Effect of F-Actin Organization in Lamellipodium on Viscoelasticity and Migration of Huh-7 Cells Under pH Microenvironments Using AM-FM Atomic Force Microscopy
- 1School of Physics, Sun Yat-sen University, Guangzhou, China
- 2State Key Laboratory of Optoelectronic Materials and Technologies, Sun Yat-sen University, Guangzhou, China
- 3Centre for Physical Mechanics and Biophysics, School of Physics, Sun Yat-sen University, Guangzhou, China
Cytoskeleton is responsible for fundamental cellular processes and functions. The filamentous actin (F-actin) is a key constituent of the cytoskeleton system which is intrinsically viscoelastic and greatly determines the mechanical properties of cells. The organization and polymerization of F-actin are relevant to the viscoelasticity distribution and the migration of living cells responding to pH microenvironments. Recently, progression in various diseases such as cancers have been found that cellular migration is related to the alterations in the viscoelasticity of lamellipodium. However, the correlation among F-actin organization, viscoelastic properties and cellular migration of living cancer cells under different pH microenvironments are still poorly understood. Conventional experimental methods of optical microscopy and atomic force microscopy (AFM) can neither break the trade-off between resolution and rate in cytoskeleton imaging, nor achieve the structural characterization and the mechanical measurement simultaneously. Although multifrequency AFM with amplitude modulation-frequency modulation (AM–FM) enables us to probe both the surface topography and the viscoelasticity distribution of cells, it is difficult to image the cytoskeletal filaments with the diameter down to the scale of tens of nanometers. Here, we have improved the AM-FM AFM by employing the high damping of cell culture medium to increase the signal-to-noise ratio and achieve a stable imaging of F-actin with the resolution down to 50 nm under in situ microenvironment. The approach that can successfully visualize the structures of cytoskeletal filaments and measure the distribution of mechanical properties simultaneously enable us to understand the relationship between the organization of F-actin and the viscoelasticity of living Huh-7 cancer cells under different pH values. Our experimental results have demonstrated that, unlike the randomly distributed F-actin and the homogeneous viscoelasticity at the normal pH level of 7.4, the living Huh-7 cancer cells with the reduced pH level of 6.5 show highly oriented and organized F-actin along the lamellipodium direction associated with the significant gradient increase both in elasticity and viscosity, which are confirmed by immunofluorescence confocal microscopy. The F-actin organization and the gradient viscoelasticity of lamellipodium provide structural and mechanical understanding on the adhesion and migration of living cancer cells that undergo metastasis and malignant transformation.
Introduction
Living cells, as the smallest unit in biological systems, plays a pivotal role in human life and disease processes [1]. Changes in the viscoelastic properties of cells are relevant to the fundamental cellular physiological behaviors and functions, such as cell migration [2], adhesion [3], differentiation [4] and absorption [5]. Cytoskeleton that is an essential structural component of cells, is intrinsically viscoelastic and responsible for the cell viscoelasticity [6], controlling the cellular development and maintenance [7]. In the cytoskeleton system, the filamentous actin (F-actin) (Figure 1) is one of the most major constituent building up many higher order structures in cells (e.g., stress fibers, lamellipodia, and filopodia) [8]. The arrangement and distribution of F-actin greatly dominates the mechanical properties of cells including viscoelasticity [9–13]. For example, the actin cortex is a thin layer that lies beneath the plasma membrane, maintaining and regulating cell topography [10]. Oberleithner et al. found that the depolymerization of cortical actin induced by altering the local microenvironment reduces the elasticity of cells, thus modulating the deformation of the plasma membrane [11]. The stress fibers are arranged in parallel by F-actin, connecting the cytoskeleton to the extracellular matrix via focal adhesions [12]. Wang et al. reported that viscosity of F-actin can significantly increase stability of the cell adhesion [13]. The cellular protrusions including lamellipodium and filopodium located at the edge of cells is involved with the cell migration [14, 15]. Lamellipodia are sheet-like structures, from which filopodia usually grow out [16]. Lamellipodial branched actin network not only generates a pushing force by actin polymerization but also provides crucial mechanical support for cell migration through the extracellular matrix or adjacent cells [17, 18]. Laurent et al. revealed that cells migrates toward the more rigidity side of the lamellipodia [19]. Moreover, lamellipodia-based cell migration plays a crucial role in cancer metastasis [20]. The malignant degree of cancer cells is related to their migration speed, that is, the metastasis and invasiveness of cancer cells [21]. In particular, in the complex microenvironment of tumor tissues [22, 23], the cancer cells will change the structure and topography of lamellipodia at an acid pH level, and then accelerate their metastasis [24, 25]. However, the organization of the F-actin in lamellipodia under different pH microenvironments associated with the underlying relationship with the viscoelasticity and migration of living cancer cells remain unclear and is in urgent need to be fully interpreted. The nanomechanical mechanism revealing the effects of F-actin in lamellipodia on the cell viscoelasticity and migration represents a meaningful approach to understand the physical nature in the malignant metastasis of cancer cell response to the change of pH microenvironments.
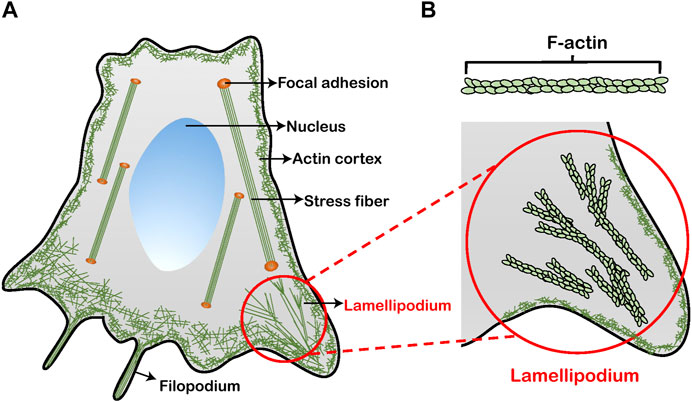
FIGURE 1. Schematics of a cell with F-actin architectures. (A) Four primary types of F-actin structures in the cytoskeleton: actin cortex, stress fiber, filopodium, and lamellipodium. (B) A magnified view of the lamellipodium. The formation of lamellipodium is facilitated by the organization of F-actin.
To understand the correlation among F-actin organization, viscoelastic properties and cellular migration of living cancer cells under different pH microenvironments, it is the key issue to simultaneously obtain the high-resolution in situ images of the cytoskeleton topography and viscoelastic properties of cells. For the soft matter of cells that are heterogeneous with intrinsically viscoelastic cytoskeletons and undergo large deformation [26, 27], the structural organization and mechanical properties are highly coupled [28]. A wide variety of experimental work has been performed in order to assess the relationship between cell mechanics and microstructures [29, 30], focusing on the changes in either cytoskeletal structure [29] or elasticity [30]. However, these experiments have been limited by the characterization methods of cells and cytoskeletons. Although the fluorescence imaging techniques have the advantage in the fast in situ identification of subcellular structures, it is difficult for the optical resolution to be smaller than the diffraction limit around hundreds of nanometers and capture the details of cytoskeletal structures [31]. The transmission electron microscopy is usually adopted to observe the cells and cytoskeletons [32]. Nevertheless, the sample preparation process is complicated with fixation, dehydration, infiltration and staining, which will cause the loss of cell activity [33]. Furthermore, the imaging of transmission electron microscopy is slow and commonly conducted under vacuum condition, which make it unable for the fast in situ identification of cells and cytoskeletons under different microenvironments. For studying cellular mechanics, a number of experimental methodologies have been developed, including micropipette aspiration [34], traction force microscope [35], optical tweezers [36], and magnetic twisting cytometry [37]. Atomic force microscopy (AFM) is one of the most major and reliable methods for probing the mechanical properties of cells [38, 39]. Conventional AFM imaging based on contact mode force curve measurement has been introduced to measure the cellular viscoelasticity [40, 41]. And yet the poor resolution and long acquisition time prevent it from fast high-resolution characterization of subcellular microstructures and mechanical properties. It is a key challenge to not only break the trade-off between resolution and rate in cytoskeleton imaging, but also achieve the structural characterization and the mechanical measurement simultaneously.
In this work, we use amplitude modulation–frequency modulation (AM–FM) AFM to characterize the topography and viscoelasticity of living Huh-7 cancer cells at the same time. We have improved the AM-FM AFM by using the high damping of cell culture medium, instead of original damping materials that cannot be used in liquid, to dissipate unnecessary vibration that deviates from the standard resonance of the AFM cantilever. The efficient stabilization of the cantilever oscillation enables us to increase the signal-to-noise ratio, achieving a fast imaging and mapping simultaneously of F-actin structures, cellular topography and viscoelasticity with the ultrahigh resolution down to 50 nm under in situ pH microenvironment. Moreover, the capability of AM–FM AFM to capture the F-actin microstructures are validated by immunofluorescence confocal microscopy. By using AM–FM AFM, we study the effect of pH level on the organization of F-actin in lamellipodium and the change in the topography and viscoelasticity of living Huh-7 cancer cells. The experimental results demonstrate that the F-actin in lamellipodium under normal culture medium (pH 7.4) is short, thin and dispersed, associated with the homogeneous distribution of cell viscoelasticity. On the contrary, in acid culture medium (pH 6.5), the F-actin cytoskeletons polymerize and are woven into a long and thick bundle-like structure directed to the protruding direction of lamellipodium. Both the elasticity and viscosity show a significant gradient increase along the protruding direction, which can facilitate the adhesion and migration of living cancer cells. These results have significant implications for understanding the significance of F-actin structures and viscoelasticity in changing cell topography and behaviors, and opening a new paradigm of nanomechanical mechanisms for the metastasis of cancer cells under pH microenvironments.
Materials and Methods
Cells Culture and Treatment
The cells used in this study are the human hepatoma cell lines Huh-7 cells, obtained from the Type Culture Collection of the Chinese Academy of Sciences, Shanghai, China. The cells were cultured in Dulbecco’s Modified Eagle Medium (Gibco, Life Technologies, China) supplemented with 10% fetal bovine serum (Gibco, Life Technologies, Australia) and 1% penicillin-streptomycin (Gibco, Life Technologies, United States), at 37°C and 5% CO2 in humid conditions. The pH level of normal culture medium is set as 7.4. One day prior to the AFM experiments, the cells were seeded onto 25 mm × 25 mm glass slides. After the cells had enough time to adhere to the substrate, the existing medium was replaced with the fresh medium to remove dead and loosely attached cells every 2 h. The fresh medium was prepared in the normal or acid condition to mimic microenvironments at different pH levels. HCl (0.5 mol/L) was added precisely to the normal culture medium for the acid culture medium (pH 6.5) by using a pH meter measurement to verify the targeted pH value of 6.5. Phosphate buffer saline (PBS) solution was used to rinse the substrate every time before adding and changing the culture medium. The cells were cultured with either pH microenvironment for at least 6 h in CO2 incubator for subsequent characterization and measurement in AFM experiments. To maintain the pH level in the cell cultivation, the new acid culture medium of pH 6.5 was replaced every 2 h. By pH monitoring, we found that the acid culture medium of pH 6.5 in the CO2 incubator can be kept well within 2 h. The time of AM-FM AFM measurement was limited to a maximum of 2 h. During the AM-FM AFM measurement, the pH monitoring was employed constantly and the acid culture medium of pH 6.5 was added when needed.
Atomic Force Microscopy
AFM experiments were performed with an Asylum Research MFP-3D Infinity AFM system. The AM-FM bimodal imaging mode was performed by using BL-AC40TS cantilever (Olympus, Japan) with 0.11 nN/nm nominal force constant (k1). The spring constant for the first eigenmode k1 of the cantilever was obtained by force constant calibration. In AM-FM method that employs the tapping mode, the cantilever is excited near the first and second resonant frequencies simultaneously. To ensure the physiological conditions of cells, the cantilever was completely immersed in the liquid of culture medium during the experiments. The cantilever tuning was conducted in liquid by which the first cantilever eigenmode was excited to the resonant frequency
During the scanning, the first order amplitude
where
The loss tangent is equivalent to the ratio of the dissipated energy to stored energy:
which allows us to calculate the loss modulus for viscous property. Correlated topography and viscoelastic images were generated with a resolution of 256 × 256 pixels. And the scan speed was set at 0.5 line/s. As the modulus of the AFM tip used defers greatly from that of the glass substrate, the mechanical signal from the background part of glass substrate is meaningless and not considered, and is shown as the gray background in Figures 4, 5. The same AFM tip and imaging configuration were used for all samples to maintain consistency between measurements of cell viscoelasticity. Repetitions of experiments with more living Huh-7 cancer cells under different pH states are performed to verify the robustness of results and mechanisms in our work.
Confocal Fluorescence Microscopy
Confocal fluorescence microscopy was used to observe the F-actin microstructures and validate the AFM imaging. The cells for the imaging by confocal fluorescence microscopy were seeded onto 20 mm cell slides and incubated with the same culture conditions as for AFM measurements. The cells were washed with PBS, fixed with 4% paraformaldehyde in PBS and kept at 4°C for around 15 min. Afterwards the cells were permeabilized with 0.5% Triton X-100 in PBS at room temperature for 15 min and rinsed with PBS. The nucleus of cells was stained with DAPI (Solarbio, China) for 15 min in dark. The F-actin cytoskeleton of cells was stained in dark using Alexa-Fluor 488 Phalloidin (Solarbio, China) for 30 min. Finally, the slides were mounted with the DAPI mounting medium (Solarbio, China). The stained cells were examined by using a confocal microscope setup (Leica SP8X, Germany) to visualize the F-actin microstructures. The fluorescent F-actin cytoskeleton images were acquired with a 63x/1.40 oil immersion objective lens (Leica Microsystems, Germany).
Results and Discussion
Improved Amplitude Modulation-Frequency Modulation Atomic Force Microscopy for Mapping Topography and Viscoelasticity of Living Cells
To simultaneously obtain the images of topography and viscoelastic properties of living cells under physiological conditions, we have improved the original AM-FM AFM assembly. In the AM-FM method, two driving signals with different frequencies (black and red signals) are superposed to excite two independent vertical oscillation modes of the cantilever (Figure 2A). The first resonance operates in normal tapping mode (AM mode). The amplitude
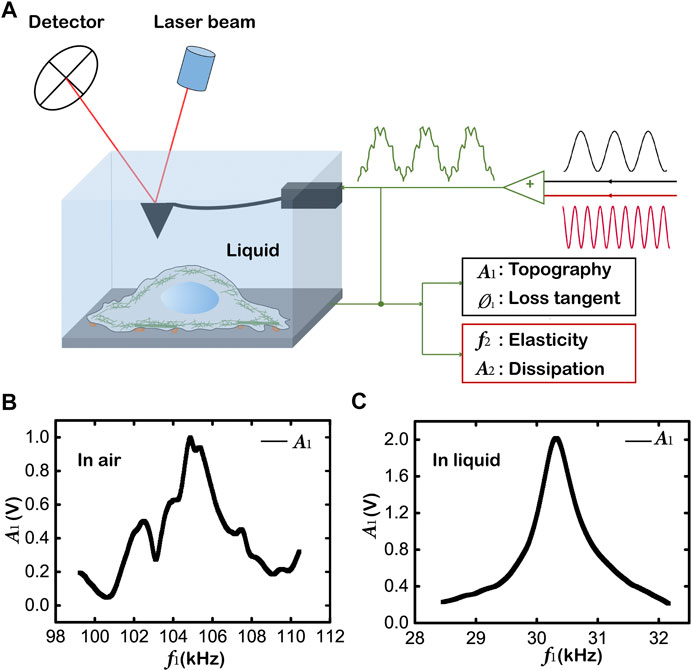
FIGURE 2. Mapping topography and viscoelasticity of living cells by AM-FM AFM. (A) Schematics of the AM-FM AFM showing the experimental setup and the working principle. The AFM tip and the whole cantilever are immersed in the liquid of culture medium for in situ measurement of living cells in physiological condition. Two separate excitation signals are combined to excite the cantilever resonances. The cantilever deflection when probing the sample is analyzed to evaluate the offsets of the two resonances for the mapping of topography and viscoelasticity. (B) The first resonance signal of the cantilever without high damping in air. (C) The first resonance signal of the cantilever in liquid.
In addition, the ratio
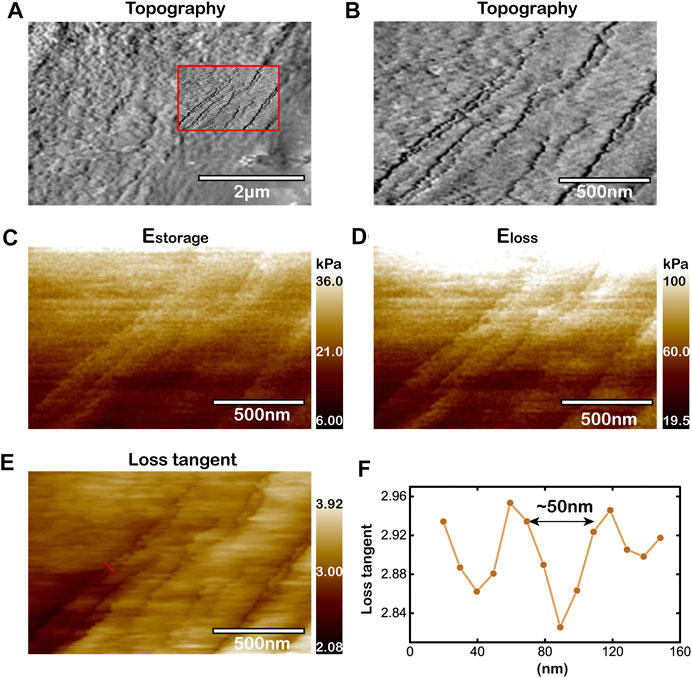
FIGURE 3. Capability to image cytoskeleton microstructures of living cells by improved AM-FM AFM. (A,B) Topography of microregion near the periphery of living cancer cell. The view of (B) magnifies the rectangular region within the red box in (A). Topography of the red box in (A). (C–E) Mapping of
Figure 3 shows the topography and viscoelasticity of living cells. It is important to note that the string microstructure observed in the images of viscoelasticity (Figures 3C–E) are highly correlated to the features observed in the topography image (Figures 3A,B). The widths of the strings are tens of nanometers. The storage moduli
Topography and Viscoelasticity of Cytoskeletons in Lamellipodium at pH 7.4
Based on the in situ AM-FM AFM method we improved for simultaneous mapping topography and viscoelasticity of living cells, it is feasible to study the relationship between the organization of cytoskeleton microstructures and the distribution of viscoelastic properties under different pH microenvironments. The microenvironment of cancer cells in tumor tissue is constantly acidified [23], with a lower extracellular pH value (∼6.5) than the basic value (∼7.4) in normal tissue [22]. The acid microenvironment enables the progression of cancer cells by promoting proliferation, the evasion of apoptosis, metabolic adaptation, migration and invasion that are involved with the malignant transformation and metastasis [46]. During this stage, the F-actin and the lamellipodium are the two crucial media linking the cellular behaviors and the mechanical properties. It has been reported that the acid extracellular pH induces the reorganization of F-actin cytoskeleton and facilitates the formation of lamellipodial protrusion [25], which can modulate the morphology and rigidity against the forces needed for cell migration [47]. It would be a key challenge and a great significance to study the correlation between the organization of F-actin structures and the viscoelasticity distribution in lamellipodium of living cancer cells under normal (pH 7.4) and acid (pH 6.5) microenvironments for revealing the nanomechanical mechanism of the cellular migration in metastasis.
In the normal microenvironment (pH 7.4), we use the improved AM-FM AFM method to characterize the topography and the viscoelasticity of living Huh-7 cells as shown in Figure 4. Figure 4A shows an overall topography of the cell edge, with the region of lamellipodium in the red box measured by larger contact force and scanning density for higher resolution in topography (Figure 4B), storage modulus
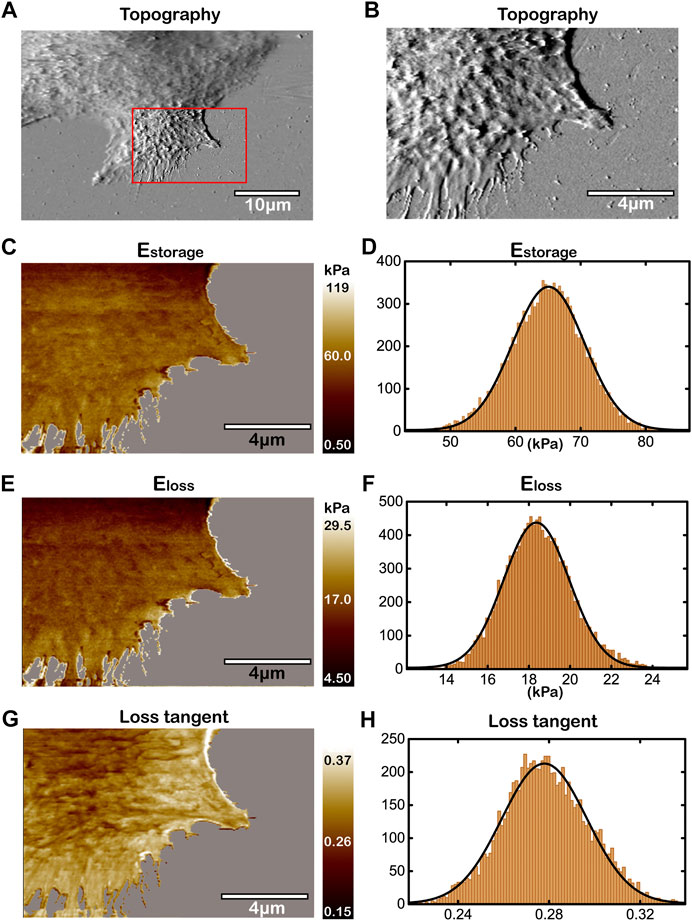
FIGURE 4. Topography and the viscoelasticity of Huh-7 cells at pH 7.4. (A) Topography of cell edge. (B) High-resolution topography of lamellipodium region within the red box in (A). (C,E,G) Mapping of
Topography and Viscoelasticity of Cytoskeletons in Lamellipodium at pH 6.5
To study the effect of acid microenvironment on living cancer cells, we change pH value of the culture medium to pH 6.5 [22], and measure the topography and the viscoelasticity of Huh-7 cells by the improved AM-FM AFM as shown in Figure 5 and Supplementary Figure S3. Unlike the homogeneous dispersion of cytoskeletons in lamellipodium at pH 7.4, the topography of Figures 5A,B show highly oriented and organized patterns of cytoskeletal structures at pH 6.5. The cytoskeletons are polymerized to long filaments and woven into thick bundle-like structures directed to the protruding direction of lamellipodium. The viscoelasticity, including storage modulus, loss modulus and loss tangent, of the same lamellipodium region in Huh-7 cells are measured and analyzed in Figures 5C–H in which the polymerized filaments and bundles are visible. The strings that have higher storage modulus, higher loss modulus and lower loss tangent than the intracellular background indicate the cytoskeletal filaments in Figures 5C,E,G, respectively. Interestingly, along with the convergence of cytoskeletal filaments towards the protruded lamellipodium, the storage modulus and loss modulus increase while the loss tangent decreases. The gradient of viscoelasticity in the lamellipodium region with the relationship to the cellular migration will be discussed in details in the latter section.
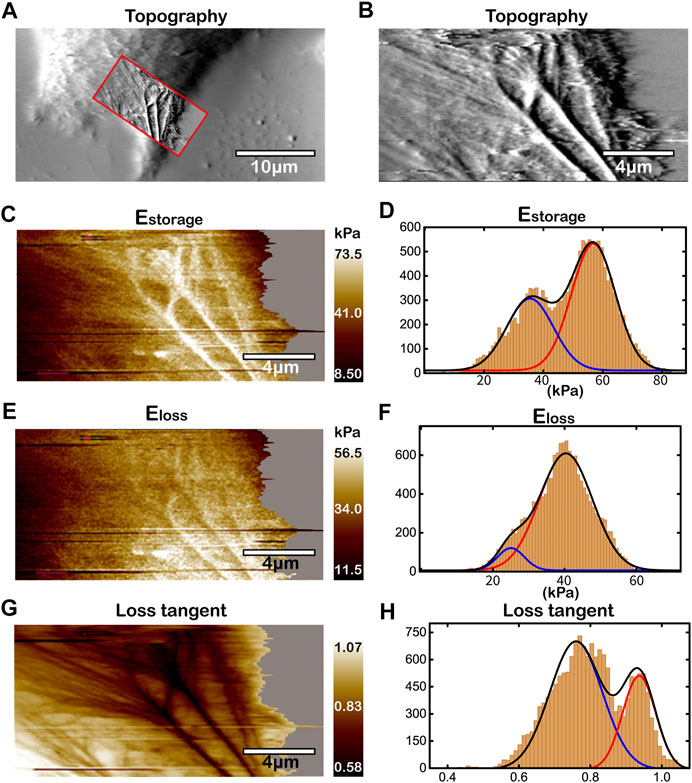
FIGURE 5. Topography and the viscoelasticity of Huh-7 cells at pH 6.5. (A) Topography of cell edge. (B) High-resolution topography of lamellipodium region within the red box in (A). (C,E,G) Mapping of
The histogram analyses of storage modulus, loss modulus and loss tangent were also performed as shown in Figures 5D,F,H, respectively. Unlike monomodal Gaussian peaks at pH 7.4, all of the storage modulus, loss modulus and loss tangent show bimodal distributions at pH 6.5, corresponding to the heterogeneous organization of cytoskeletons. In Figure 5D for elasticity, the lower peak of storage modulus with the mean value of ∼35 kPa represents the thin and unwoven cytoskeletal filaments, while the higher peak (∼60 kPa) the thick cytoskeletal filaments that have been bundled together. These results of elasticity distribution are consistent with the previous studies that the increased density of actin filaments can lead to the stiffening of cells [28, 29, 49]. The bundled actin filaments are bound by crosslinking proteins and molecular motor, such as fascin and myosin-X, providing strong viscosity [50, 51], which results in an elevated loss modulus as the higher peak (∼40 kPa) in Figure 5F. Our work visualizes the organization of cytoskeletal filaments and reveals the structural origins of the viscoelasticity distribution in the lamellipodium region of living cancer cells under acid microenvironment. Repetitions of experiments for more living Huh-7 cancer cells at pH 6.5 (Supplementary Figure S3) show similar cytoskeleton organization and cell viscoelasticity, which verify the robustness of our results. We also performed the experiments at the pH level within the range from 6.5 to 7.4, and found that the more cells with F-actin polymerization and viscoelasticity gradient can be seen at the lower pH state.
Validation of F-Actin Cytoskeletons by Immunofluorescence Confocal Microscopy
To identify the type of cytoskeletal structures shown by the improved AM-FM AFM as in Figures 4, 5, the immunofluorescence confocal microscopy was adopted and the fluorescent phalloidin that can selectively bind to F-actin was used to image the distribution of F-actin in the living Huh-7 cells (Figure 6) [52]. The Huh-7 cells were cultured in the two different pH environments (pH 7.4 and pH 6.5) as in AFM experiments and stained with Alexa-Fluor 488 Phalloidin and DAPI. The imaging of confocal microscopy shows that the F-actin filaments dyed by green are short, thin and randomly dispersed within the whole Huh-7 cell at pH 7.4 (Figure 6A). Although becoming slightly thicker near the periphery, the F-actin filaments are loosely arranged with the same homogeneous feature observed in AFM experiments (Figure 6B). On the contrary, at pH 6.5, the F-actin filaments that are much thicker and longer form highly oriented bundle-like structures (Figure 6C) and converge toward the front of lamellipodium (Figure 6D), which is in good agreement with the AFM imaging as well. The consistency of intercellular structures confirms that the cytoskeletal filaments visualized by the improved AM-FM AFM are the F-actin.
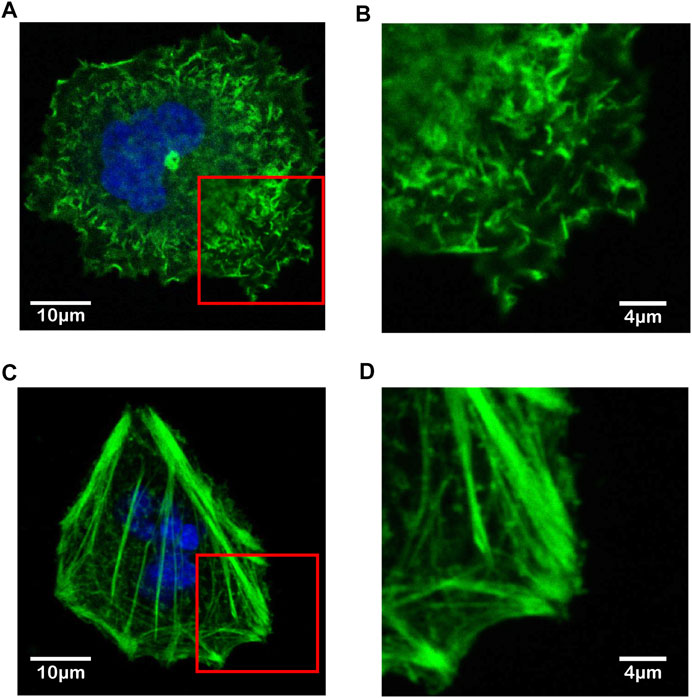
FIGURE 6. Immunofluorescence confocal microscopy images of F-actin filaments in living Huh-7 cells under different pH microenvironments. (A,B) F-actin in Huh-7 cell at pH 7.4. (C,D) F-actin in Huh-7 cell at pH 6.5. The F-actin filaments are highlighted by green and the cell nuclei by blue. The view of (B) and (D) magnifies the rectangular region near the periphery of cell within the red box in (A) and (C), respectively.
Viscoelasticity Gradient Facilitates Cell Adhesion and Migration
In previous sections, by using the improved AM-FM AFM, we have successfully characterized the viscoelasticity distribution of the lamellipodium regions in living Huh-7 cells at pH 7.4 and 6.5 (Figures 4, 5). To compare them quantitatively, we rescale the mapping of storage modulus (Figure 7A) and loss modulus (Figure 7B) to the same color range for studying the effect of viscoelasticity distribution on cell adhesion and migration. Figure 7A shows that along the direction of lamellipodium protrusion (as the arrows), the storage modulus of cell elasticity increases from ∼50 kPa to over 60 kPa at the acid microenvironment and maintains at a plateau (∼60 kPa) at the normal microenvironment. For cell viscosity following the same arrows, the loss modulus increases from 35 to over 50 kPa at pH 6.5, while staying at a low level of ∼10 kPa at pH 7.4 (Figure 7B). Combining the AFM images of cytoskeletons, we can conclude that the acid microenvironment may result in the organization of F-actin filaments and the gradient of both elasticity and viscosity in living cancer cells.
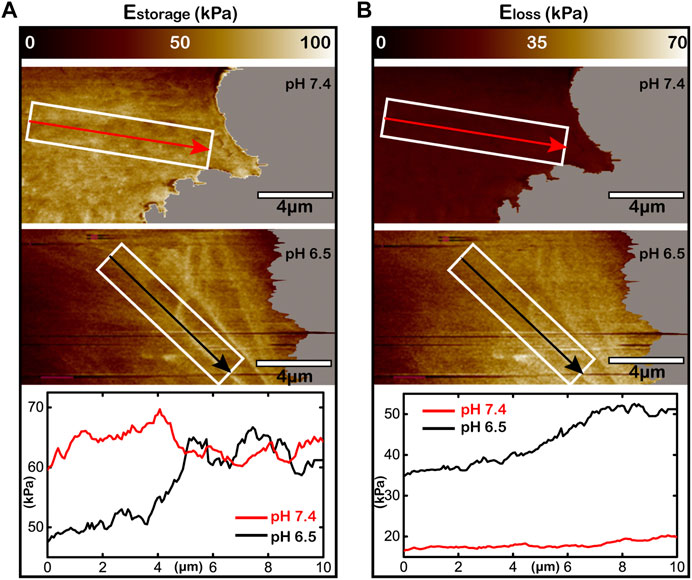
FIGURE 7. Acid microenvironment inducing viscoelasticity gradient in the lamellipodium of living Huh-7 cells. (A,B) The storage modulus for elasticity (A) and the loss modulus for viscosity (B) of the lamellipodium under different pH environments. The upper panels are at pH 7.4, the middle panels are at pH 6.5, and the lower curves analyze the storage modulus or the loss modulus within the white rectangular boxes along the arrows directed to lamellipodium protrusion. The white rectangular boxes with the directions of arrows are the same for each pH value.
Recent researches of cell migration and cancer metastasis have reported that the living cancer cells prefer to migrate toward the substrate with higher stiffness [19, 53]. However, few studies focus on the distribution and alteration of cell viscoelasticity during migration associated with the effects of cell viscoelasticity on migration. According to literatures, the higher elasticity and viscosity enable stronger adhesion to substrate [13, 54]. In our work, by using the improved AM-FM AFM, we found that the F-actin cytoskeleton aggregate and form the thick bundle-like filaments directed to the protruding direction of lamellipodium, leading to gradient increases in elasticity and viscosity. The gradient of viscoelasticity suggests a gradient of adhesion strength, which means that the lamellipodium can achieve stable adhesion at the front but have high possibility to detach the substrate at the back, resulting in the driving force for cell migration towards the direction of lamellipodium protrusion. Moreover, the aggregation and the stiffening of F-actin cytoskeleton allow focal adhesions to enhance cell adhesion, and provide sufficient cell rigidity against the forces needed for migration [55, 56]. In all, the homogeneity of F-actin cytoskeleton and viscoelasticity in the lamellipodium at pH 7.4 suppresses the migration of cancer cells, which consistent with the fact of uneasy malignant transformation of cancer cells under normal environment [47, 57]. Conversely, the aggregation of F-actin cytoskeleton and the gradient of viscoelasticity at pH 6.5 facilitate cell adhesion and migration, which explains the strong tendency in migration and metastasis of cancer cells under the acidified microenvironment in tumor tissues [47, 58]. These findings may help us to understand the structural and nanomechanical mechanism of malignant behavior of cancer cells under different pH microenvironments.
Conclusions
In summary, we have improved the original AM-FM AFM assembly, achieved the simultaneous mappings of topography and viscoelasticity of living cells under physiological conditions, and reached an ultrahigh resolution down to 50 nm for the imaging of F-actin cytoskeletons. By using the improved AM-FM AFM, we were able to study the effect of pH microenvironment on organization of F-actin cytoskeletons and the distribution of viscoelasticity in lamellipodia of living Huh-7 cancer cells correlated with the cell adhesion and migration. The acidified environment can transform the short, thin and dispersed F-actin structures to the long, thick and oriented bundle-like structure converged along the protruding direction of lamellipodium. The conformation of F-actin cytoskeletons leads to the gradient increases of both elasticity and viscosity directed to the lamellipodium front, which provides sufficient driving force and rigidity that can facilitate cell adhesion and migration. Our work has paved the avenue for the in situ characterization of the F-actin microstructures and cell viscoelasticity, and contributed insights into the structural origins and nanomechanical mechanism of the migration and metastasis of living cancer cells under the acidified microenvironment.
Data Availability Statement
The original contributions presented in the study are included in the article/Supplementary Material, further inquiries can be directed to the corresponding authors.
Author Contributions
WZ, XZ, and YZ conceived the research. MC, WZ, XZ, ZL, and SY designed, performed, and analyzed the experiments. WZ supervised the project. MC, WZ, XZ, and YZ wrote the manuscript. All authors discussed the results and commented on the manuscript.
Funding
This study was supported by the National Natural Science Foundation of China (Grants 11972383 and 81827802) to WZ, by the National Natural Science Foundation of China (Grant 11672339) to YZ, by the Fundamental Research Funds for the Central Universities (Grant 19LGPY258) to WZ, and by the Natural Science Foundation of Guangdong Province, China (Grant 2021A1515010348) to WZ. The funders had no influence on study design, data collection and analysis, decision to publish or preparation of the manuscript.
Conflict of Interest
The authors declare that the research was conducted in the absence of any commercial or financial relationships that could be construed as a potential conflict of interest.
Acknowledgments
The experiments reported were conducted on the Physical Research Platform in School of Physics, Sun Yat-sen University (PRPSP, SYSU) and the Instrumental Analysis and Research Center, Sun Yat-sen University. We thank Teaching Center of Biology Experiment, Sun Yat-sen University for assistance with confocal fluorescence microscopy imaging.
Supplementary Material
The Supplementary Material for this article can be found online at: https://www.frontiersin.org/articles/10.3389/fphy.2021.674958/full#supplementary-material
References
1. Ellinger I, Ellinger A. Smallest Unit of Life: Cell Biology. In: E Jensen-Jarolim, editor. Comparative Medicine Anatomy and Physiology. Germany: Springer Press (2014). p. 19–33. doi:10.1007/978-3-7091-1559-6_2
2. Lange JR, Fabry B. Cell and Tissue Mechanics in Cell Migration. Exp Cel Res (2013) 319(16):2418–23. doi:10.1016/j.yexcr.2013.04.023
3. Khalili A, Ahmad M. A Review of Cell Adhesion Studies for Biomedical and Biological Applications. Int J Mol Sci (2015) 16:18149–84. doi:10.3390/ijms160818149
4. Engler AJ, Sen S, Sweeney HL, Discher DE. Matrix Elasticity Directs Stem Cell Lineage Specification. Cell (2006) 126(4):677–89. doi:10.1016/j.cell.2006.06.044
5. Wei Q, Huang C, Zhang Y, Zhao T, Zhao P, Butler P, et al. Mechanotargeting: Mechanics-dependent Cellular Uptake of Nanoparticles. Adv Mater (2018) 30(27):1707464. doi:10.1002/adma.201707464
6. Fletcher DA, Mullins RD. Cell Mechanics and the Cytoskeleton. Nature (2010) 463(7280):485–92. doi:10.1038/nature08908
7. Pollard TD, Cooper JA. Actin, a Central Player in Cell Shape and Movement. Science (2009) 326(5957):1208–12. doi:10.1126/science.1175862
8. Blanchoin L, Boujemaa-Paterski R, Sykes C, Plastino J. Actin Dynamics, Architecture, and Mechanics in Cell Motility. Physiol Rev (2014) 94(1):235–63. doi:10.1152/physrev.00018.2013
9. Fritzsche M, Erlenkämper C, Moeendarbary E, Charras G, Kruse K. Actin Kinetics Shapes Cortical Network Structure and Mechanics. Sci Adv (2016) 2(4):e1501337. doi:10.1126/sciadv.1501337
10. Chugh P, Paluch EK. The Actin Cortex at a Glance. J Cel Sci (2018) 131(14). doi:10.1242/jcs.186254
11. Oberleithner H, Callies C, Kusche-Vihrog K, Schillers H, Shahin V, Riethmüller C, et al. Potassium Softens Vascular Endothelium and Increases Nitric Oxide Release. Proc Natl Acad Sci U S A (2009) 106(8):2829–34. doi:10.1073/pnas.0813069106
12. Tojkander S, Gateva G, Lappalainen P. Actin Stress Fibers - Assembly, Dynamics and Biological Roles. J Cel Sci (2012) 125(8):1855–64. doi:10.1242/jcs.098087
13. Li L, Zhang W, Wang J. A Viscoelastic-Stochastic Model of the Effects of Cytoskeleton Remodelling on Cell Adhesion. R Soc Open Sci (2016) 3(10):160539. doi:10.1098/rsos.160539
14. Jacquemet G, Hamidi H, Ivaska J. Filopodia in Cell Adhesion, 3D Migration and Cancer Cell Invasion. Curr Opin Cel Biol (2015) 36:23–31. doi:10.1016/j.ceb.2015.06.007
15. Bergert M, Chandradoss SD, Desai RA, Paluch E. Cell Mechanics Control Rapid Transitions between Blebs and Lamellipodia during Migration. Proc Natl Acad Sci (2012) 109(36):14434–9. doi:10.1073/pnas.1207968109
16. Nemethova M, Auinger S, Small JV. Building the Actin Cytoskeleton: Filopodia Contribute to the Construction of Contractile Bundles in the Lamella. J Cel Biol (2008) 180(6):1233–44. doi:10.1083/jcb.200709134
17. Mueller J, Szep G, Nemethova M, De Vries I, Lieber AD, Winkler C, et al. Load Adaptation of Lamellipodial Actin Networks. Cell (2017) 171(1):188–200. doi:10.1016/j.cell.2017.07.051
18. Bieling P, Li T-D, Weichsel J, McGorty R, Jreij P, Huang B, et al. Force Feedback Controls Motor Activity and Mechanical Properties of Self-Assembling Branched Actin Networks. Cell (2016) 164(1-2):115–27. doi:10.1016/j.cell.2015.11.057
19. Laurent VM, Kasas S, Yersin A, Schäffer TE, Catsicas S, Dietler G, et al. Gradient of Rigidity in the Lamellipodia of Migrating Cells Revealed by Atomic Force Microscopy. Biophysical J (2005) 89(1):667–75. doi:10.1529/biophysj.104.052316
20. Friedl P, Alexander S. Cancer Invasion and the Microenvironment: Plasticity and Reciprocity. Cell (2011) 147(5):992–1009. doi:10.1016/j.cell.2011.11.016
21. Rofstad EK, Mathiesen B, Kindem K, Galappathi K. Acidic Extracellular pH Promotes Experimental Metastasis of Human Melanoma Cells in Athymic Nude Mice. Cancer Res (2006) 66(13):6699–707. doi:10.1158/0008-5472.can-06-0983
22. Estrella V, Chen T, Lloyd M, Wojtkowiak J, Cornnell HH, Ibrahim-Hashim A, et al. Acidity Generated by the Tumor Microenvironment Drives Local Invasion. Cancer Res (2013) 73(5):1524–35. doi:10.1158/0008-5472.can-12-2796
23. Corbet C, Feron O. Tumour Acidosis: from the Passenger to the Driver’s Seat. Nat Rev Cancer (2017) 17(10):577–93. doi:10.1038/nrc.2017.77
24. Suzuki A, Maeda T, Baba Y, Shimamura K, Kato Y. Acidic Extracellular pH Promotes Epithelial Mesenchymal Transition in Lewis Lung Carcinoma Model. Cancer Cel Int (2014) 14(1):1–11. doi:10.1186/s12935-014-0129-1
25. Li S, Xiong N, Peng Y, Tang K, Bai H, Lv X, et al. Acidic pHe Regulates Cytoskeletal Dynamics through Conformational Integrin β1 Activation and Promotes Membrane Protrusion. Biochim Biophys Acta Mol Basis Dis (2018) 1864(7):2395–408. doi:10.1016/j.bbadis.2018.04.019
26. Efremov YM, Velay-Lizancos M, Weaver CJ, Athamneh AI, Zavattieri PD, Suter DM, et al. Anisotropy vs Isotropy in Living Cell Indentation with AFM. Sci Rep (2019) 9(1):1–12. doi:10.1038/s41598-019-42077-1
27. Brückner BR, Nöding H, Janshoff A. Viscoelastic Properties of Confluent MDCK II Cells Obtained from Force Cycle Experiments. Biophysical J (2017) 112(4):724–35. doi:10.1016/j.bpj.2016.12.032
28. Rianna C, Ventre M, Cavalli S, Radmacher M, Netti PA. Micropatterned Azopolymer Surfaces Modulate Cell Mechanics and Cytoskeleton Structure. ACS Appl Mater Inter (2015) 7(38):21503–10. doi:10.1021/acsami.5b06693
29. Calzado-Martín A, Encinar M, Tamayo J, Calleja M, San Paulo A. Effect of Actin Organization on the Stiffness of Living Breast Cancer Cells Revealed by Peak-Force Modulation Atomic Force Microscopy. ACS Nano (2016) 10(3):3365–74. doi:10.1021/acsnano.5b07162
30. Wang N, Zhang M, Chang Y, Niu N, Guan Y, Ye M, et al. Directly Observing Alterations of Morphology and Mechanical Properties of Living Cancer Cells with Atomic Force Microscopy. Talanta (2019) 191:461–8. doi:10.1016/j.talanta.2018.09.008
32. Henson JH, Yeterian M, Weeks RM, Medrano AE, Brown BL, Geist HL, et al. Arp2/3 Complex Inhibition Radically Alters Lamellipodial Actin Architecture, Suspended Cell Shape, and the Cell Spreading Process. Mol Biol Cel (2015) 26(5):887–900. doi:10.1091/mbc.e14-07-1244
33. Svitkina TM. Platinum Replica Electron Microscopy: Imaging the Cytoskeleton Globally and Locally. Int J Biochem Cel Biol (2017) 86:37–41. doi:10.1016/j.biocel.2017.03.009
34. Hochmuth RM. Micropipette Aspiration of Living Cells. J Biomech (2000) 33(1):15–22. doi:10.1016/S0021-9290(99)00175-X
35. Peschetola V, Laurent VM, Duperray A, Michel R, Ambrosi D, Preziosi L, et al. Time-dependent Traction Force Microscopy for Cancer Cells as a Measure of Invasiveness. Cytoskeleton (2013) 70(4):201–14. doi:10.1002/cm.21100
36. Ayala YA, Pontes B, Ether DS, Pires LB, Araujo GR, Frases S, et al. Rheological Properties of Cells Measured by Optical Tweezers. BMC Biophys (2016) 9(1):1–11. doi:10.1186/s13628-016-0031-4
37. Hu S, Eberhard L, Chen J, Love JC, Butler JP, Fredberg JJ, et al. Mechanical Anisotropy of Adherent Cells Probed by a Three-Dimensional Magnetic Twisting Device. Am J Physiol Cel Physiol (2004) 287(5):C1184–C1191. doi:10.1152/ajpcell.00224.2004
38. Krieg M, Fläschner G, Alsteens D, Gaub BM, Roos WH, Wuite GJL, et al. Atomic Force Microscopy-Based Mechanobiology. Nat Rev Phys (2019) 1(1):41–57. doi:10.1038/s42254-018-0001-7
39. Haase K, Pelling AE. Investigating Cell Mechanics with Atomic Force Microscopy. J R Soc Interf (2015) 12(104):20140970. doi:10.1098/rsif.2014.0970
40. Iyer S, Gaikwad RM, Subba-Rao V, Woodworth CD, Sokolov I. Atomic Force Microscopy Detects Differences in the Surface Brush of Normal and Cancerous Cells. Nat Nanotech (2009) 4(6):389–93. doi:10.1038/nnano.2009.77
41. Efremov YM, Dokrunova AA, Bagrov DV, Kudryashova KS, Sokolova OS, Shaitan KV. The Effects of Confluency on Cell Mechanical Properties. J Biomech (2013) 46(6):1081–7. doi:10.1016/j.jbiomech.2013.01.022
42. Kocun M, Labuda A, Meinhold W, Revenko I, Proksch R. Fast, High Resolution, and Wide Modulus Range Nanomechanical Mapping with Bimodal Tapping Mode. ACS Nano (2017) 11(10):10097–105. doi:10.1021/acsnano.7b04530
43. Proksch R, Yablon DG. Loss Tangent Imaging: Theory and Simulations of Repulsive-Mode Tapping Atomic Force Microscopy. Appl Phys Lett (2012) 100(7):073106. doi:10.1063/1.3675836
44. Guan D, Charlaix E, Qi RZ, Tong P. Noncontact Viscoelastic Imaging of Living Cells Using a Long-Needle Atomic Force Microscope with Dual-Frequency Modulation. Phys Rev Appl (2017) 8(4):044010. doi:10.1103/PhysRevApplied.8.044010
45. Haga H, Sasaki S, Kawabata K, Ito E, Ushiki T, Sambongi T. Elasticity Mapping of Living Fibroblasts by AFM and Immunofluorescence Observation of the Cytoskeleton. Ultramicroscopy (2000) 82(1-4):253–8. doi:10.1016/S0304-3991(99)00157-6
46. Webb BA, Chimenti M, Jacobson MP, Barber DL. Dysregulated pH: a Perfect Storm for Cancer Progression. Nat Rev Cancer (2011) 11(9):671–7. doi:10.1038/nrc3110
47. Mogilner A, Rubinstein B. The Physics of Filopodial Protrusion. Biophysical J (2005) 89(2):782–95. doi:10.1529/biophysj.104.056515
48. Guerrero CR, Garcia PD, Garcia R. Subsurface Imaging of Cell Organelles by Force Microscopy. ACS Nano (2019) 13(8):9629–37. doi:10.1021/acsnano.9b04808
49. Gardel ML, Shin JH, MacKintosh FC, Mahadevan L, Matsudaira P, Weitz DA. Elastic Behavior of Cross-Linked and Bundled Actin Networks. Science (2004) 304(5675):1301–5. doi:10.1126/science.1095087
50. Mattila PK, Lappalainen P. Filopodia: Molecular Architecture and Cellular Functions. Nat Rev Mol Cel Biol (2008) 9(6):446–54. doi:10.1038/nrm2406
51. Rigato A, Miyagi A, Scheuring S, Rico F. High-frequency Microrheology Reveals Cytoskeleton Dynamics in Living Cells. Nat Phys (2017) 13(8):771–5. doi:10.1038/nphys4104
52. Dancker P, Löw I, Hasselbach W, Wieland T. Interaction of Actin with Phalloidin: Polymerization and Stabilization of F-Actin. Biochim Biophys Acta (1975) 400(2):407–14. doi:10.1016/0005-2795(75)90196-8
53. Ponti A, Machacek M, Gupton SL, Waterman-Storer CM, Danuser G. Two Distinct Actin Networks Drive the Protrusion of Migrating Cells. Science (2004) 305(5691):1782–6. doi:10.1126/science.1100533
54. Yao H, Gao H. Mechanics of Robust and Releasable Adhesion in Biology: Bottom-Up Designed Hierarchical Structures of Gecko. J Mech Phys Sol (2006) 54(6):1120–46. doi:10.1016/j.jmps.2006.01.002
55. Natale CF, Ventre M, Netti PA. Tuning the Material-Cytoskeleton Crosstalk via Nanoconfinement of Focal Adhesions. Biomaterials (2014) 35(9):2743–51. doi:10.1016/j.biomaterials.2013.12.023
56. Qian J, Wang J, Lin Y, Gao H. Lifetime and Strength of Periodic Bond Clusters between Elastic Media under Inclined Loading. Biophysical J (2009) 97(9):2438–45. doi:10.1016/j.bpj.2009.08.027
57. White KA, Grillo-Hill BK, Barber DL. Cancer Cell Behaviors Mediated by Dysregulated pH Dynamics at a Glance. J Cel Sci (2017) 130(4):663–9. doi:10.1242/jcs.195297
Keywords: amplitude modulation-frequency modulation atomic force microscopy, living cancer cells, viscoelasticity gradient, F-actin cytoskeleton, lamellipodium, pH microenvironments, migration
Citation: Chen M, Zhu W, Liang Z, Yao S, Zhang X and Zheng Y (2021) Effect of F-Actin Organization in Lamellipodium on Viscoelasticity and Migration of Huh-7 Cells Under pH Microenvironments Using AM-FM Atomic Force Microscopy. Front. Phys. 9:674958. doi: 10.3389/fphy.2021.674958
Received: 02 March 2021; Accepted: 29 April 2021;
Published: 13 May 2021.
Edited by:
Ying Li, University of Connecticut, United StatesReviewed by:
Zhao Qin, Syracuse University, United StatesLei Tao, University of Connecticut, United States
Copyright © 2021 Chen, Zhu, Liang, Yao, Zhang and Zheng. This is an open-access article distributed under the terms of the Creative Commons Attribution License (CC BY). The use, distribution or reproduction in other forums is permitted, provided the original author(s) and the copyright owner(s) are credited and that the original publication in this journal is cited, in accordance with accepted academic practice. No use, distribution or reproduction is permitted which does not comply with these terms.
*Correspondence: Wenpeng Zhu, zhuwp3@mail.sysu.edu.cn; Xiaoyue Zhang, zhangxy26@mail.sysu.edu.cn