1 cells and stronger DNA damage to tumor cells when using 68Ga to excite the PS. Another interesting isotope for PDT is 90Y considering its half-life of 64.1 h and the endpoint energy of 2.278 MeV leading to an higher number of emitted Cerenkov photons as described in section 4.1. The use of 90Y as PDT source was investigated in vitro by [32] with the C6 glioma cell line in combination with the widely used aminolevulinic acid (ALA) or the porphyrin-based PS TTPS2a. It has been found that the cell viability was reduced respectively down to 60 and 45% for the combination of 90Y with ALA or TTPS2a using an activity equal to 60 μCi in the well.
5 PDT Using Radioluminescence Light
In section 4 we focused our attention on the use of Cerenkov light emitted by beta plus or beta minus radioisotopes as a possible internal source for PDT. Another possible source that has been investigated is radioluminescence light that is normally produced by the interaction of ionizing radiations with a scintillator material. However it has been recently shown that radioluminescence light can also be produced in non scintillating material like: Plexiglas, glass [33] small animals [34] or ex vivo tissue [35]. An alternative solution to solve the problem of light tissue penetration is the use of an external X-rays source in combination with scintillating nanoparticles (ScNPs). This type of NPs behave as a local X-ray converter, more precisely the ScNPs are able to absorb X-ray and then produce scintillation light that can excite the PS and induce ROS production. An example of the use of Fluoride-based material for X–ray excited optical imaging can be found in [36] were NaGdF4:Eu3+ NPs were used to convert X–ray into optical photons within the 600–700 nm range. By using this NPs it was possibile to perform X-ray luminescence optical tomography. Another interesting approach to perform X–ray excited optical imaging is the use of quantum dots (QDs) to convert X-ray into visible photons. A recent example of this method was described in [37] where core-type CdTe has been used to convert X-rays with energies between 20 and 120 keV. Phantom experiments were carried out by filling a syringe with 0.1 ml aqueous suspensions of 715 nm-emitting CdTe QDs, with 0, 0.005, 0.016, 0.13, and 0.46 mg of CdTe. The syringe was then irradiated at: 55, 70, and 100 kVp resulting in intense photons emission that could be imaged in 1 s using a sensitive EM-CCD detector. Mouse imaging was performed by irradiating at 55 kV p and 500 mA a mouse subcutaneously injected with 0.46 mg of CdTe in back of the neck. The corresponding optical emission image shows a good match with the corresponding anatomical region. One of the first attempts to use scintillating light for PDT in vivo was described in [38]. In this paper the authors combined porphyrins with scintillating rare Earth NPs exited by X-rays in order to obtain X-rays excited optical luminescence (XEOL). The interesting feature of rare Earth NPs is the emission peak at around 400 nm that matches well with the strong absorption peak (Soret band) of porphyrins. In [39] it has been reported the synthesis of LaF3:Tb3+-meso-tetra(4-carboxyphenyl)porphine (MTCP) NPs conjugates with the addition of folic acid to improve the targeting to folate receptors. It has been found that this type of NPs can potentially be used to trigger PDT to treat deep cancer. In [40] a micellar system based on amphiphilic lanthanide chelates that includes the Hyp PS inside the core has been developed. This micellar system, once exited by X-ray, was able to produce singlet oxygen production through PDT. It is also important to note that the inclusion of lanthanides in the structure allow the detection of the micellar systems using optical or magnetic resonance imaging. More recently [41] investigated the use of titania NP in particular dumbbell-like Au–TiO2 NPs (DATs). This type of NP can be activated by X-rays resulting in the production of secondary photons or electrons, leading to ROS production.
6 Discussion and Conclusion
In this short review we discussed the potential use of Cerenkov and radioluminescence light as internal sources for PDT. The main advantages of using these excitation sources are reduced light scattering and absorption by the tissue and, at the same time, a lower cytotoxic effect on normal tissue since most of the light is emitted inside the target (e.g., tumor) region. The use of Cerenkov and radioluminescence light for PDT significantly expand the more common applications as: preclinical [39, 42, 43], clinical [44] and ex vivo [45] diagnostic tool. An important aspect that should be further exploited regarding the use of Cerenkov and radioluminescence light sources, is the possibility of enhancing the cytotoxic effect of PDT. This can be achieved by inducing further damage using the so called radiotherapy cross fire effect obtained with beta emitters like: 90Y, 177Lu or 32P [46]. Another interesting research line should focus on a better integration between the PS with different radioisotopes in order to optimize photon absorption, at the same time reducing the distances and, thus, possible photons loss. PDT based on radioluminescence light should be further exploited by developing more efficient ScNPs, for example. In conclusion the use of Cerenkov and radioluminescence light sources is a promising clinical approach for PDT considering also the possibility of using several radiopharmaceuticals already approved for clinical use. Secondly, and more importantly, the combination of radiotherapy and PDT can have an important local synergistic effect for cancer treatment, while possibly reducing side effects to normal tissues.
Author Contributions
AS performed the literature review and wrote the manuscript. FB contributed to manuscript revision and figures. All authors have read and agreed to the published version of the article.
Conflict of Interest
The authors declare that the research was conducted in the absence of any commercial or financial relationships that could be construed as a potential conflict of interest.
References
1. Oniszczuk A, Wojtunik-Kulesza KA, Oniszczuk T, Kasprzak K. The potential of photodynamic therapy (PDT)-Experimental investigations and clinical use. Biomed Pharmacother (2016) 83:912–29. doi:10.1016/j.biopha.2016.07.058
2. Dougherty TJ. A brief history of clinical photodynamic therapy development at roswell park cancer institute. J Clin Laser Med Surg (1996) 14:219–21. doi:10.1089/clm.1996.14.219
3. Baskaran R, Lee J, Yang SG. Clinical development of photodynamic agents and therapeutic applications. Biomater Res (2018) 22:25. doi:10.1186/s40824-018-0140-z
4. Chen C, Wang J, Li X, Liu X, Han X. Recent advances in developing photosensitizers for photodynamic cancer therapy. Comb Chem High Throughput Screen (2017) 20:414–22. doi:10.2174/1386207320666170113123132
5. Zhang J, Jiang C, Figueiró Longo JP, Azevedo RB, Zhang H, Muehlmann LA. An updated overview on the development of new photosensitizers for anticancer photodynamic therapy. Acta Pharmaceutica Sinica B (2018a) 8:137–46. doi:10.1016/j.apsb.2017.09.003
6. Agostinis P, Berg K, Cengel KA, Foster TH, Girotti AW, Gollnick SO, et al. Photodynamic therapy of cancer: an update. CA: A Cancer J Clinicians 61 (2011) 250–81. doi:10.3322/caac.20114
7. Hahn K, Zhang Y, Mu C, Xu Q, Jing X, Wang D, et al. Versatile nanoplatforms with enhanced photodynamic therapy: designs and applications. Theranostics (2020) 10:7287–318. doi:10.7150/thno.46288
8. Dang MD, Hill JS. A history of photodynamic therapy. ANZ J Surg (1991) 61:340–8. doi:10.1111/j.1445-2197.1991.tb00230.x
9. Ackroyd R, Kelty C, Brown N, Reed M. The history of photodetection and photodynamic therapy. Photochem Photobiol (2001) 74:656–69. doi:10.1562/0031-8655(2001)074<0656:thopap>2.0.co;2
10. Moan J, Peng Q. An outline of the hundred-year history of PDT. Anticancer Res (2003) 23:3591–600.
11. Lipson RL, BALDES EJ, OLSEN AM. The use of a derivative of hematoporhyrin in tumor detection. J Natl Cancer Inst (1961) 26:1–11.
12. Dougherty TJ, Kaufman JE, Goldfarb A, Weishaupt KR, Boyle D, Mittleman A. Photoradiation therapy for the treatment of malignant tumors. Cancer Res (1978) 38:2628–35.
13. Kamkaew A, Cheng L, Goel S, Valdovinos HF, Barnhart TE, Liu Z, et al. Cerenkov radiation induced photodynamic therapy using chlorin e6-loaded hollow mesoporous silica nanoparticles. ACS Appl Mater Inter (2016) 8:26630–7. doi:10.1021/acsami.6b10255
14. Cai SZF, Yang G, Lim WQ, Verma A, Chen H, Thanabalu T, et al. Catalase-integrated hyaluronic acid as nanocarriers for enhanced photodynamic therapy in solid tumor. ACS Nano (2019) 13:4742–51. doi:10.1021/acsnano.9b01087
15. Zhao Y, Wang F, Liu C, Wang Z, Kang L, Huang Y, et al. Nanozyme decorated metal-organic frameworks for enhanced photodynamic therapy. ACS Nano (2018b) 12:651–61. doi:10.1021/acsnano.7b07746
16. Dong T, Shen X, Li L, Guan Z, Gao N, Yuan P, et al. Gold nanorods as dual photo-sensitizing and imaging agents for two-photon photodynamic therapy. Nanoscale (2012) 4:7712–9. doi:10.1039/C2NR32196C
17. Yao JV. Cerenkov radiation and its applications. Br J Appl Phys (1955) 6:227–32. doi:10.1088/0508-3443/6/7/301
18. Spinelli AE, Boschi F. Novel biomedical applications of Cerenkov radiation and radioluminescence imaging. Physica Med (2015) 31:120–9. doi:10.1016/j.ejmp.2014.12.003
19. Boschi F, Spinelli AE. Nanoparticles for cerenkov and radioluminescent light enhancement for imaging and radiotherapy. Nanomaterials (Basel) (2020) 10. doi:10.3390/nano10091771
20. Mitchell GS, Gill RK, Boucher DL, Li C, Cherry SR. In vivo Cerenkov luminescence imaging: a new tool for molecular imaging. Phil Trans R Soc A (2011) 369:4605–19. doi:10.1098/rsta.2011.0271
21. Zhang Z, Cai M, Bao C, Hu Z, Tian J. Endoscopic Cerenkov luminescence imaging and image-guided tumor resection on hepatocellular carcinoma-bearing mouse models. Nanomedicine: Nanotechnology, Biol Med (2019) 17:62–70. doi:10.1016/j.nano.2018.12.017
22. Ran C, Zhang Z, Hooker J, Moore A. In vivo photoactivation without “light”: use of cherenkov radiation to overcome the penetration limit of light. Mol Imaging Biol (2012) 14:156–62. doi:10.1007/s11307-011-0489-z
23. Kotagiri N, Sudlow GP, Akers WJ, Achilefu S. Breaking the depth dependency of phototherapy with Cerenkov radiation and low-radiance-responsive nanophotosensitizers. Nat Nanotech (2015) 10:370–9. doi:10.1038/nnano.2015.17
24. Kavadiya S, Biswas P. Design of cerenkov radiation-assisted photoactivation of TiO2 nanoparticles and reactive oxygen species generation for cancer treatment. J Nucl Med (2019) 60:702–9. doi:10.2967/jnumed.118.215608
25. Duan D, Liu H, Xu Y, Han Y, Xu M, Zhang Z, et al. Activating tio2 nanoparticles: gallium-68 serves as a high-yield photon emitter for cerenkov-induced photodynamic therapy. ACS Appl Mater Inter (2018) 10:5278–86. doi:10.1021/acsami.7b17902
26. Liu HA, Aranda-Lara L, Morales-Ávila E, Torres-García E, Camacho-López MÁ, Sánchez-Holguín M, et al. In vitro irradiation of doxorubicin with 18f-fdg cerenkov radiation and its potential application as a theragnostic system. J Photochem Photobiol B: Biol (2020) 210:111961. doi:10.1016/j.jphotobiol.2020.111961
27. Luna-Gutiérrez KW, Gao J-P, Sharma T. Photodynamic enhancement of doxorubicin cytotoxicity. Cancer Chemother Pharmacol (1994) 35:17–20. doi:10.1007/bf00686279
28. Martynenko IV, Kuznetsova VA, Orlova O, Kanaev PA, Maslov VG, Loudon A, et al. Chlorin e6-ZnSe/ZnS quantum dots based system as reagent for photodynamic therapy. Nanotechnology (2015) 26:055102. doi:10.1088/0957-4484/26/5/055102
29. Goel S, Ferreira CA, Chen F, Ellison PA, Siamof CM, Barnhart TE, et al. Activatable hybrid nanotheranostics for tetramodal imaging and synergistic photothermal/photodynamic therapy. Adv Mater (2018) 30:1704367. doi:10.1002/adma.201704367
30. Cai P, Yang W, He Z, Jia H, Wang H, Zhao W, et al. A chlorin-lipid nanovesicle nucleus drug for amplified therapeutic effects of lung cancer by internal radiotherapy combined with the cerenkov radiation-induced photodynamic therapy. Biomater Sci (2020) 8:4841–51. doi:10.1039/d0bm00778a
31. Gao Q, Liu N, Hou Z, Shi J, Su X, Sun X. Radioiodinated persistent luminescence nanoplatform for radiation‐induced photodynamic therapy and radiotherapy. Adv Healthc Mater. (2020) 10:2000802. doi:10.1002/adhm.202000802
32. Hartl BA, Hirschberg H, Marcu L, Cherry SR. Activating photodynamic therapy in vitro with cerenkov radiation generated from yttrium-90. J Environ Pathol Toxicol Oncol (2016) 35:185–92. doi:10.1615/jenvironpatholtoxicoloncol.2016016903
33. Pagliazzi M, Boschi F, Spinelli AE. Imaging of luminescence induced by beta and gamma emitters in conventional non-scintillating materials. RSC Adv (2014) 4:13687–92. doi:10.1039/C3RA47102K
34. Spinelli AE, Lo Meo S, Calandrino R, Sbarbati A, Boschi F. Optical imaging of Tc-99m-based tracers: in vitro and in vivo results. J Biomed Opt (2011) 16:116023. doi:10.1117/1.3653963
35. Ackerman NL, Boschi F, Spinelli AE. Monte Carlo simulations support non-Cerenkov radioluminescence production in tissue. J Biomed Opt (2017) 22:1–11. doi:10.1117/1.jbo.22.8.086002
36. Sudheendra L, Das GK, Li C, Stark D, Cena J, Cherry S, et al. NaGdF4:Eu3+ nanoparticles for enhanced X-ray excited optical imaging. Chem Mater (2014) 26:1881–8. doi:10.1021/cm404044n
37. Kennedy SG, Butler MN, Adeyemi SS, Kalber T, Patrick PS, Zaw Thin M, et al. Imaging of X-ray-excited emissions from quantum dots and biological tissue in whole mouse. Sci Rep (2019) 9:19223. doi:10.1038/s41598-019-55769-5
38. Chen W, Zhang J. Using nanoparticles to enable simultaneous radiation and photodynamic therapies for cancer treatment. J Nanosci Nanotech (2006) 6:1159–66. doi:10.1166/jnn.2006.327
39. Liu Y, Chen W, Wang S, Joly AG. Investigation of water-soluble x-ray luminescence nanoparticles for photodynamic activation. Appl Phys Lett (2008) 92:043901. doi:10.1063/1.2835701
40. Kascakova S, Giuliani A, Lacerda S, Pallier A, Mercere P, Toth E, et al. X-ray-induced radiophotodynamic therapy (RPDT) using lanthanide micelles: beyond depth limitations. Nano Research (2015) 8:2373–9. doi:10.1007/s12274-015-0747-5
41. Cheng K, Sano M, Jenkins CH, Zhang G, Vernekohl D, Zhao W, et al. Synergistically enhancing the therapeutic effect of radiation therapy with radiation activatable and reactive oxygen species-releasing nanostructures. ACS Nano (2018) 12:4946–58. doi:10.1021/acsnano.8b02038
42. Wei F, Calderan L, D’Ambrosio D, Marengo M, Fenzi A, Calandrino R, et al. In vivo 18F-FDG tumour uptake measurements in small animals using Cerenkov radiation. Eur J Nucl Med Mol Imaging (2011) 38:120–7. doi:10.1007/s00259-010-1630-y
43. Sbarbati W, Qin W, Hu Z, Suo Y, Zhao R, Ma X, et al. Comparison of Cerenkov luminescence imaging (CLI) and gamma camera imaging for visualization of let-7 expression in lung adenocarcinoma A549 Cells. Nucl Med Biol (2012) 39:948–53. doi:10.1016/j.nucmedbio.2012.05.004
44. Ma AE, Ferdeghini M, Cavedon C, Zivelonghi E, Calandrino R, Fenzi A, et al. First human cerenkography. J Biomed Opt (2013) 18 020502. doi:10.1117/1.jbo.18.2.020502
45. Sbarbati AE, Schiariti MP, Grana CM, Ferrari M, Cremonesi M, Boschi F. Cerenkov and radioluminescence imaging of brain tumor specimens during neurosurgery. J Biomed Opt (2016) 21:050502. doi:10.1117/1.jbo.21.5.050502
Keywords: photodynamic therapy, Cerenkov radiation, radioluminescence, nano particles, photosensitizer
Citation: Spinelli AE and Boschi F (2021) Photodynamic Therapy Using Cerenkov and Radioluminescence Light. Front. Phys. 9:637120. doi: 10.3389/fphy.2021.637120
Received: 02 December 2020; Accepted: 01 February 2021;
Published: 29 March 2021.
Edited by:
Zhen Cheng, Stanford University, United StatesReviewed by:
Kevin Diamond, McMaster University, CanadaZhenhua Hu, University of Chinese Academy of Sciences, China
Copyright © 2021 Spinelli and Boschi. This is an open-access article distributed under the terms of the Creative Commons Attribution License (CC BY). The use, distribution or reproduction in other forums is permitted, provided the original author(s) and the copyright owner(s) are credited and that the original publication in this journal is cited, in accordance with accepted academic practice. No use, distribution or reproduction is permitted which does not comply with these terms.
*Correspondence: Antonello E. Spinelli, spinelli.antonello@hsr.it