- Research Center of Laser Fusion, China Academy of Engineering Physics, Mianyang, China
In this work, an all-fiberized polarization-maintained (PM) fiber laser has been demonstrated with a near-top-hat-shaped spectrum. By optimizing the modulation signal to generate near-top-hat-shaped spectrums, a 3-kW PM fiber laser has been achieved at <10 GHz linewidth with the polarization extinction ratio of 96% and beam quality of 1.156, which is the highest output power ever reported with approximately 10 GHz linewidth, and further scaling of output power is limited by stimulated Brillouin scattering. By decomposing the mode content, the proportion of the fundamental mode in the output laser is above 97%. The stimulated Raman scattering suppression ratio reaches 62 dB at the maximal output power.
1 Introduction
High-power linearly polarized fiber lasers with a narrow linewidth and near-diffraction-limited beam quality are required in various scientific, industrial, and advanced applications, such as the coherent LiDAR system, non-linear frequency conversion, and beam combining [1, 2]. Due to the high demand for high-power linearly polarized fiber lasers, researchers have made great progress on power scaling of narrow-linewidth polarization-maintained (PM) fiber lasers in the last few years, and even a 5-kW PM fiber laser has been reported [3]. To suppress the onset of detrimental stimulated Brillouin scattering (SBS) effects, the linewidth of these multi-kilowatt linearly polarized fiber lasers has been broadened to several tens of GHz, which is a key factor in the penetration of fiber lasers in the aforementioned applications. Taking the beam combining applications, for example, which is an opportune method to break through the brightness limitation of the fiber laser system, the linewidth of PM laser is generally required to be within 10 GHz to achieve higher combining efficiency and better beam quality [4]. Specially designed spectral broadening formats have been proposed to achieve high-power PM lasers with 10 GHz linewidth, including pseudo-random binary sequence (PRBS) [5–7], filtered white noise signal (WNS) [8–12], multi-phase coded signal (MPCS) modulation [13], and near-top-hat-shaped spectrum [14–18]. In recent years, great progress has been made in power scaling of PM fiber laser within 10 GHz linewidth [5–19], and the highest output power reaches 2.3 kW with 8.5 GHz linewidth using a near-top-hat-shaped spectrum seed laser in public reports [15].
In this paper, we conducted a PM narrow-linewidth all-fiber laser experiment with near-top-hat-shaped signal modulation formats. The PM laser delivered 3-kW single-mode laser at < 10 GHz linewidth by applying the near-top-hat-shaped signal modulation format. At 3 kW output power, the polarization extinction ratio (PER) was 96%, and the beam quality was 1.156. A high optical signal-to-noise ratio (OSNR) was achieved with the stimulated Raman scattering (SRS) suppression ratio of approximately 62 dB, and further power scaling of the laser was limited by the onset of SBS.
2 Materials and methods
2.1 Optimization of the phase modulation signal
For fiber lasers with a narrower linewidth, the match of the optical length can be simplified for coherent combining systems [20], and the systems can be more robust against environmental perturbations. In order to scale the output power of the PM fiber laser system with narrower spectral linewidths, the modulation signal is optimized. Comparing it to common WNS and PRBS modulation formats, the top-hat-shaped spectrum can reach the highest SBS threshold with the same linewidth [21, 22], and the top-hat-shaped spectrum has a longer coherent length [18], so we optimize the phase modulation signal to obtain the top-hat-shaped spectrum.
The generation of the top-hat-shaped spectrum by phase modulation is to make the spectrum of modulated lasers rectangular in theory. The spectrum of the modulated laser P(υ) is expressed as the Fourier transform of the modulated laser:
where E0 is the amplitude of the laser and φ(t) is the phase modulation signal. Since the Fourier transform of the sinc function is rectangular, E0eiφ(t) = sint/t, but the equation has no real number solution. Therefore, it is impossible to modulate a single-frequency laser to a perfect top-hat-shaped spectrum by phase modulation. We can only optimize the modulation signal to make the phase-modulated spectrum as close to the top-hat-shaped spectrum as possible. The optimization method is established, as illustrated in Figure 1.
First, we define the target spectrum Ptarget(υ) and the modulated spectrum P(υ). Next, the cost function is established as Σi (P(i)-Ptarget(i))2, and the phase modulation signal φ(j), j = 1, 2 … N-1, N is initialized. Then, we optimize the phase modulation signal by the grid search method to make the cost function reach a stable value. The specific method is to search φ(1) in [-π, π] to minimize the cost function, while fixing the other N-1 points, and φ(1) is determined followed by φ(2) and φ(3) until φ(N) by using the same method; this is the first iteration process. We repeat several iterations until the cost function reaches a stable value, and then, the optimized phase modulation signal φ(j) is obtained. By this optimization method, the arbitrary-shaped modulation spectrum can be realized theoretically by changing the target spectrum. When the target spectrum is the top-hat-shaped spectrum, the cost function and SBS threshold versus iteration number is shown in Figure 2. With the increase in the iteration number, the cost function decreases and the SBS threshold increases. When the iteration number is above 25, the cost function and SBS threshold remain stable, which indicates that the phase modulation signal has reached the optimal state.
When the iteration number is 25, the optimized phase modulation signal and spectrum produced by optimized phase modulation are shown in Figures 3A, B. It can be seen that the near-top-hat-shaped spectrum is realized by optimizing the phase modulation signal.
2.2 Experimental setup
Figure 4 shows the experimental setup, which is based on a master oscillator power amplification (MOPA) structure. The seed was a 1064-nm single-frequency linearly polarized fiber laser with a linewidth of 10 kHz, delivering an output power of 20 mW. The seed was phase-modulated by a phase modulator, driven by an amplified modulation signal to broaden the linewidth of the seed to suppress SBS in an amplifier. The broadened seed laser was amplified by a three-stage all-fiber amplifier. Two pre-amplifiers boosted the laser seed to approximately 20 W, where a PM Yb-doped fiber (YDF) with a core/inner-cladding diameter of 10/125 μm was used. Then, the pre-amplified laser was injected into the main amplifier through a PM mode field adaptor (MFA). Between the PM MFA and pre-amplifiers, a PM isolator (ISO) was inserted to prevent damage from the backward light, and the multi-mode fiber port of ISO was used to monitor the backward power and backward spectrum. The main amplifier was constructed by PM YDF with a mode field diameter of 20 μm, which was coiled on a water-cooled aluminous plate. To enhance MI suppression, the backward pump configuration was employed in the main amplifier stage, and six laser diode modules centered at 976 nm were coupled into the YDF through a (6 + 1) × 1 PM signal/pump combiner. Two home-made PM cladding power strippers (CPSs) were used to strip the residual pump light and cladding signal light. An anti-reflection coated end cap was used to deliver and collimate the amplified laser.
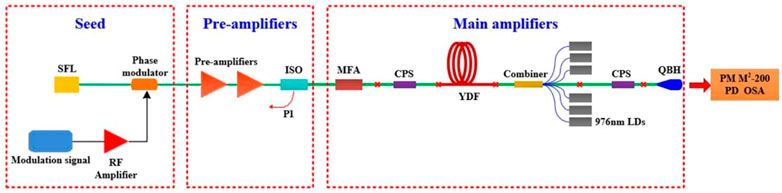
FIGURE 4. Experimental setup of the PM narrow-linewidth fiber laser (SFL, single-frequency laser; RF, radio frequency; ISO, isolator; MFA, mode field adaptor; CPS, cladding power stripper; YDF, Yb-doped fiber; QBH, quartz block head; PD, photo-detector; OSA, optical spectrum analyzer; PM, power meter).
3 Experimental results and discussion
The optimized modulation signal is used to drive the phase modulator to broaden the linewidth of the single-frequency laser, and the spectrum is measured by an optical spectrum analyzer (OSA) with the spectral resolution of 2 pm, as shown in Figure 5. The spectrum presents a near-top-hat-shaped profile, and the full width at half maximum (FWHM) is 18 pm. However, compared with the theoretical results, there are some large power fluctuations and obvious sidebands in the spectrum. The main reason is that the RF amplifier and phase modulator used in the experiment have non-linear response coefficients at different frequencies, leading to the distortion of the experimental results.
The seed laser is amplified to approximately 20 W using two pre-amplifiers. By injecting the pre-amplified seed into the main amplifier, the output power and backward power of a PM fiber amplifier versus the pump power are shown in Figure 6. With the scaling of pump power, the output power of the fiber laser increases linearly. When the pump power is 3,860 W, the output power reaches 3,050 W, and the optical-to-optical efficiency is approximately 78%. At 3 kW output power, the backward power is approximately 25 mW.
The spectrum is also measured by an OSA with a spectral resolution of 0.02 nm, and output spectrums and backward spectrums at different output powers are shown in Figure 7. The output spectrums are shown in Figure 7A, and the fine output spectrum from 1,063.5 nm to 1,063.9 nm is shown in Figure 7A. Due to the limitation of the spectral resolution, the measured FWHM of the seed laser is 0.04 nm, and the linewidth slightly broadened to 0.04 nm at the maximum output power. The broadening of the spectral substrate is due to amplified spontaneous emission (ASE), and no Raman light has been observed at the maximal output power, meaning that the SRS suppression ratio is >62 dB. The backward spectrums at different output powers are shown in Figure 7B. SBS increases obviously with the scaling of the output power, and the laser produces random intensity peaks in the backward light spectrum at 3,050 W output power, which indicates that laser power has reached the SBS threshold [23].
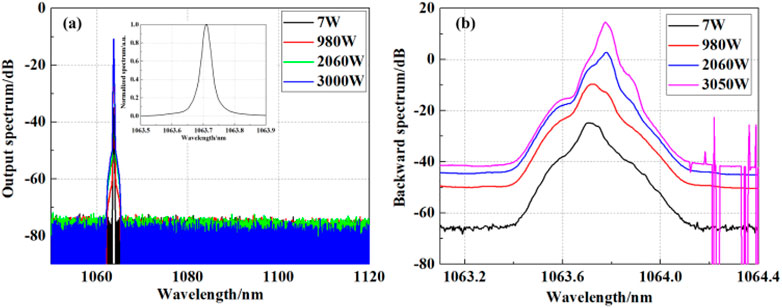
FIGURE 7. (A) Output spectrums and (B) backward spectrums at different output powers for the PM fiber laser system.
The beam quality factors M2 at different output powers are shown in Figure 8A. The beam quality of the seed laser is M2 = 1.19, and the fiber laser maintains the near-diffraction-limited beam quality with the scaling of the output power. The beam quality at 3 kW is shown in Figure 8B, which is Mx2 = 1.134 and My2 = 1.178. M2 is calculated as
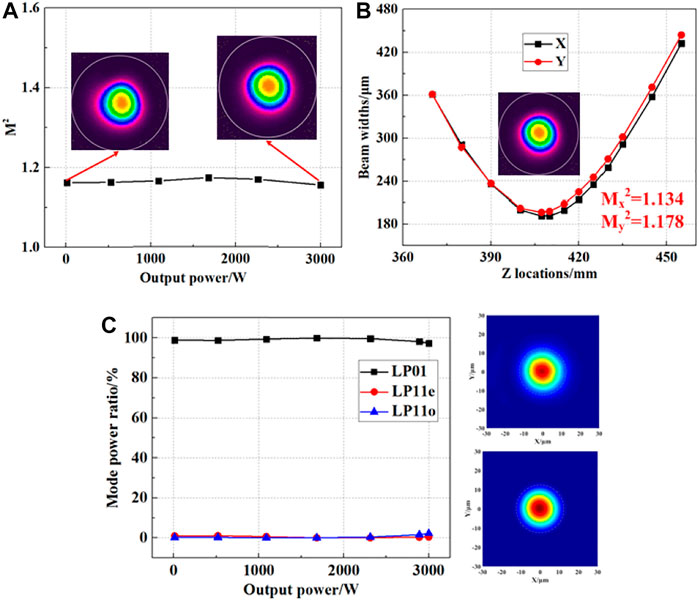
FIGURE 8. (A) M2 factors at different output powers, (B) beam quality at 3 kW, and (C) power proportion of LP01, LP11e, and LP11o modes at different output powers and the output spot (top) and mode reconstruction spot (bottom) at 3 kW.
The output power of the p-polarization light and s-polarization light and the PER at different pump powers are shown in Figure 9, which are measured using the assemble components of a half-wavelength plate and a polarization plate. The measured PER changes between 95.77% (14.91 dB) and 97.52% (18.31 dB) for PM fiber amplifiers with approximately 2% fluctuation with the increase in the output power. At the maximum output power, the PER is measured to be approximately 96%.
4 Conclusion
In this paper, a high-power PM narrow-linewidth fiber laser based on the MOPA structure was built. Based on this system, we optimized the modulation signal by using the grid search method to obtain the near-top-hat-shaped spectrum. With the near-top-hat-shaped spectrum modulation signal, a 3-kW linearly polarized laser was realized with <10 GHz spectrum linewidth and near-diffraction-limited beam quality, which is the highest output power for the PM fiber laser reported so far, with a linewidth of approximately 10 GHz.
Data availability statement
The original contributions presented in the study are included in the article/supplementary material; further inquiries can be directed to the corresponding author.
Author contributions
All authors listed have made a substantial, direct, and intellectual contribution to the work and approved it for publication.
Funding
This work was supported by the National Natural Science Foundation of China (No. 62205317).
Conflict of interest
The authors declare that the research was conducted in the absence of any commercial or financial relationships that could be construed as a potential conflict of interest.
Publisher’s note
All claims expressed in this article are solely those of the authors and do not necessarily represent those of their affiliated organizations, or those of the publisher, the editors, and the reviewers. Any product that may be evaluated in this article, or claim that may be made by its manufacturer, is not guaranteed or endorsed by the publisher.
References
1. Zheng Y, Zhu Z, Liu X, Yu M, Li S, Zhang L, et al. High power, high-beam-quality spectral beam combination of six narrow-linewidth fiber amplifiers with two transmission diffraction gratings. Appl Opt (2019) 58:8339–43. doi:10.1364/AO.58.008339
2. Zeng X, Cui S, Cheng X, Zhou J, Qi W, Feng Y, Resonant frequency doubling of phase-modulation-generated few-frequency fiber laser. Opt Lett (2020) 45:4944–7. doi:10.1364/OL.401348
3. Wang Y, Feng Y, Peng W, Sun Y, Yang X, Wang Y, et al. 5kW near diffraction limit high extinction ratio narrow linewidth polarization maintaining fiber laser. High Power Laser Part Beams (2022) 34:112002.
4. Gregory DG, Chun-Ching S, Joshua ER, Perturbative analysis of coherent combining efficiency with mismatched lasers. Opt Express (2010) 18:25403–14. doi:10.1364/OE.18.025403
5. Flores A, Robin C, Lanari A, Dajani I, Pseudo-random binary sequence phase modulation for narrow linewidth, kilowatt, monolithic fiber amplifiers. Opt Express (2014) 22:17735–44. doi:10.1364/OE.22.017735
6. Liu M, Yang Y, Shen H, Zhang J, Zou X, Wang H, et al. 1.27kW, 2.2GHz pseudo-random binary sequence phase modulated fiber amplifier with Brillouin gain-spectrum overlap. Scientific Rep (2020) 10:629. doi:10.1038/s41598-019-57408-5
7. Jun C, Jung M, Shin W, Yu BA, Yoon YS, Park Y, et al. 818W Yb-doped amplifier with <7GHz linewidth based on pseudo-random phase modulation in polarization maintained all-fiber configuration. Laser Phys Lett (2019) 16:015102. doi:10.1088/1612-202X/aaee11
8. Ma P, Tao R, Su R, Wang X, Zhou P, Liu Z, 1.89kW all-fiberized and polarization-maintained amplifiers with narrow linewidth and near-diffraction-limited beam quality. Opt Express (2016) 24(4):4187–95. doi:10.1364/OE.24.004187
9. Su R, Tao R, Wang X, Zhang H, Ma P, Zhou P, et al. 2.43 kW narrow linewidth linearly polarized all-fiber amplifier based on mode instability suppression. Laser Phys Lett (2017) 14:085102. doi:10.1088/1612-202X/aa760b
10. Huang Z, Shu Q, Tao R, Chu Q, Luo Y, Yan D, et al. 5kW record high power narrow linewidth laser from traditional step-index monolithic fiber amplifier. IEEE Photon Tech Lett (2021) 33:1181–4. doi:10.1109/LPT.2021.3112270
11. Chang Z, Wang Y, Sun Y, Peng W, Ke W, Ma Y, et al. 1.5kW polarization-maintained Yb-doped amplifier with 13GHz linewidth by suppressing the self-pulsing and stimulated Brillouin scattering. Appl Opt (2019) 58:6419–25. doi:10.1364/AO.58.006419
12. Wang Y, Feng Y, Ma Y, Chang Z, Peng W, Sun Y, et al. 2.5 kW narrow linewidth linearly polarized all-fiber MOPA with cascaded phase-modulation to suppress SBS induced self-pulsing. IEEE Photon J (2020) 12:1–15. doi:10.1109/JPHOT.2020.2997935
13. Lai W, Ma P, Song J, Ren S, Liu W, Zhou P, Kilowatt-level, narrow linewidth, polarization-maintained all-fiber amplifiers based on multi-phase coded signal modulation and laser gain competition. Results Phys (2021) 31:105050. doi:10.1016/j.rinp.2021.105050
14. Harish AV, Nilsson J, Optimization of phase modulation with arbitrary waveform generators for optical spectral control and suppression of stimulated Brillouin scattering. Opt Express (2015) 23:6988–99. doi:10.1364/OE.23.006988
16. Harish AV, Nilsson J J, Optimization of phase modulation formats for suppression of stimulated Brillouin scattering in optical fibers. IEEE J Selected Top Quan Elect (2018) 24:1–10. doi:10.1109/JSTQE.2017.2753041
17. Li W, Ma P, Chen Y, Ren S, Yu D, Liu W, et al. Confined-doped fiber enabled kilowatt-level all-fiber laser with 1.28GHz linewidth. Opt Express (2023) 31(5):8855–63. doi:10.1364/OE.484330
18. Li W, Ma P, Chen Y, Song J, Lai W, Liu W, et al. 694W sub-GHz polarization-maintained tapered fiber amplifier based on spectral and pump wavelength optimization. Opt Express (2022) 30(15):26875–85. doi:10.1364/OE.463082
19. Edgecumbe J, Björk D, Galipeau J, Boivin G, Christensen S, Samson B, et al. Kilowatt-Level PM amplifiers for beam combining. Front Opt 2008. Rochester, New York USA: FTuJ2 (2008). doi:10.1364/FIO.2008
20. Gregory DG, Stuart JM, Joshua ER, Timothy SM, Peter AT, Wickham MG, et al. Active phase and polarization locking of a 1.4 kW fiber amplifier. Opt Lett (2010) 35(10):1542–4. doi:10.1364/OL.35.001542
21. Yang Y, Li B, Liu M, Huang X, Feng Y, Cheng D, et al. Optimization and visualization of phase modulation with filtered and amplified maximal-length sequence for SBS suppression in a short fiber system: A theoretical treatment. Opt Express (2021) 29(11):16781–803. doi:10.1364/OE.426070
22. White JO, Young JT, Wei C, Hu J, Menyuk CR, Seeding fiber amplifiers with piecewise parabolic phase modulation for high SBS thresholds and compact spectra. Opt Express (2019) 27(3):2962–74. doi:10.1364/OE.27.002962
23. Panbhiharwala Y, Harish AV, Venkitesh D, Nilsson J, Srinivasan B, Investigation of temporal dynamics due to stimulated Brillouin scattering using statistical correlation in a narrow-linewidth CW high power fiber amplifier. Opt Express (2018) 26:33409–17. doi:10.1364/OE.26.033409
24. Yoda H, Polynkin P, Mansuripur M, Beam quality factor of higher order modes in a step-index fiber. J Lightwave Tech (2006) 24(3):1350–5. doi:10.1109/JLT.2005.863337
25. Huang L, Guo S, Leng J, Lu H, Zhou P, Cheng X, Real-time mode decomposition for few-mode fiber based on numerical method. Opt Express (2015) 23:4620–9. doi:10.1364/OE.23.004620
26. Chen F, Zhao S, Wang Q, Ma J, Lu Q, Wan J, et al. Modal decomposition of a fibre laser beam based on the push-broom stochastic parallel gradient descent algorithm. Opt Commun (2021) 481:126538. doi:10.1016/j.optcom.2020.126538
Keywords: polarization-maintained fiber lasers, stimulated Brillouin scattering, mode instability, high-power fiber lasers, phase modulation
Citation: Chu Q, Shu Q, Li F, Guo C, Yan Y, Zhang H, Liu Y, Tao R, Lin H and Wang J (2023) Power scaling of high-power linearly polarized fiber lasers with <10 GHz linewidth. Front. Phys. 11:1198305. doi: 10.3389/fphy.2023.1198305
Received: 01 April 2023; Accepted: 09 May 2023;
Published: 24 May 2023.
Edited by:
Takeo Minamikawa, Tokushima University, JapanReviewed by:
Yudong Lian, Hebei University of Technology, ChinaShuangqiang Liu, Sun Yat-sen University, China
Copyright © 2023 Chu, Shu, Li, Guo, Yan, Zhang, Liu, Tao, Lin and Wang. This is an open-access article distributed under the terms of the Creative Commons Attribution License (CC BY). The use, distribution or reproduction in other forums is permitted, provided the original author(s) and the copyright owner(s) are credited and that the original publication in this journal is cited, in accordance with accepted academic practice. No use, distribution or reproduction is permitted which does not comply with these terms.
*Correspondence: Qiuhui Chu, Y2h1cWl1aHVpQDE2My5jb20=