- Instituto de Biología Molecular y Celular de Plantas, Consejo Superior de Investigaciones Científicas – Universidad Politécnica de Valencia, Valencia, Spain
In plants, the only confirmed function for thermospermine is regulating xylem cells maturation. However, genes putatively encoding thermospermine synthases have been identified in the genomes of both vascular and non-vascular plants. Here, we verify the activity of the thermospermine synthase genes and the presence of thermospermine in vascular and non-vascular land plants as well as in the aquatic plant Chlamydomonas reinhardtii. In addition, we provide information about differential content of thermospermine in diverse organs at different developmental stages in some vascular species that suggest that, although the major role of thermospermine in vascular plants is likely to be xylem development, other potential roles in development and/or responses to stress conditions could be associated to such polyamine. In summary, our results in vascular and non-vascular species indicate that the capacity to synthesize thermospermine is conserved throughout the entire plant kingdom.
Introduction
Polyamines are positively charged aliphatic compounds with a widespread presence in all living organisms (Tabor and Tabor, 1984). The diamine putrescine (Put) and the triamine spermidine (Spd) are the most commonly found polyamines, while the tetra-amine spermine (Spm) is found in yeasts, most animals, seed plants and some bacteria (Pegg and Michael, 2010). Another tetra-amine, thermospermine (Tspm), has been detected in archaea, diatoms and plants, but not in animals or bacteria (Michael, 2016).
The ability to synthesize specific polyamines is clade-specific and is mainly the result of evolutionary adjustments in the polyamine biosynthesis pathway, reflected in the presence or absence of specific polyamine biosynthetic enzymes in given clades. Triamines and tetra-amines are mainly synthesized by a set of aminopropyl transferases, which are evolutionarily related to each other. It has been proposed that while Spm synthase (SPMS) genes in fungi and plants have likely emerged independently after duplication and neofunctionalization of Spd synthase (SPDS) genes (Minguet et al., 2008), the Tspm synthase (TSPMS) gene in plants was probably acquired through endosymbiosis of a cyanobacterium. However, it is arguable whether such gene originally encoded a TSPMS or an agmatine aminopropyl transferase (Pegg and Michael, 2010; Michael, 2016). All in all, the capacity to synthesize different polyamines in a given species is defined by (i) the presence or absence of specific polyamine biosynthetic enzymes and (ii) the degree of specificity of the polyamine biosynthesis enzymes toward their substrates. For instance, it has been reported that an aminopropyl transferase from the archaea Pyrobaculum aerophilum displays its highest specificity in vitro toward norspermidine, resulting in norspermidine biosynthesis, but it is also able to synthesize Tspm from Spd (Knott et al., 2007). Similarly, the gymnosperm Pinus sylvestris lacks a specific SPMS gene, but the aminopropyl transferase encoded by PsSPDS efficiently converts Put to Spd, as well as Spd to Spm (Vuosku et al., 2018). Both this flexibility in substrate recognition and the repeated independent generation of new aminopropyl transferases along evolution can be explained by the alteration of a few key residues in the active site of aminopropyl transferases that determine their characteristic substrate discrimination, as proposed through structural modeling and crystal structure comparisons of active sites (Wu et al., 2007; Minguet et al., 2008; Sekula and Dauter, 2018).
In plants, polyamines have been implicated in the response to stress and in the modulation of developmental processes (Chen et al., 2018). Correlations between specific endogenous levels of polyamines in plants under different stress conditions or during the progression of specific developmental processes have been extensively reported. In addition, the effects provoked by exogenous polyamines application have been largely documented. However, beyond such physiological reports, solid evidences for polyamines roles come from the analysis of loss of function mutants in polyamine metabolism genes. In this way, Put and Spd have been proved to be essential for life (Imai et al., 2004), while the tetra-amine Spm has been shown to be required for proper acclimation to salt, drought and heat stress (Yamaguchi et al., 2006, 2007; Sagor et al., 2013). Furthermore, both Spm and Tspm promote protection against bacterial pathogens (Gonzalez et al., 2011; Marina et al., 2013). However, the participation of Tspm could be a secondary effect of its primary function in vascular development (Vera-Sirera et al., 2010).
The most extensively studied role for a polyamine is that of Tspm in the regulation of xylem differentiation. The Arabidopsis acaulis5 (acl5) mutant, impaired in TSPMS activity, displays stunted growth (Hanzawa et al., 1997, 2000) which has been associated to an increase in vascular cell proliferation, premature xylem cell death and miss-regulated lignin deposition (Clay and Nelson, 2005; Muniz et al., 2008). In Arabidopsis, the ACL5 gene is specifically expressed in developing xylem cells (Clay and Nelson, 2005; Muniz et al., 2008), and the HD-ZIPIII transcription factor AtHB8 -which directs xylem differentiation and displays the same expression domain as ACL5- mediates its induction by auxin (Baima et al., 2014). Auxin has been shown to promote cell proliferation by ensuring the formation of complexes between Target of Monopteros5 (TMO5) and Lonesome Highway (LHW), leading to the induction of cytokinin biosynthesis (De Rybel et al., 2013, 2014). Recent evidence suggests that Tspm is part of a negative feed-forward loop triggered by auxin that maintains proliferation of vascular cells within the correct range (Katayama et al., 2015; Vera-Sirera et al., 2015). The mechanism involves the promotion of translation of a small family of Suppressor of ACL5 (SACL) transcriptional regulators (Imai et al., 2006; Kakehi et al., 2008) which compete with TMO5 for the formation of inactive SACL-LHW complexes (Katayama et al., 2015; Vera-Sirera et al., 2015). This is not the only case in which polyamines have been implicated in translational regulation (Hanfrey et al., 2002, 2005).
Systematic sequencing of plant transcriptomes and genomes has revealed the presence of ACL5 putative homologs in all plant lineages of vascular and non-vascular plants (including algae), raising the following questions: Do all putative ACL5 genes across plant lineages encode enzymes with bona-fide TSPMS activity, even those present in non-vascular species? Is the expression of these genes associated to xylem development in all vascular plants? To start answering these questions, we have (i) examined the enzymatic activity of the ACL5 homologs of several species in key plant lineages, (ii) searched for Tspm presence in vascular and non-vascular plants and (iii) studied the ACL5 expression pattern in vegetative and reproductive organs of several seed-plant species at different developmental stages.
Materials and Methods
Plant Material and Growth Conditions
Arabidopsis thaliana Columbia-0 (Col-0) plants were grown on MS media with sucrose (1%) under long-day conditions (16 h light, 8 h dark) at 22°C in a growth room. Samples for polyamine analysis and RNA extraction from Col-0 were taken after 15 days of growth. P. abies plant material was collected from adult trees that were identified in Catalonia (Spain) and frozen at –80°C until further analysis. S. lepidophylla plants were obtained from an external vendor, and hydrated before freezing the material at –80°C for further analysis. P. patens plant material was obtained from Jesús Vicente Carbajosa’s Lab (CBGP-Madrid, Spain) and grown in BCD medium + 1 mM Ca2+ under continuous light conditions in a growth room (Ashton and Cove, 1977). M. polymorpha Tak-1 plants were grown on ½ strength Gamborg’s B5 medium for 15 days before tissue recollection under continuous light conditions in a growth room. C. reinhardttii plant material was obtained from Federico Valverde’s Lab (IBVF-Sevilla, Spain).
Phylogenetic Analysis
Sequences used in this study were obtained by extensive BLAST analysis (tblastn) using the Arabidopsis thermospermine synthase (ACL5-AT5G19530), spermine synthase (SPMS-AT5G53120), and spermidine synthase 1 and 2 (SPDS1-AT1G23820, SPDS2-AT1G70310) genes as baits in the NCBI1, Phytozome2 and OneKP3 databases. All the sequences were managed using the Benchling tool4. Alignment of the sequences was done with using the MUSCLE algorithm (Siebers et al., 2017) included in the SeaView 4.6.4 GUI (Gouy et al., 2010), with 16 iterations, default clustering methods, gap open score of –2.7, and hydrophobicity multiplier of 1.2, followed by manual curation. To select the best-fit model of amino acid substitution, the ProtTest v3.4.2 (Darriba et al., 2011) was used on final multiple sequence alignment, together with AIC model for ranking. Maximum likelihood tree was produced with PhyML v3.1 (Guindon et al., 2010), using the best-scored model of amino acid substitution. Statistical significance was evaluated by bootstrap analysis of 1000 replicates. Phylogenetic tree graphical representations were initially generated using FigTree (version 1.4.3) software5, and final cartoons edited manually.
RNA Extraction and PCR Analysis
Total RNA was isolated from 200 mg of frozen powdered tissues using RNeasy plant mini kit (Qiagen) for A. thaliana and M. polymorpha tissues following the manufacturer’s instructions. RNA from C. reinhardtii, P. patens, S. lepidophylla and P. abies was extracted by Trizol-Chloroform method as described (Siebers et al., 2017). cDNA synthesis was performed on 1 μg DNase-treated RNA using PrimeScriptTM 1st strand cDNA synthesis kit (Takara). The resulting cDNA was used for PCR analysis with target gene-specific primers (Supplementary Table S2).
Cloning and Expression in Yeast
Coding sequence regions of thermospermine synthase genes from the selected species were obtained either from cDNA (M. polymorpha, S. lepidophylla and A. thaliana) or synthesized (Integrated DNA Technologies, IDT). The primers used for amplification are summarized in Supplementary Table S2. For plasmid construction, the CDS of the genes was cloned into pDONR207 through Gateway recombination (Invitrogen) and eventually into the destination vector pAG426GPD-ccdb-HA (a gift from Susan Lindquist; Addgene plasmid number 14252) for expression in yeast. The A. thaliana thermospermine synthase CDS was cloned into pCR8 through Golden Braid technology (Sarrion-Perdigones et al., 2011) and then shifted to Gateway technology to clone the CDS into the pDEST like the other species.
Yeast strain BY4741 (MATa his3 leu2 met15 ura3), kindly provided by Ramón Serrano’s lab (IBMCP-Valencia, Spain), was grown on Synthetic Defined (SD) medium and transformed with the pAG426GPD containing the CDS of interest by LiAc/ssDNA/PEG. Selected transformants were grown on 50 mL liquid SD medium lacking Trp and Ura for 2 days, then harvested by centrifugation (about 200 mg of pellet) and frozen for further polyamine quantification.
Polyamine Quantification
Polyamine extraction from tissues and standards was done with a modification of a previously described method (Naka et al., 2010) as follows. About 1 g of plant samples in 3 replicates were frozen in liquid nitrogen and kept at –80°C until use. The tissue was ground with mortar and pestle (in the case of yeast pellets, the cells were broken with glass balls) and resuspended in 2.5 mL of 5% perchloric acid (PCA) in a 15-mL tube. At this point 500 μL of the internal standard (diethylamine 1 mM, DEA) was added to the 5% PCA. The samples were kept on ice for 1 h and centrifuged at 4°C for 20 min at 15000×g. The whole supernatant was collected (between 2 and 3.5 mL) and transferred to a fresh 15 mL falcon tube. For each mL of supernatant, 0.66 mL of 2 M NaOH was added. Then, benzoylation started with the addition of 10 μL of benzoyl chloride. After 1 min vortex, plant extracts were left at room temperature for 20 min. Then, for each mL of initial supernatant used, 1.33 mL of saturated NaCl solution was added. Two mL of diethyl ether was added, vortexed, and centrifuged at 3000×g for 1 min for the separation of the phases. The supernatant was transferred to a new pyrex vial and dried completely using N2. The remaining polyamines were resuspended in 130 μL methanol and filtered with a filter syringe (pore size 0.2 μm). Then, the filtrate was transferred to a plastic vial for HPLC analysis. Briefly, 30-μL aliquots were injected through a Waters 717plus autosampler into a 1525 Waters Binary HPLC pump equipped with a 996 Waters PDA detector and using a Luna C18(2) (Phenomenex) column (250 × 4.6 mm, i.d. 5 μm). The column was equilibrated with 58% solvent A (acidic H2O containing 10 mL acetic acid for each liter of distilled water) and 42% solvent B (acetonitrile). Elution was carried out at room temperature and for polyamine separation a 1 mL min–1 flow rate was used the isocratic gradient of 42% acetonitrile for 25 min. Then, the column was washed with 42–100% acetonitrile within 3 min and kept at 100% acetonitrile for 10 min. Eventually, the column was equilibrated with 42% acetonitrile for 17 min before the next injection. Detection of polyamines was performed at 254 nm.
Expression Analysis
Data for TSPMS gene expression in A. thaliana, P. trichocarpa, M. truncatula, S. lycopersicum, Symphytum tuberosum, O. sativa, G. max, V. vinifera, B. distachyon, E. grandis, P. abies, Ananas comosus and P. patens in different tissues was gathered from the Bio-Analytic Resource for Plant Biology (BAR6). Expression data was classified according to the organ tissue (stem, root, flower and leaf) and tissue age (mature or young). The total expression data per gene and species in the target organs was used to calculate the percentage of expression of the TSPMS gene in each organ. Heatmaps were drawn using the Matrix2png tool7.
Results
Identification of Thermospermine Synthase Genes Across Plant Lineages
Polyamine biosynthesis enzymes display high degree of similarity (Hashimoto et al., 1998; Panicot et al., 2002; Teuber et al., 2007). Therefore, to guarantee accurate identification of putative ACL5 orthologs, we screened the Phytozome and OneKP databases using as baits the Arabidopsis ACL5 sequence, and also the sequences of the two Arabidopsis genes encoding SPDS, and the single gene for SPMS (see Section “Materials and Methods” for details). We obtained 542 sequences covering every major plant lineages and included members of representative bacteria, archaea and other non-plant eukaryotic groups, which were aligned and used for the construction of a phylogenetic tree (Figure 1A and Supplementary Files S1–S3). This is the most extensive phylogenetic study of plant polyamine aminopropyl transferases done to date, and it not only confirms some of the previous evolutionary models (Hashimoto et al., 1998; Panicot et al., 2002; Teuber et al., 2007; Minguet et al., 2008), but also provides new details on certain key events.
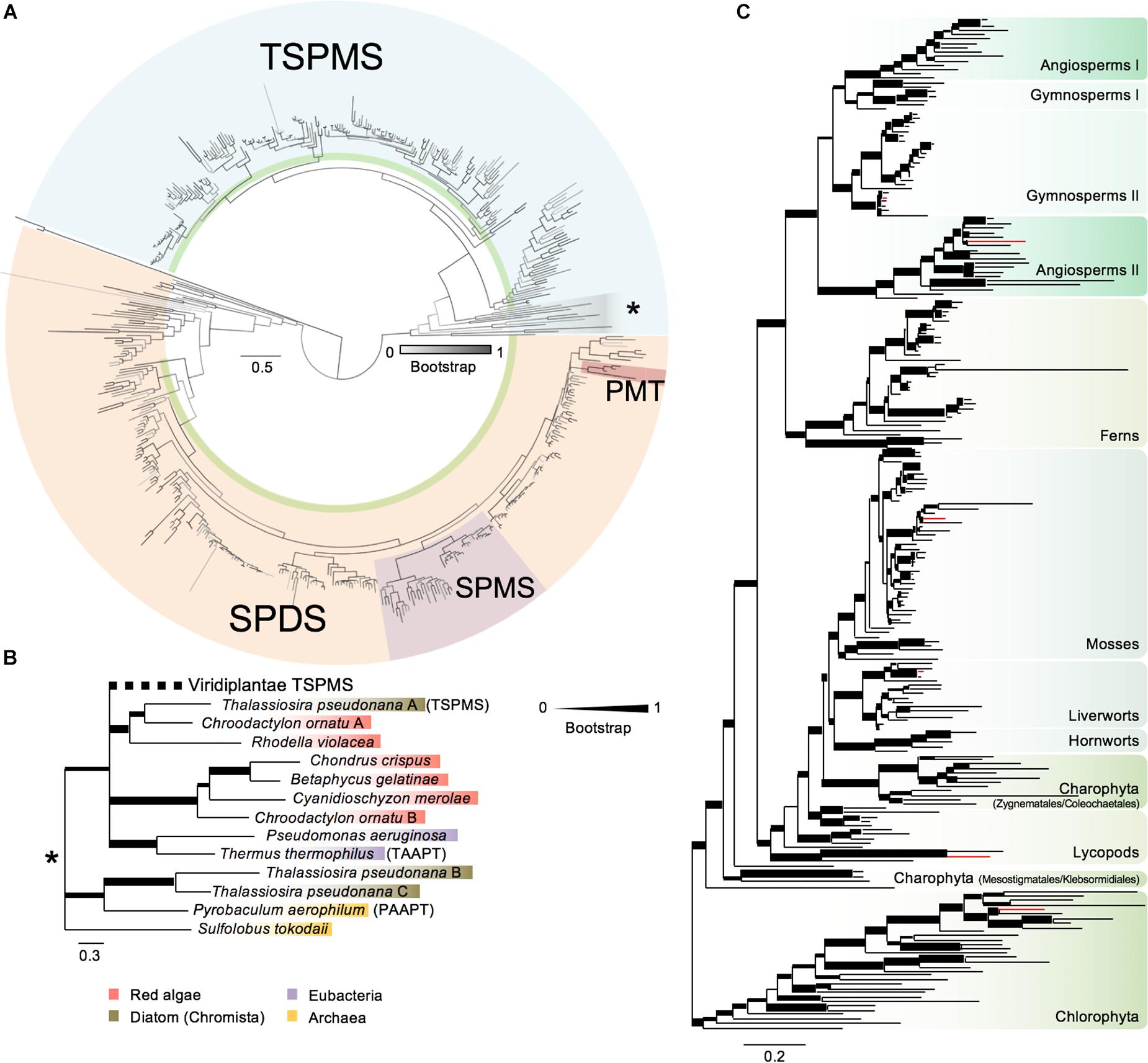
Figure 1. Phylogenetic analysis of polyamine aminopropyl transferases. (A) Phylogenetic analysis of polyamine aminopropyl transferases protein sequences with special focus in plant sequences. Support values associated with branches are maximum likelihood bootstrap values from 1000 replicates depicted as a color range (gray scale). Black branches indicate a bootstrap of 1 (100% support). Blue background indicates TSPMS clade sequences and light brown SPDS-related sequences. The remaining sequences belong to metazoan SPMS used as outgroups to root the tree. Purple and dark brown indicate SPMS sequences from Spermatophyta and PMT sequences from Solanaceae/Convolvulaceae, respectively. Green ribbons mark Viridiplantae sequences. Gray shaded sequences with an asterisk indicate basal branches of the TSPMS clade. (B) Phylogenetic tree of TSPMS basal branches extracted from panel (A), marked with an asterisk. Support values associated with branches and displayed as bar thickness are maximum likelihood bootstrap values from 1000 replicates. Values under 0.75 have been merged to ease visualization. Names in brackets indicate experimentally tested forms of aminopropyl transferases: TSPMS, thermospermine synthase; TAAPT, triamine/agmatine aminopropyl transferase; PAAPT, polyamine aminopropyl transferase. Viridiplantae sequences have been collapsed. (C) Phylogenetic analysis of Viridiplantae TSPMS from tree (A). Support values associated with branches and displayed as bar thickness are maximum likelihood bootstrap values from 1000 replicates. Background colors indicate sequences from major lineages of plants. Red colored branches indicate chosen sequences for experimental analysis. Scale bars in panels (A–C) indicate substitution per residue distances.
First, our analysis expands the presence of ACL5 homologs and SPDS genes to all plant lineages not previously studied, like charophytes and chlorophytes (Figure 1A). Second, the exclusive origin of SPMS genes in angiosperms, previously proposed based on only a few sequences (Minguet et al., 2008), is now set in the common ancestor of all Spermatophytes with more sequences from gymnosperms and their absence in multiple genomes of other land plants. Third, we find indications for a possible transfer of an additional SPDS of Holomycota origin to Setaphyta, based on the presence of extra copies in the genomes of the bryophyte Sphagnum and of several liverworts that unequivocally cluster with some sequences of that class (Supplementary Files S1–S3). Fourth, as proposed in previous analyses (Minguet et al., 2008; Takano et al., 2012), our more extensive search still identifies ACL5 orthologs only in plants, red algae, diatoms, archaea and bacteria (Figures 1A,B). The new data are compatible with the proposed model that TSPMS activity in Archaeplastida has a prokaryotic origin, while the common root with the Archaea Sulfolobus and Pyrobaculum, of two additional copies of ACL5 orthologs in Thalassiosira pseudonana suggests a possible second event of horizontal gene transfer to Chromista (Figure 1B). And fourth, there are indications of an early ACL5 duplication in Spermatophyta, followed by several species-specific losses of one of the copies within the angiosperms (some Brassicaceae like Arabidopsis thaliana, some Poaceae) (Figure 1C).
Thermospermine Is Synthesized Across All Plant Lineages
Our phylogenetic analyses identified at least one putative ACL5 ortholog in all studied non-vascular plant species (Figure 1C). Since the only confirmed function for Tspm in plants is the regulation of xylem maturation dynamics (which, by definition, does not occur in non-vascular plants), we wondered whether the identified sequences encode enzymes displaying actual TSPMS activity. Therefore, we expressed the ACL5 homologs of representative plant lineages in yeast, an organism that is unable to produce Tspm. Among the non-vascular plants, we tested ACL5 homologs of a chlorophyte (Chlamydomonas reinhardtii), a liverwort (Marchantia polymorpha) and a moss (Physcomitrella patens (Supplementary Table S1). As representative vascular plants we chose one lycophyte (Selaginella lepidophylla), and one gymnosperm (Picea abies), with the angiosperm A. thaliana as a control (Supplementary Table S1). As previously shown, HPLC analysis showed the presence of Put, Spd and Spm, but not Tspm, in the extracts of a wild-type yeast strain transformed with an empty plasmid (Figure 2A). In contrast, all the tested ACL5 homologs allowed the production of Tspm in yeast (Figures 2B–G) demonstrating that these genes encode enzymes with TSPMS activity. This is in accordance with the previously reported partial complementation of the Arabidopsis acl5 mutant by one of the PpACL5 orthologs (Takahashi and Kakehi, 2010). The observed differences in Tspm production between the different ACL5 genes suggest either species-specific variation in TSPMS activity kinetic parameters or in the variable capacity of yeast to express the different heterologous genes.
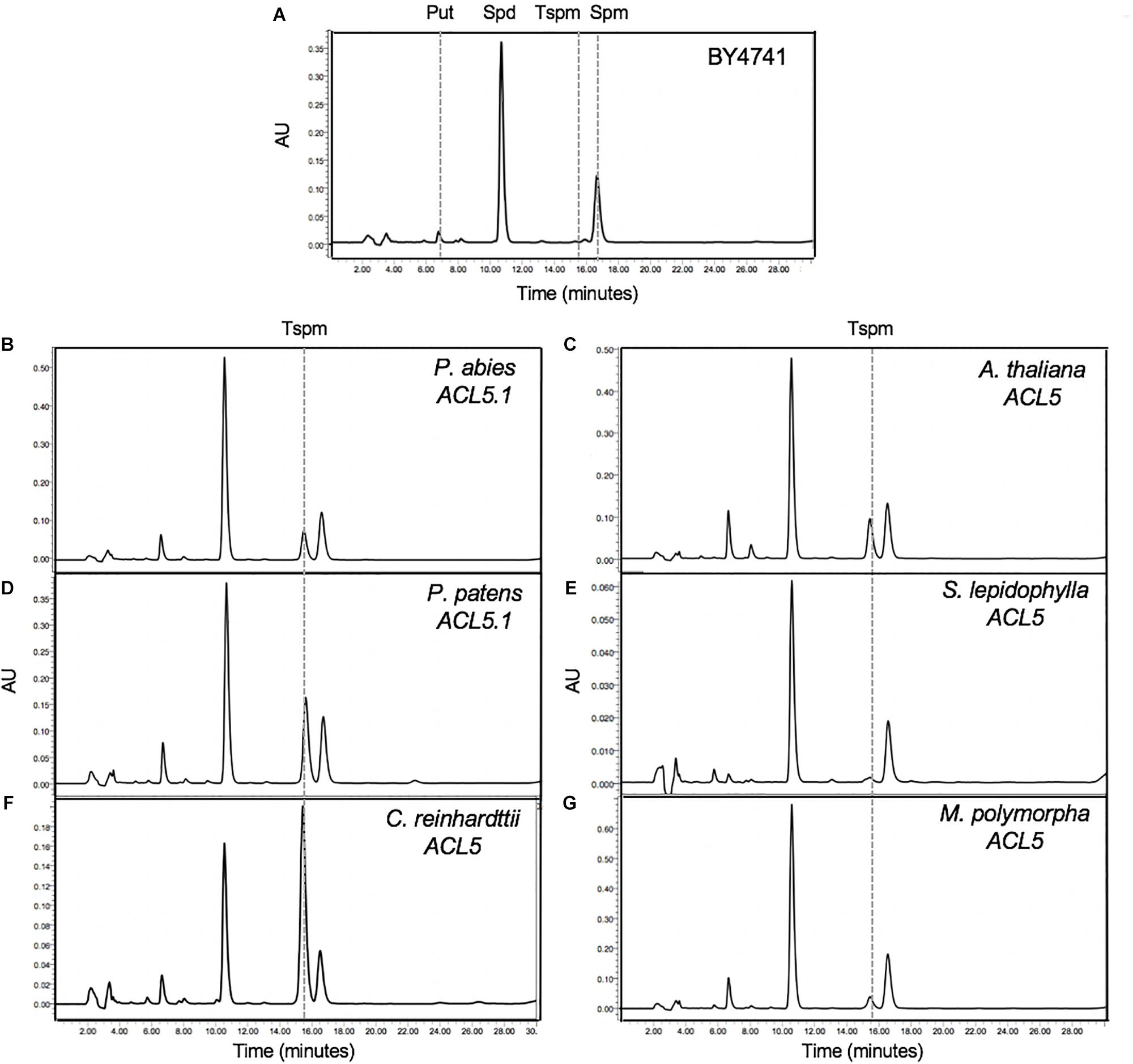
Figure 2. Functional analysis of ACL5 orthologs. Saccharomyces cerevisiae BY4741 strain, unable to produce Tspm (A), was transformed with the putative ACL5 from P. abies (B), A. thaliana (C), P. patens (D), S. lepidophylla ACL5.1 (E), C. reinhardtii (F) and M. polymorpha (G), and the ability to produce Tspm was tested with an HPLC analysis. Graphs show the polyamine profile of yeast extracts after benzoylation and fluorescence detection with the help of a fluorimeter. Tspm, thermospermine; AU, absorbance units.
To check whether the presence of ACL5 orthologs with TSPMS activity correlated with the ability of these species to synthesize Tspm in vivo, we examined the polyamine levels in samples of these plants grown in standard conditions (see Section “Materials and Methods”). As expected, all vascular and non-vascular species accumulated Put and Spd to different levels (Table 1). For instance, Put levels in the chlorophyte C. reinhardtii were between one and three orders of magnitude higher than in the land plants examined, while Spd levels were generally higher in the land plants than in C. reinhardtii, except for P. patens. On the other hand, Spm was detectable in A. thaliana and P. abies, in agreement with previous reports for the occurrence of this tetraamine in seed plants (Gonzalez et al., 2011; Vuosku et al., 2018) and the presence of SPMS orthologs in this clade (Figure 1A). The detection of Spm in S. lepidophylla despite the absence of SPMS orthologs in lycophytes indicates that this tetra-amine might be synthesized by a less strict SPDS, as already suggested for P. sylvestris (Vuosku et al., 2018). With respect to Tspm, all the species tested were able to synthesize this polyamine at different levels, varying between 1.13 nM/g (fresh weight) in C. reinhardtii and 154 nM/g in P. abies. It is worth noting that, while previous reports show that Tspm tends to accumulate less than Spm in Arabidopsis (Naka et al., 2010; Rambla et al., 2010; Cai et al., 2016; Yoshimoto et al., 2016), in the species, tissues and conditions analyzed here, the two polyamines showed comparable levels. Although this result does not demonstrate that the ACL5 orthologs identified in all the selected species are responsible for the Tspm synthesis shown here, this possibility is further supported by the observation that these genes are actually expressed in the same growth conditions as the ones used for Tspm quantification (Figure 3).
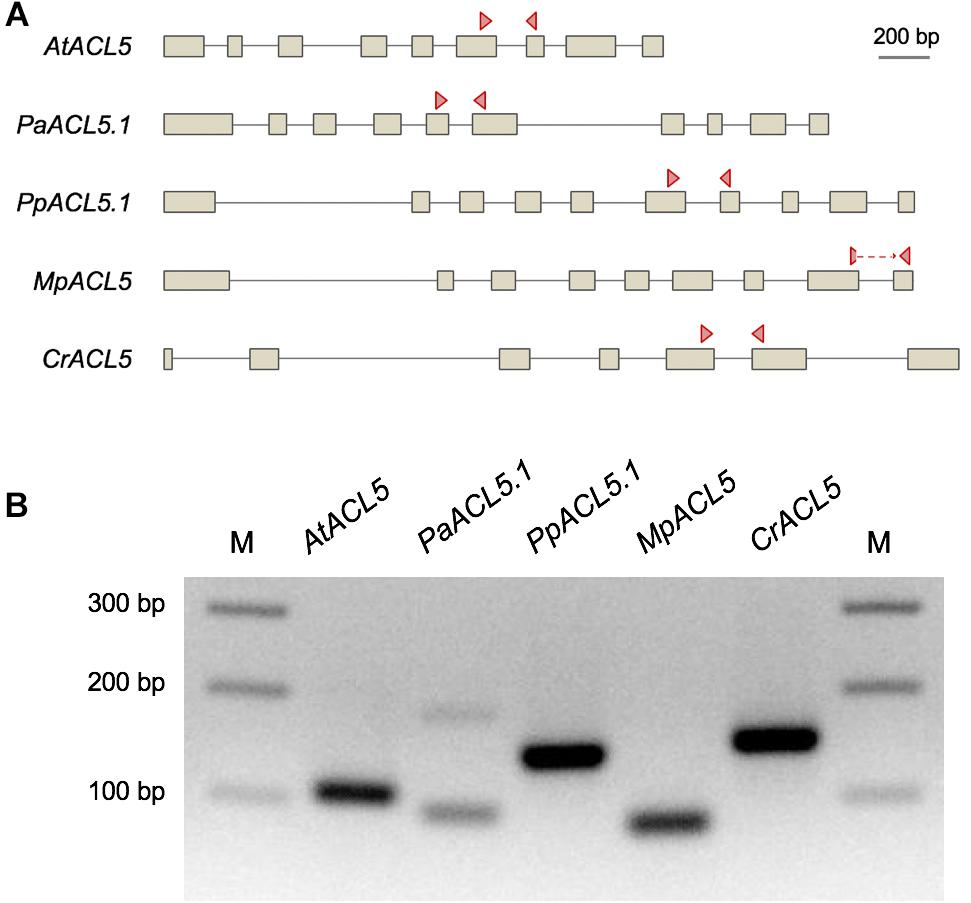
Figure 3. Detection of the expression of ACL5 homologs in several plant species by PCR. (A) Schemes of the genes selected for this analysis. Exons are represented as boxes, and arrowheads indicate the primer pairs used for PCR detection, which are located on different exons, except for MpACL5, in which one of them spans an exon-exon junction. (B) PCR products amplified from cDNA. Plant material for RNA extraction and later cDNA synthesis was grown in the same standard conditions than the plants used for polyamine quantification by HPLC analysis.
Differential ACL5 Expression Dynamics in Vegetative and Reproductive Organs Throughout Development in Seed Plants
Tspm synthase activity has been associated to xylem development in A. thaliana (Muniz et al., 2008; Vera-Sirera et al., 2015), Populus trichocarpa (Milhinhos et al., 2013) and P. sylvestris (Vuosku et al., 2018). To investigate whether this association can be extended to other vascular plants and to point to additional potential roles in these species, we took advantage of the vast amount of publicly available transcriptomic data and examined the expression pattern of the ACL5 orthologs in vegetative and reproductive organs of several vascular species at different developmental stages.
Although for some species we did not find data for stem or root, the available transcriptomic data shows that, for all studied species, ACL5 transcripts tend to accumulate more in vegetative than in reproductive organs (Figure 4A). In the species for which transcriptomic data exists for both stem and root, ACL5 expression is mostly higher in stems than in roots (7 out of 13 of the ACL5 homologs), while only in two cases is the opposite behavior observed. In Medicago truncatula, where two ACL5 paralogs exist, we found that one of them is more prominently accumulated in the stem, while the other one accumulates more in the root. Since our analyses are based on percentage of transcript accumulation across organs, in the species for which no transcriptomic data is available for stem tissue (i.e., Solanum lycopersicum), the proportion of transcript accumulation appears to be highly enriched in roots. For the same reason, in general, we regard the cases in which we found strong ACL5 accumulation in flowers to the non-availability of expression data for stem or root (i.e., Oryza sativa or Glycine max ACL5#4). Only in the case of Vitis vinifera (where transcriptomic data was available for all tested organs) we found that one of the paralogs showed a (slight) preferential expression in flowers than in vegetative organs. In P. trichocarpa, where three paralogs exist, we found that none of them display preferential expression for any of the analyzed organs.
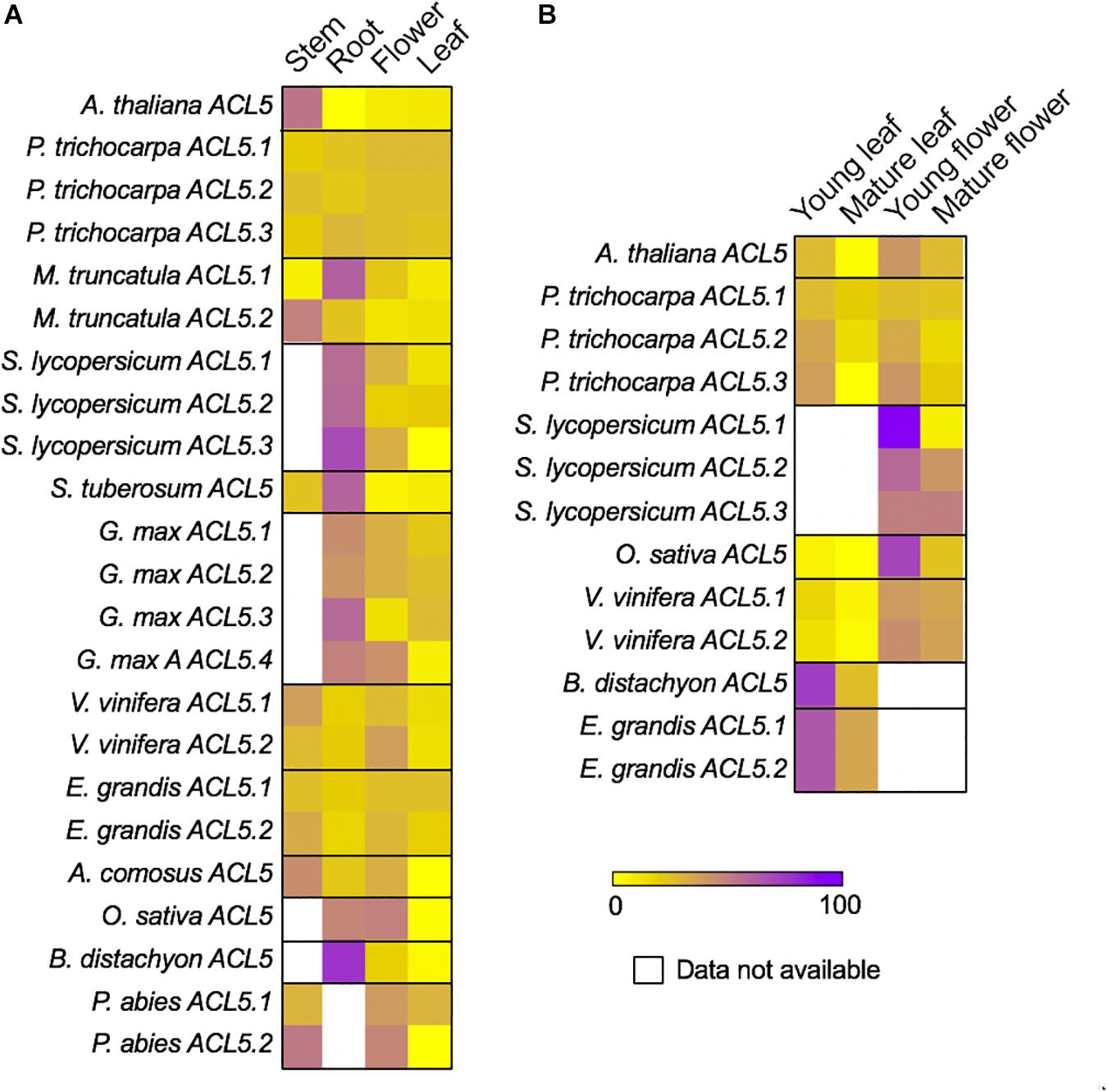
Figure 4. Differential expression of TSPMS genes in representative seed plants. Relative expression data of TSPMS is shown as percentage of expression of each gene per species. (A) Expression data of TSPMS in A. thaliana, P. trichocarpa, M. truncatula, S. lycopersicum, S. tuberosum, G. max, V. vinifera, E. grandis, A. comosus, O. sativa, B. distachyon and P. abies in stem, root, flower and leaf. (B) Expression data of TSPMS in from A. thaliana, P. trichocarpa, S. lycopersicum, O. sativa, V. vinifera, B. distachyon and E. grandis in different developmental stages of leaf and flower.
We also observed a marked preference of ACL5 expression for young vs. mature organs (Figure 4B). In A. thaliana, P. trichocarpa, O. sativa and V. vinifera, this was visible in both flowers and leaves. There was no preferential expression between leaves and flowers except for the case of V. vinifera, in which both paralogs displayed higher preference for flowers than for leaves. For S. lycopersicum, we only found transcriptomes for young and mature flowers, while in Brachypodium distachyon and Eucalyptus grandis, we only found transcriptomes for young and mature leaves, and in all of them we found enriched ACL5 expression in young organs. These results indicate a fairly widespread expression of ACL5 homologs across organs, and mostly in young tissues with active vasculature development.
Discussion
Our current knowledge about thermospermine is that its only confirmed function in plants is the coordination of xylem maturation. However, previous phylogenetic work reported the existence in the genomes of non-vascular plants of sequences clustering with TSPMS (Minguet et al., 2008; Pegg and Michael, 2010), implying that Tspm might exist and, perhaps, play developmental and/or stress response-related roles also in such species. On one hand, as mentioned above, sequence similarity between all polyamine biosynthesis enzymes – especially those using putrescine or spermidine as substrates- has been extensively reported (Hashimoto et al., 1998; Panicot et al., 2002; Teuber et al., 2007). On the other hand, the sequencing of large numbers of genomes and transcriptomes of non-vascular plant species is relatively recent, and therefore previously published comparative analyses missed key clades in plant phylogeny.
It has been proposed that TSPMS had appeared in plants by endosymbiosis of a cyanobacterium (Minguet et al., 2008). Our data are compatible with three alternative hypotheses in this respect (Figure 5). The most parsimonious one is that TSPMS was already present before Eubacteria and Archaea and was lost in the major eukaryotic branch (Figure 5C). A second loss would have occurred during the divergence of Glaucophyta, explaining the absence in such branch and the presence in the Chromista, Rhodophyta and Viridiplantae branches. A less parsimonious, but plausible possibility is that TSPMS was lost in the last eukaryotic common ancestor (LECA) and that it then was transferred either from Eubacteria or from Archaea (i) to Archaeplastida after Glaucophyta had diverged and (ii) to Chromista (Figure 5B). A third, less parsimonious hypothesis would postulate that TSPMS was lost in LECA and transferred either from Eubacteria or from Archaea to the early lineage of Archaeplastida and to Chromista (Figure 5A). TSPMS would have been lost again in Glaucophyta. However, phylogenetic positioning of TSPMS points to the horizontal transfer of TSPMS genes from Rhodophyta to diatoms (and possibly other algal groups originated during secondary chloroplast acquisition), thus excluding this latter hypothesis, which is also based in a very low sampling among Chromista representatives. Given that it is almost practically impossible to differentiate between the two remaining models, the hypothesis that contemplates only one loss and two reported horizontal transfers seems the most likely one (Figure 5B).
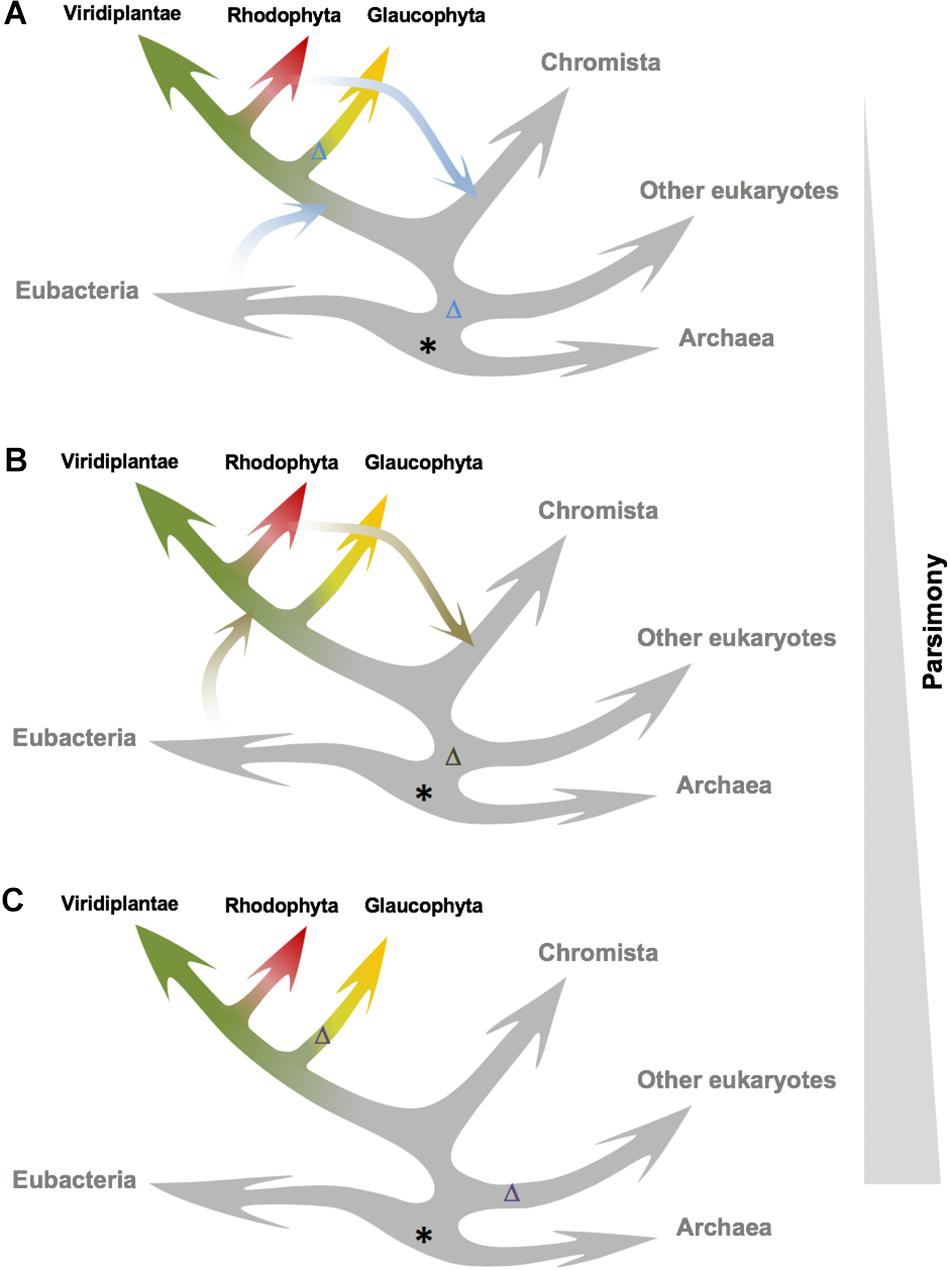
Figure 5. Alternative models for TSPMS activity origin and evolution in plants. The three models are ordered by increasing parsimony. (A) The ancestor of TSPMS activity in LUCA (possibly with a different activity) is lost in LECA; then it is acquired by Archaeplastida via HGT, and lost again in Glaucophyta. Chromista acquire it through secondary endosymbiosis. (B) Similar to the previous one, but Archaeplastida acquire the ACL5 ortholog after divergence of Glaucophyta. (C) The ancestor of TSPMS activity in LUCA is lost early in major eukaryotic clades, after the divergence of the green lineage, followed by an additional loss in Glaucophyta. Putative origin of TSPMS genes is marked with an asterisk, and predicted loss of TSPMS genes is marked with a Δ.
The identification of TSPMS activity in non-vascular plants clearly argues for possible functions of Tspm other than in xylem development. It has been proposed that Tspm might play a role in defense against stress conditions (Gonzalez et al., 2011; Marina et al., 2013), so it could also be the case that such activity is conserved across vascular and non-vascular plants and that developmental functions – either related with vascular development or with other, yet to be identified, processes – were only acquired in vascular plant lineages. In any case, further experimentation using mutant, overexpression and marker lines in emerging non-vascular model plant species such as M. polymorpha or P. patens might provide further information about what developmental and/or stress related processes might be controlled by this polyamine.
The in silico analyses of relative abundance of ACL5 transcripts in different organs in representative seed-plant species presented here aimed at carrying out a first approach toward understanding whether, apart from coordinating xylem maturation, TSPM plays any other role in vascular plants. The higher prevalence of ACL5 transcripts in vegetative than in reproductive organs and in young (presumably developing) than in mature organs argues for higher association of ACL5 expression in organs developing more proportion of vascular tissues (vegetative) at early developmental stages, when vasculature is probably developing. However, the presence of ACL5 transcripts in reproductive organs and in mature vegetative organs also argues for potential other (maybe less prominent) activities of TSPM in plants.
In summary, our work shows that Tspm exists in non-vascular plants and that there is a correlation between Tspm presence, TSPMS activity and ACL5 expression throughout vascular and non-vascular plant lineages. Our results indicate that Tspm might play developmental and/or stress-related roles (apart from the described role in xylem development) not only in non-vascular but also in vascular plants. Future experimentation will shed new light on such intriguing topic.
Data Availability
All datasets generated for this study are included in the manuscript and/or the Supplementary Files.
Author Contributions
AS-G, JH-G, MB, and JA conceived and designed the work. AS-G, JH-G, and ML-G performed all in silico and experimental analyses. JA and MB wrote the first draft of the manuscript, to which all authors contributed.
Funding
This work in the laboratories was funded by grants BFU2016-80621-P and BIO2016-79147-R of the Spanish Ministry of Economy, Industry and Competitiveness. AS-G and JH-G are recipients of Fellowships of the Spanish Ministry of Science, Innovation and Universities BES-2017-080387 and of the Spanish Ministry of Education, Culture and Sport FPU15/01756, respectively. JA holds a Ramón y Cajal Contract RYC-2014-15752.
Conflict of Interest Statement
The authors declare that the research was conducted in the absence of any commercial or financial relationships that could be construed as a potential conflict of interest.
Acknowledgments
We thank the members of the Hormone Signaling and Plasticity Lab at IBMCP (http://plasticity.ibmcp.csic.es/) for useful discussions and suggestions. We acknowledge Federico Valverde (IBVF-Sevilla, Spain), Jesús Vicente Carbajosa (CBGP-Madrid, Spain) and Ramón Serrano (IBMCP-Valencia, Spain) for sharing C. reinhardttii, P. patens and yeast materials, respectively.
Supplementary Material
The Supplementary Material for this article can be found online at: https://www.frontiersin.org/articles/10.3389/fpls.2019.00663/full#supplementary-material
Footnotes
- ^ http://www.ncbi.nlm.nih.gov
- ^ http://www.phytozome.net
- ^ http://db.cngb.org/onekp/
- ^ https://benchling.com
- ^ http://tree.bio.ed.ac.uk/software/figtree/
- ^ http://bar.toronto.ca
- ^ https://matrix2png.msl.ubc.ca/
References
Ashton, N. W., and Cove, D. J. (1977). The isolation and preliminary characterization of auxotrophic and analoguie-resistant mutants of the moss, Physcomitrella patens. Mol. Gen. Genet. 154, 87–95.
Baima, S., Forte, V., Possenti, M., Penalosa, A., Leoni, G., Salvi, S., et al. (2014). Negative feedback regulation of auxin signaling by ATHB8/ACL5-BUD2 transcription module. Mol. Plant 7, 1006–1025. doi: 10.1093/mp/ssu051
Cai, Q., Fukushima, H., Yamamoto, M., Ishii, N., Sakamoto, T., Kurata, T., et al. (2016). The SAC51 family plays a central role in thermospermine responses in Arabidopsis. Plant Cell Physiol. 57, 1583–1592. doi: 10.1093/pcp/pcw113
Chen, D., Shao, Q., Yin, L., Younis, A., and Zheng, B. (2018). Polyamine function in plants: metabolism, regulation on development, and roles in abiotic stress responses. Front. Plant Sci. 9:1945. doi: 10.3389/fpls.2018.01945
Clay, N. K., and Nelson, T. (2005). Arabidopsis thickvein mutation affects vein thickness and organ vascularization, and resides in a provascular cell-specific spermine synthase involved in vein definition and in polar auxin transport. Plant Physiol. 138, 767–777. doi: 10.1104/pp.104.055756
Darriba, D., Taboada, G. L., Doallo, R., and Posada, D. (2011). ProtTest 3: fast selection of best-fit models of protein evolution. Bioinformatics 27, 1164–1165. doi: 10.1093/bioinformatics/btr088
De Rybel, B., Adibi, M., Breda, A. S., Wendrich, J. R., Smit, M. E., Novak, O., et al. (2014). Plant development. Integration of growth and patterning during vascular tissue formation in Arabidopsis. Science 345:1255215. doi: 10.1126/science.1255215
De Rybel, B., Moller, B., Yoshida, S., Grabowicz, I., Barbier de Reuille, P., Boeren, S., et al. (2013). A bHLH complex controls embryonic vascular tissue establishment and indeterminate growth in Arabidopsis. Dev. Cell 24, 426–437. doi: 10.1016/j.devcel.2012.12.013
Gonzalez, M. E., Marco, F., Minguet, E. G., Carrasco-Sorli, P., Blazquez, M. A., Carbonell, J., et al. (2011). Perturbation of spermine synthase gene expression and transcript profiling provide new insights on the role of the tetraamine spermine in Arabidopsis defense against Pseudomonas viridiflava. Plant Physiol. 156, 2266–2277. doi: 10.1104/pp.110.171413
Gouy, M., Guindon, S., and Gascuel, O. (2010). SeaView version 4: a multiplatform graphical user interface for sequence alignment and phylogenetic tree building. Mol. Biol. Evol. 27, 221–224. doi: 10.1093/molbev/msp259
Guindon, S., Dufayard, J. F., Lefort, V., Anisimova, M., Hordijk, W., and Gascuel, O. (2010). New algorithms and methods to estimate maximum-likelihood phylogenies: assessing the performance of PhyML 3.0. Syst. Biol. 59, 307–321. doi: 10.1093/sysbio/syq010
Hanfrey, C., Elliott, K. A., Franceschetti, M., Mayer, M. J., Illingworth, C., and Michael, A. J. (2005). A dual upstream open reading frame-based autoregulatory circuit controlling polyamine-responsive translation. J. Biol. Chem. 280, 39229–39237. doi: 10.1074/jbc.M509340200
Hanfrey, C., Franceschetti, M., Mayer, M. J., Illingworth, C., and Michael, A. J. (2002). Abrogation of upstream open reading frame-mediated translational control of a plant S-adenosylmethionine decarboxylase results in polyamine disruption and growth perturbations. J. Biol. Chem. 277, 44131–44139. doi: 10.1074/jbc.M206161200
Hanzawa, Y., Takahashi, T., and Komeda, Y. (1997). ACL5: an Arabidopsis gene required for internodal elongation after flowering. Plant J. 12, 863–874.
Hanzawa, Y., Takahashi, T., Michael, A. J., Burtin, D., Long, D., Pineiro, M., et al. (2000). ACAULIS5, an Arabidopsis gene required for stem elongation, encodes a spermine synthase. EMBO J. 19, 4248–4256. doi: 10.1093/emboj/19.16.4248
Hashimoto, T., Tamaki, K., Suzuki, K., and Yamada, Y. (1998). Molecular cloning of plant spermidine synthases. Plant Cell Physiol. 39, 73–79.
Imai, A., Hanzawa, Y., Komura, M., Yamamoto, K. T., Komeda, Y., and Takahashi, T. (2006). The dwarf phenotype of the Arabidopsis acl5 mutant is suppressed by a mutation in an upstream ORF of a bHLH gene. Development 133, 3575–3585.
Imai, A., Matsuyama, T., Hanzawa, Y., Akiyama, T., Tamaoki, M., Saji, H., et al. (2004). Spermidine synthase genes are essential for survival of Arabidopsis. Plant Physiol. 135, 1565–1573. doi: 10.1104/pp.104.041699
Kakehi, J. I., Kuwashiro, Y., Niitsu, M., and Takahashi, T. (2008). Thermospermine is required for stem elongation in Arabidopsis thaliana. Plant Cell Physiol. 49, 1342–1349. doi: 10.1093/pcp/pcn109
Katayama, H., Iwamoto, K., Kariya, Y., Asakawa, T., Kan, T., Fukuda, H., et al. (2015). A negative feedback loop controlling bHLH complexes is involved in vascular cell division and differentiation in the root apical meristem. Curr. Biol. 25, 3144–3150. doi: 10.1016/j.cub.2015.10.051
Knott, J. M., Romer, P., and Sumper, M. (2007). Putative spermine synthases from Thalassiosira pseudonana and Arabidopsis thaliana synthesize thermospermine rather than spermine. FEBS Lett. 581, 3081–3086.
Marina, M., Sirera, F. V., Rambla, J. L., Gonzalez, M. E., Blazquez, M. A., Carbonell, J., et al. (2013). Thermospermine catabolism increases Arabidopsis thaliana resistance to Pseudomonas viridiflava. J. Exp. Bot. 64, 1393–1402. doi: 10.1093/jxb/ert012
Michael, A. J. (2016). Polyamines in eukaryotes, bacteria, and archaea. J. Biol. Chem. 291, 14896–14903. doi: 10.1074/jbc.R116.734780
Milhinhos, A., Prestele, J., Bollhoner, B., Matos, A., Vera-Sirera, F., Rambla, J. L., et al. (2013). Thermospermine levels are controlled by an auxin-dependent feedback loop mechanism in populus xylem. Plant J. 75, 685–698. doi: 10.1111/tpj.12231
Minguet, E. G., Vera-Sirera, F., Marina, A., Carbonell, J., and Blazquez, M. A. (2008). Evolutionary diversification in polyamine biosynthesis. Mol. Biol. Evol. 25, 2119–2128. doi: 10.1093/molbev/msn161
Muniz, L., Minguet, E. G., Singh, S. K., Pesquet, E., Vera-Sirera, F., Moreau-Courtois, C. L., et al. (2008). ACAULIS5 controls Arabidopsis xylem specification through the prevention of premature cell death. Development 135, 2573–2582. doi: 10.1242/dev.019349
Naka, Y., Watanabe, K., Sagor, G. H., Niitsu, M., Pillai, M. A., Kusano, T., et al. (2010). Quantitative analysis of plant polyamines including thermospermine during growth and salinity stress. Plant Physiol. Biochem. 48, 527–533. doi: 10.1016/j.plaphy.2010.01.013
Panicot, M., Minguet, E. G., Ferrando, A., Alcazar, R., Blazquez, M. A., Carbonell, J., et al. (2002). A polyamine metabolon involving aminopropyl transferase complexes in Arabidopsis. Plant Cell 14, 2539–2551.
Pegg, A. E., and Michael, A. J. (2010). Spermine synthase. Cell. Mol. Life Sci. 67, 113–121. doi: 10.1007/s00018-009-0165-5
Rambla, J. L., Vera-Sirera, F., Blázquez, M. A., Carbonell, J., and Granell, A. (2010). Quantitation of biogenic tetraamines in Arabidopsis thaliana. Anal. Biochem. 397, 208–211. doi: 10.1016/j.ab.2009.10.013
Sagor, G. H., Berberich, T., Takahashi, Y., Niitsu, M., and Kusano, T. (2013). The polyamine spermine protects Arabidopsis from heat stress-induced damage by increasing expression of heat shock-related genes. Transgenic Res. 22, 595–605. doi: 10.1007/s11248-012-9666-3
Sarrion-Perdigones, A., Falconi, E. E., Zandalinas, S. I., Juarez, P., Fernandez-del-Carmen, A., Granell, A., et al. (2011). GoldenBraid: an iterative cloning system for standardized assembly of reusable genetic modules. PLoS One 6:e21622. doi: 10.1371/journal.pone.0021622
Sekula, B., and Dauter, Z. (2018). Crystal structure of thermospermine synthase from Medicago truncatula and substrate discriminatory features of plant aminopropyltransferases. Biochem. J. 475, 787–802. doi: 10.1042/bcj20170900
Siebers, T., Catarino, B., and Agusti, J. (2017). Identification and expression analyses of new potential regulators of xylem development and cambium activity in cassava (Manihot esculenta). Planta 245, 539–548. doi: 10.1007/s00425-016-2623-2
Tabor, C. W., and Tabor, H. (1984). Polyamines. Annu. Rev. Biochem. 53, 749–790. doi: 10.1146/annurev.bi.53.070184.003533
Takahashi, T., and Kakehi, J. (2010). Polyamines: ubiquitous polycations with unique roles in growth and stress responses. Ann. Bot. 105, 1–6. doi: 10.1093/aob/mcp259
Takano, A., Kakehi, J., and Takahashi, T. (2012). Thermospermine is not a minor polyamine in the plant kingdom. Plant Cell Physiol. 53, 606–616. doi: 10.1093/pcp/pcs019
Teuber, M., Azemi, M. E., Namjoyan, F., Meier, A. C., Wodak, A., Brandt, W., et al. (2007). Putrescine N-methyltransferases–a structure-function analysis. Plant Mol. Biol. 63, 787–801. doi: 10.1007/s11103-006-9126-7
Vera-Sirera, F., De Rybel, B., Urbez, C., Kouklas, E., Pesquera, M., Alvarez-Mahecha, J. C., et al. (2015). A bHLH-based feedback loop restricts vascular cell proliferation in plants. Dev. Cell 35, 432–443. doi: 10.1016/j.devcel.2015.10.022
Vera-Sirera, F., Minguet, E. G., Singh, S. K., Ljung, K., Tuominen, H., Blazquez, M. A., et al. (2010). Role of polyamines in plant vascular development. Plant Physiol. Biochem. 48, 534–539. doi: 10.1016/j.plaphy.2010.01.011
Vuosku, J., Karppinen, K., Muilu-Makela, R., Kusano, T., Sagor, G. H. M., Avia, K., et al. (2018). Scots pine aminopropyltransferases shed new light on evolution of the polyamine biosynthesis pathway in seed plants. Ann. Bot. 121, 1243–1256. doi: 10.1093/aob/mcy012
Wu, H., Min, J., Ikeguchi, Y., Zeng, H., Dong, A., Loppnau, P., et al. (2007). Structure and mechanism of spermidine synthases. Biochemistry 46, 8331–8339. doi: 10.1021/bi602498k
Yamaguchi, K., Takahashi, Y., Berberich, T., Imai, A., Miyazaki, A., Takahashi, T., et al. (2006). The polyamine spermine protects against high salt stress in Arabidopsis thaliana. FEBS Lett. 580, 6783–6788. doi: 10.1016/j.febslet.2006.10.078
Yamaguchi, K., Takahashi, Y., Berberich, T., Imai, A., Takahashi, T., Michael, A. J., et al. (2007). A protective role for the polyamine spermine against drought stress in Arabidopsis. Biochem. Biophys. Res. Commun. 352, 486–490. doi: 10.1016/j.bbrc.2006.11.041
Keywords: plants, polyamines, thermospermine, evolution, development
Citation: Solé-Gil A, Hernández-García J, López-Gresa MP, Blázquez MA and Agustí J (2019) Conservation of Thermospermine Synthase Activity in Vascular and Non-vascular Plants. Front. Plant Sci. 10:663. doi: 10.3389/fpls.2019.00663
Received: 08 March 2019; Accepted: 02 May 2019;
Published: 11 June 2019.
Edited by:
Rubén Alcázar, University of Barcelona, SpainReviewed by:
Thomas Berberich, Senckenberg Nature Research Society, GermanyTaku Takahashi, Okayama University, Japan
Copyright © 2019 Solé-Gil, Hernández-García, López-Gresa, Blázquez and Agustí. This is an open-access article distributed under the terms of the Creative Commons Attribution License (CC BY). The use, distribution or reproduction in other forums is permitted, provided the original author(s) and the copyright owner(s) are credited and that the original publication in this journal is cited, in accordance with accepted academic practice. No use, distribution or reproduction is permitted which does not comply with these terms.
*Correspondence: Javier Agustí, jagusti@ibmcp.upv.es