- 1Shandong Provincial Key Laboratory of Plant Stress, College of Life Sciences, Shandong Normal University, Jinan, China
- 2State Key Laboratory of Crop Biology, College of Life Sciences, Shandong Agricultural University, Tai’an, China
- 3College of Marine Life Sciences, and Frontiers Science Center for Deep Ocean Multispheres and Earth System, Ocean University of China, Qingdao, China
- 4Institute of Integrative Biology, University of Liverpool, Liverpool, United Kingdom
Cyanobacteria are autotrophs whose photosynthetic process is similar to that of higher plants, although the photosynthetic apparatus is slightly different. They have been widely used for decades as model systems for studying the principles of photosynthesis, especially the effects of environmental stress on photosynthetic activities. Salt stress, which is the most common abiotic stress in nature, combines ionic and osmotic stresses. High cellular ion concentrations and osmotic stress can alter normal metabolic processes and photosynthesis. Additionally, salt stress increases the intracellular reactive oxygen species (ROS) contents. Excessive amounts of ROS will damage the photosynthetic apparatus, inhibit the synthesis of photosystem-related proteins, including the D1 protein, and destroy the thylakoid membrane structure, leading to inhibited photosynthesis. In this review, we mainly introduce the effects of salt stress on the cyanobacterial membranes and photosynthetic apparatus. We also describe specific salt tolerance mechanisms. A thorough characterization of the responses of membranes and photosynthetic apparatus to salt stress may be relevant for increasing agricultural productivity.
Introduction
Salt stress is an abiotic factor that greatly influences plant survival and development. There are currently more than 1.2 billion hectares of land affected by salt, accounting for about 6% of the total global land area (Wicke et al., 2011; Yuan et al., 2016; Sui et al., 2018). Annual worldwide economic losses due to salt stress can exceed US$12 billion (Shabala, 2013; Song and Wang, 2014). Therefore, improving salt tolerance of agriculturally important plants is critical.
Cyanobacteria have a photosynthetic system similar to that of higher plants, and their cytoplasm and thylakoid membranes are similar to those of the chloroplast of higher plants in lipid composition and membrane assembly generally (Rodriguez-Ezpeleta et al., 2005; Los et al., 2010). Therefore, cyanobacteria can be used as a model system to study the mechanisms of photosynthesis, membrane lipids and signal transduction under abiotic stress (Los and Murata, 2004; Jensen and Leister, 2014), which may also provide an applicable model for higher plants. To survive in extreme or variable environment, cyanobacteria have evolved specific metabolic mechanisms and regulatory systems (Tandeau de Marsac and Houmard, 1993). For example, Synechocystis sp. PCC6803 (hereafter referred to as Synechocystis 6803), a unicellular freshwater cyanobacterium, can tolerate up to 1.2 M NaCl (Desplats et al., 2005). The photosynthetic apparatus of Synechocystis 6803 are similar to those of higher plants and are easier to separate (Öquist et al., 1995; Allakhverdiev and Murata, 2008). And compared with higher plants, they have relatively simple genetic systems (Haselkorn, 1991). In cyanobacteria, the thylakoid membrane is the only site of photosynthesis and also the main site of respiratory electron transport in cells (Mullineaux, 2014). The growth cycle of cyanobacteria is short, making it is easy to be treated with various salt concentrations, and there are relatively few other interfering factors. These characteristics have made cyanobacteria the ideal model system for studying the photosynthetic responses to salt stress.
High NaCl concentrations are toxic to cells, ultimately resulting in a damaged photosynthetic apparatus (Figure 1). In cells of photosynthetic organisms, salt stress leads to a decrease in cell volume and osmotic stress, it also inhibits the photosynthetic electron transfer process (Allakhverdiev and Murata, 2008; Feng et al., 2014; Zhao et al., 2019). 0.5 M NaCl inactivated both PSII and PSI in Synechococcus cells due to the changes in K/Na ratio (Allakhverdiev et al., 2000). In spirulina platensis, salt stress leads to a reduction in PSII electron transport by increasing the number of QB-non-reduction reaction centers (Lu and Vonshak, 1999). After that, it was be found that salt stress did not directly affect the PSII activity itself in the dark, but the same salt stress combined with photosynthesis effective radiation blocked electron transport between QA and QB (primary and secondary quinone electron acceptors of PSII located in proteins D2 and D1, respectively), and the degree of inhibition was proportional to the intensity of light (Lu and Zhang, 2000; Lu and Vonshak, 2002). Sudhir et al. (2005) found that NaCl not only inhibited PSII activity by inhibiting the D1 protein, but also decreased energy transfer from light harvesting antenna to PSII by changing other thylakoid membrane proteins such as 47 kDa chlorophyll protein (CP) and 94 kDa protein in Arthrospira platensis. Intracellular sodium and potassium homeostasis is important for maintaining the normal activity of enzymes (Hu et al., 2016; Chen et al., 2017; Ma et al., 2019), cell membrane potential (Zhang et al., 2014; Wei et al., 2017), and normal cell volume (Serrano and Rodrigueznavarro, 2001; Zhu, 2003; Shao et al., 2014). The low concentration of Na+ helps to regulate the pH in plants and cyanobacteria, as well as the fixation of nitrogen and carbon dioxide (Karandashova and Elanskaya, 2005; Han et al., 2012; Fan et al., 2019). Excessive Na+ and Cl– flows into the cell disrupted ion homeostasis, leading to accumulation of reactive oxygen species (ROS) (Scarpeci et al., 2008; Feng et al., 2015; Song et al., 2020). High ROS levels are toxic to cells, with the associated destruction of the photosynthetic apparatus and membrane lipid peroxidation (Zhang et al., 2012; Sui and Han, 2014; Yang et al., 2020), adversely affecting photosynthesis. Additionally, high NaCl concentrations inhibit the de novo synthesis of proteins, including many photosystem-related proteins, such as the D1 protein (Allakhverdiev et al., 2002). For example, ROS produced in cells under salt stress could inhibit transcription factor activities, resulting in downregulated psbA expression (Jimbo et al., 2018).
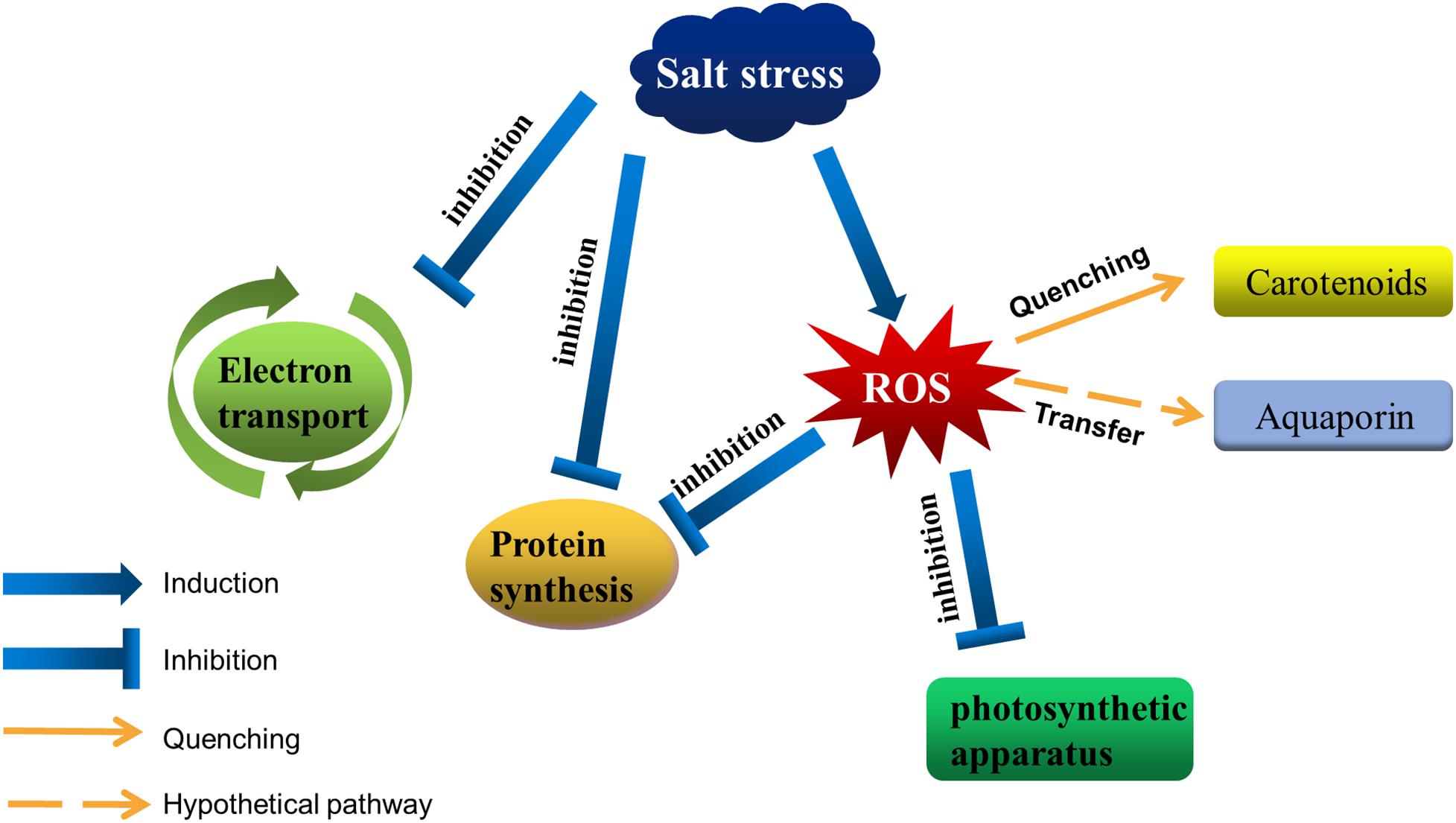
Figure 1. Salt stress affects photosynthetic activities in cyanobacteria. Salt stress inhibits the electron transfer around the photosystem, as well as protein synthesis. Moreover, it disrupts intracellular ion homeostasis, resulting in the accumulation of ROS in cells. Excessive ROS destroys the photosynthetic apparatus and inhibits protein synthesis. Cyanobacteria can decrease the amount of ROS by accumulating carotenoids, and aquaporins may help transfer ROS from the photosystem to other regions.
Cyanobacteria have evolved diverse physiological mechanisms to cope with salt stress. Cyanobacteria may alter the content of certain membrane proteins in order to repair or resynthesize photosynthetic complexes damaged by salt stress. For instance, salt stress results in the enhancement of the vesicle-inducing protein in plastids 1 (Vipp1) in Synechocystis 6803 (Huang et al., 2006). The Vipp1 have been suggested to be involved in the assembly of the thylakoid membrane system by transferring lipids from the inner envelope of chloroplast (Li et al., 1994; Vothknecht et al., 2012). It has also been found that photosystem I (PSI) and photosystem II (PSII) reaction center cores are originally synthesized in the plasma membrane of cyanobacteria (Zak et al., 2001). Therefore, the increased Vipp1 may help accelerate the transport of the reaction center cores to thylakoid membrane under salt stress (Huang et al., 2006). Marin et al. reported that the acclimation of Synechocystis 6803–684 mM NaCl (4%, w/v) can be divided into two phases: the initial response to salt shock and longer-term adaptation to salt (Marin et al., 2004). In the first phase, the expression levels of many genes are upregulated, whereas in the second stage, the expression levels of only a few of these genes remain upregulated, such as the genes involved in glucosylglycerol (GG) synthesis and those encoding ABC transporters (Marin et al., 2004). Kanesaki et al. revealed that the expression of many genes is induced in response to salt stress, including the genes associated with the D1 protein at the photochemical reaction center of PSII (Kanesaki et al., 2002; Qiao et al., 2013). For instance, the rbp3 gene, which encodes a type II RNA-binding protein, helps maintain the transcript levels of acyl-lipid desaturase genes (desA, desB, and desD) and the substantial abundance of unsaturated membrane lipid (Tang et al., 2010). This review focuses on the effects of salt stress on membrane, the photosystem and photosynthetic activities of cyanobacteria. We also describe the mechanisms in cyanobacteria that facilitate the protection of photosystems from the detrimental effects of salinity stress.
Effects of ROS on Photosynthesis Under Salt Stress
The O2 molecule has two unpaired electrons that have the same spin quantum number (Gill and Tuteja, 2010). Due to its spin restriction, molecular oxygen prefers to receive electrons from high energy level to produce the so called ROS which are toxic to cells at high concentration (Ahanger et al., 2017). ROS includes singlet oxygen (1O2), superoxide anion (O2–), hydrogen peroxide (H2O2), and the hydroxyl radical (OH⋅) etc. In plant, peroxisomes not only destroy ROS due to the activities of peroxidases and catalases, but also produce superoxide radicals and there are, at least, two sites of superoxide generation: one in the organelle matrix, the generating system being xanthine oxidase, and another site in the peroxisomal membranes dependent on NAD(P)H (del RÍo et al., 2002). Thus in plants, chloroplasts and peroxisomes are the main producers of ROS during the day, while mitochondria are the main producers at night (Moller, 2001; Foyer and Noctor, 2003; Gill and Tuteja, 2010). In cyanobacteria, ROS are also mainly derived from photochemical reactions and the photosynthetic electron transport (Latifi et al., 2009; Wada et al., 2013). In the process of photosynthetic electron transfer, ROS are mainly produced at three sites (Asada, 1999): (1) The oxygen-evolving complex in the water photolysis releases ROS due to state III inactivation. (2) The electrons are lost to O2 by PQ on the reducing side of PSII to produce H2O2. (3) The electrons are transferred to O2 directly or by ferredoxin (Fd) to produce O2–. on the reduction side of PSI. Under high light stress, due to the limited acceptors in the respiratory chain, electron leakage leads to the reduction of triplet oxygen (3O2) into O2–. (Mittler, 2002). Studies have shown that cyanobacteria cells under salt stress have a huge demand for ATP synthesis, which may reduce the fixation rate of CO2 and lead to the excessive reduction of ferroredoxin pool (Van Thor et al., 2000; Latifi et al., 2009). This may be one of the reasons why salt stress result in oxidative stress.
Under abiotic stress conditions, singlet chlorophyll is transformed into triplet chlorophyll, which transfers its energy to 3O2 to form 1O2. 1O2 has a short half-life in the cell and reacts with nearby target molecules (proteins, pigments, and lipids) immediately (Gorman and Rodgers, 1992; Latifi et al., 2009). It has been shown that 1O2 and H2O2 can inhibit the translational elongation of psbA mRNA (Nishiyama et al., 2001, 2004). And H2O2 also interrupts the energy transfer between the core and the terminal emitter of phycobilisomes in Synechocystis 6803 (Liu et al., 2005). Excessive ROS will lead to membrane lipid peroxidation, which not only directly affects the normal function of cells, but also aggravates oxidative stress by producing lipid-derived free radicals (Montillet et al., 2005). ROS reacts with large molecules such as phospholipids and enzymes in the membrane to form lipid peroxidation products, resulting in decreased membrane fluidity (Gill and Tuteja, 2010). And ROS damages membrane proteins and ion channels, causing leakiness of some substance.
The scavenging of ROS in cyanobacteria cells is mainly by energy dissipation, antioxidant enzymes and non-enzymatic antioxidants. For instance, energy dissipation can be carried out by the “CP43′ protein”, and this process is induced by iron deficiency in cyanobacteria (Latifi et al., 2009). Cyanobacteria can also dissipate energy through the Mehler-like reaction. Mehler first described the process of reducing O2 to H2O2 by photosynthetic electron transport chain in chloroplast, and this reaction is therefore called the Mehler reaction (Mehler, 1951; Mehler and Brown, 1952). After that, H2O2 is rapidly detoxified to water by the ascorbate peroxidase pathway. In this process, the electrons are split from the water in oxygen releasing complex (OEC) and then flow through the PSI to produce water again. Therefore, it is called the water-water cycle, or “pseudocyclic electron flow” (Asada, 1999, 2000). Compared with plant, there is a similar reaction in cyanobacteria, known as the Mehler-like reaction. This reaction can reduce O2 with electrons mediated by PSI by means of soluble flavoproteins 1 and flavoproteins 3 proteins (Vicente et al., 2002; Helman et al., 2003; Helman et al., 2005; Allahverdiyeva et al., 2011; Allahverdiyeva et al., 2013). It is a four-electron transfer reaction and it does not produce ROS and may protect PSI against production of O2– (Allahverdiyeva et al., 2013). The superoxide dismutase (SOD), most effective intracellular enzymatic antioxidant, can remove O2– by catalyzing its dismutation: one O2– being reduced to H2O2 and another oxidized to O2 (Gill and Tuteja, 2010). Kanesaki et al. demonstrated that salt stress and hyperosmotic stress induced the expression of sodB, which encoded an FeSOD enzyme (Kanesaki et al., 2002). Non-enzymatic antioxidant carotenoids can protect cellular structures, especially the photosystems, from the damage by scavenging 1O2 and excitation energy. Carotenoids are a group of highly reductive substances that can protect cellular structures, especially the photosystems, from the damage by scavenging 1O2 and excitation energy. In photosynthetic organisms, carotene mainly has three functions: harvesting light energy, acting as a light screen, and quenching singlet oxygen (Frank and Cogdell, 1996). In thylakoids, the carotenoids have two functions: harvesting light energy and photoprotection via the quenching of energy and 1O2 (Sedoud et al., 2014). The orange carotenoid protein (OCP) is a soluble blue-green photoactive protein that binds a single keto-carotenoid molecule (Kirilovsky and Kerfeld, 2013, 2016; Dominguez-Martin and Kerfeld, 2019). And salt stress increases its transcripts and proteins levels (Kanesaki et al., 2002; Fulda et al., 2006). In cyanobacteria, OCP interacting with the phycobilisome, increases energy dissipation in the form of heat, thereby decreasing the amount of energy arriving at the reaction centers (Wilson et al., 2006). Kawasaki et al. (2013) found a purified astaxanthin-binding OCP from a eukaryotic microalga, named AstaP, shows high solubility in water and can quench singlet oxygen. And they found that the gene encoding AstaP was significantly up-regulated by salt stress (Kawasaki et al., 2013). A lack of β-carotene prevents cyanobacteria from generating a functional PSII, whereas a lack of almost all xanthophylls resulted in a considerable increase in the intracellular ROS content (Sozer et al., 2010; Zhu et al., 2010). Thus, in response to environmental stress, including salt stress, carotenoids are critical for maintaining the stability of photosynthesis. In addition to the above, aquaporins may also be involved in the scavenging of ROS under salt stress. Some aquaporin isoforms mediate permeation of glycerol, H2O2 or CO2 in addition to water (Maurel, 2007). Some plant aquaporins expressed in yeast can transport H2O2 molecules (Bienert et al., 2007), implying that aquaporins may be able to maintain the stability of photosystems by transferring ROS to other regions. The ΔaqpZ cells of Synechocystis 6803 showed defects in macronutrient metabolism, pH homeostasis, and cell division under photomixotrophic conditions (Akai et al., 2011), but whether the above mechanism applies to cyanobacteria remains further experimental exploration.
In some cyanobacteria such as PCC6803 which contain polyunsaturated fatty acids, ROS would react with the acyl chains of polyunsaturated fatty acids (PUFA) or their membrane lipid residues, triggering the chain reaction of lipid peroxidation (Fryer, 1992; Yang et al., 2008). In order to maintain the normal physiological function of cells under salt stress, cyanobacteria not only need to remove excessive ROS, but also the oxidation products of ROS, such as lipid peroxides. It has been showed that tocopherol and carotenoids were very important for the scavenging of lipid peroxides in Synechocystis 6803 (Maeda et al., 2005). The content of tocopherol in cyanobacteria increased under high light and decreased when glucose was added to the medium (Backasch et al., 2005). This effect also showed that tocopherol takes part in protection against oxidative damage.
Effects of Salt Stress on Polypeptide Composition of Photosystem
Salt stress could inhibit protein synthesis, with the D1 protein among the most obviously affected. In the natural environments, salt stress often occurs concomitantly with high light stress. Many studies have focused on the effects of salt stress on cyanobacterial PSII under intense light (Allakhverdiev and Murata, 2004; Allakhverdiev et al., 2005; Jantaro et al., 2005; Murata et al., 2007). A previous study revealed that exposure to 0.5 M NaCl can inhibit the repair of photodamaged PSII in Synechocystis 6803, but it did not directly accelerate the photodamage to PSII (Murata et al., 2007). It was also indicated that 0.5 M NaCl suppressed the synthesis of the D1 protein at the translational level (Murata et al., 2007). Allakhverdiev and Murata demonstrated that the initial rate of photoinactivation is unaffected by various NaCl concentrations, but the initial recovery rate decreases by 50 and 100% in the presence of 0.5 M and 1.0 M NaCl, respectively (Allakhverdiev and Murata, 2004). By monitoring the incorporation of [35S]Met into the thylakoid membrane proteins under the recovery conditions, they observed that the synthesis of the D1 protein and many other proteins was completely impeded by 1.0 M NaCl (Allakhverdiev and Murata, 2004). Jantaro et al. (2005) determined that under severe osmotic stress conditions, the abundance of the PSII D1 protein in Synechocystis 6803 was decreased, whereas the protein contents of PsaA/B and NdhF3 were unaffected. They also proved that osmotic stress is more detrimental to photosynthesis than ionic stress. The D1 protein appeared to be one of the proteins targeted by osmotic stress conditions (Jantaro et al., 2005).
The repair of PSII involves the degradation of D1, transcription of psbA genes, translation of psbA transcripts, incorporation of pre-D1 into PSII, processing of pre-D1, as well as the assembly of the PSII dimer and the oxygen-evolving complex (Figure 2; Murata et al., 2007). Ohnishi and Murata reported that salt stress inhibited not only protein synthesis but also the degradation of the D1 protein in the photodamaged PSII of Synechococcus elongatus PCC 7942 (hereafter Synechococcus 7942), with betaine alleviating both of these inhibitory effects (Ohnishi and Murata, 2006; Nishiyama and Murata, 2014). Allakhverdiev et al. (2002) observed that the translation of mRNAs might be the primary cellular process inhibited by a decrease in the intracellular ATP level. Transcription was only partially affected by the ATP content and was likely to be a secondary target (Jantaro et al., 2005). During translation, the extension of polypeptide chains and the transfer of amino acids require energy from ATP, making ATP synthesis a potential rate-limiting step for the complete repair of photodamaged PSII (Jantaro et al., 2005).
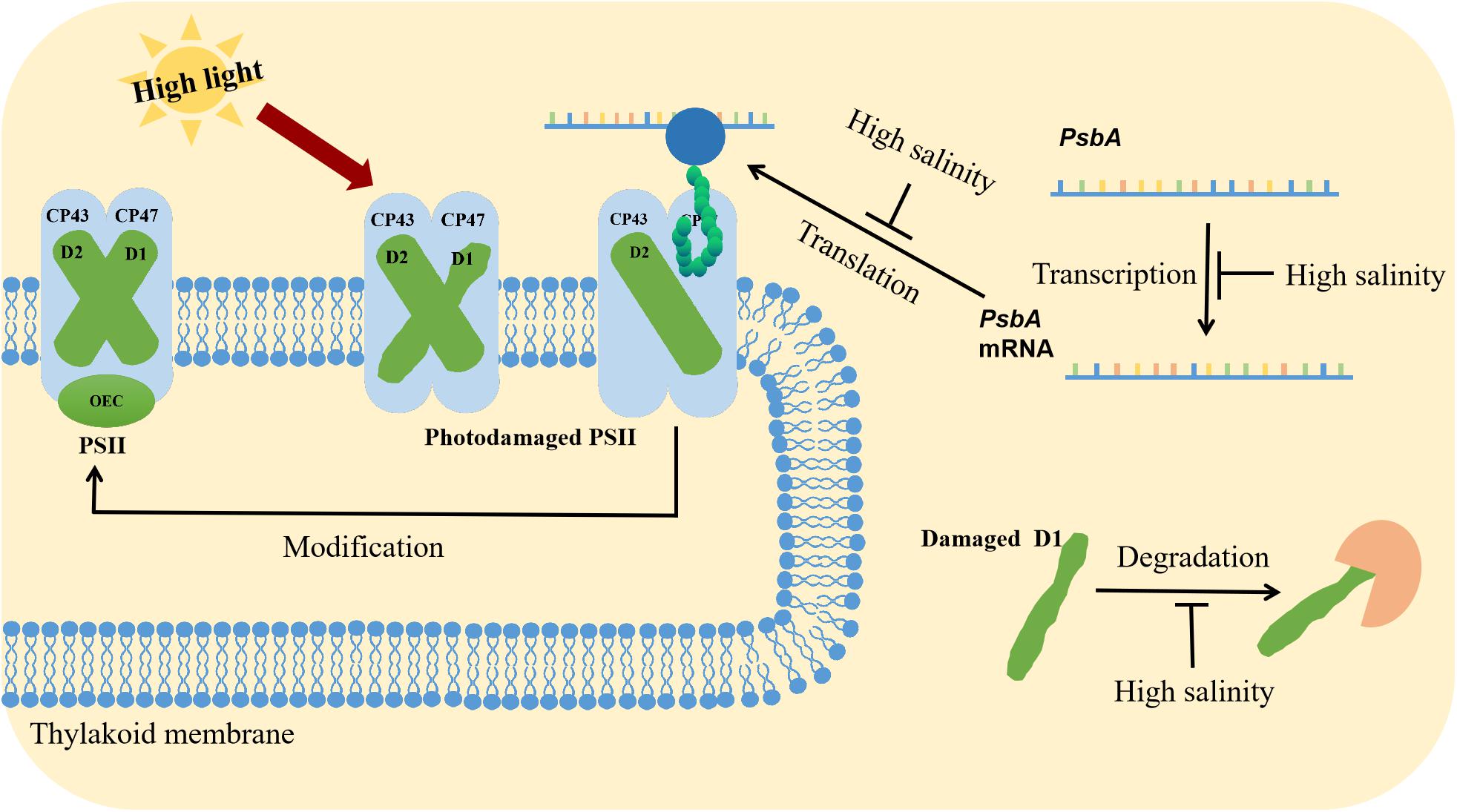
Figure 2. Synergistic effects of high light and salinity stress on PSII. High light stress destroys the PSII structure. The repair of PSII involves the degradation of the D1 protein, the transcription, and translation of psbA, the incorporation of pre-D1 into PSII, the processing of pre-D1, and the assembly of the PSII dimer and the oxygen-evolving complex. Salt stress inhibits psbA transcription and translation.
Nishiyama et al. (2001, 2004) reported that ROS could inhibit the elongation step of the translation of psbA mRNA, which encodes the D1 protein in Synechocystis 6803. Biochemical studies reveal that ROS destroyed EF-G and EF-Tu, which regulates translational elongation (Kojima et al., 2007; Yutthanasirikul et al., 2016; Jimbo et al., 2018). Specifically, EF-G translocated peptidyl-tRNA from the A site to the P site of the ribosome, and it forms an intramolecular disulfide bond when inactivated by H2O2 (Kojima et al., 2009). In contrast, EF-Tu delivered aminoacyl-tRNA to the A site of the ribosome, and when inactivated by H2O2, it formed sulfenic acid and an intermolecular disulfide bond (Yutthanasirikul et al., 2016). Intense light conditions could enhance de novo protein synthesis of a Synechocystis 6803 mutant, whose Cys-82 targeted by ROS is replaced by a Ser residue (Jimbo et al., 2018). Thus, it is reasonable that excessive ROS contents induced by environmental stress can inhibit translation by disrupting transcription factor structures.
Many experiments have confirmed that salt stress inhibits de novo protein synthesis during the PSII repair process in cyanobacteria, but in addition to the above studies, the effects of salt stress on the synthesis of other proteins in photosynthetic apparatus remain to be studied. And besides ROS stress, the other effects of salt stress (such as ion stress and osmotic stress) on protein synthesis also need to be further studied. Three hypotheses have been proposed (Murata et al., 2007). First, the influx of NaCl into the cell may directly inhibit protein synthesis by destabilizing polysomes and ribosomes (Tester and Davenport, 2003; Murata et al., 2007). It was previously indicated that Rubisco of Tamarix jordanis was inactivated in response to high NaCl concentrations (Solomon et al., 1994). Thus, another hypothesis is that the primary target of salt stress is Rubisco. Salt stress inhibits the fixation of CO2 and diminishes the regeneration of acceptors for the linear electron transport thereby inducing the generation of ROS, which subsequently inhibits protein synthesis (Murata et al., 2007; Nishiyama and Murata, 2014). The third hypothesis is that an increase in the intracellular NaCl concentration inactivates ATP synthesis, thereby decreasing the intracellular ATP content that is essential for protein synthesis (Allakhverdiev et al., 2005). In addition to these hypotheses, we speculate that salt stress may also indirectly inhibit protein synthesis by inducing ROS production.
Effect of Lipid Composition and Fatty Acid Desaturation on the Salt Resistance of Cyanobacteria
The cell membrane is a sensor of environmental stress, which can activate a series of protective reactions by adjusting the stress perception and rigidity of cell structure (Sui et al., 2018). In cyanobacteria and plants, the thylakoid membrane structure and fluidity were affected by lipid composition and the degree of fatty acid desaturation (Mikami and Murata, 2003). The relationship between lipids and salt stress has been widely studied in plants and cyanobacteria. Sui et al. demonstrated that salt stress increases the content of unsaturated fatty acids in the euhalophyte Suaeda salsa, which increased the photosystem tolerance to salt stress (Sui et al., 2017). In the halophyte Thellungiella, increasing the content of phosphatidylglycerol (PG) and sulfoquinovosyl diacylglycerol (SQDG) as well as the ratio of monogalactosyldiacylglycerols/digalactosyldiacylglycerol (MGDG/DGDG) in thylakoid membranes can alleviate PSII photoinhibition under salt stress (Sui and Han, 2014).
In response to salt stress, cyanobacteria could alter not only the composition of lipids but also the unsaturated degree of membrane lipids. The changes in lipid composition of Synechococcus sp. PCC6311 under salt stress include the increase of the proportion of unsaturated fatty acids and phosphatidyl glycerol (Huflejt et al., 1990). Ritter and Yopp (1993) observed that the proportion of mono/digalactosyl diacyl glycerols changes when exposed the cyanobacterium Aphanothece halophytica to salt stress. Changes in ion-exchange properties of the cytoplasmic membrane caused by changes in polar lipids may hamper the function of potassium ion channels (Joset et al., 1996), thereby reducing the inflow of Na+ and reducing the damage of photosynthetic apparatus. The decrease in the protein to lipid ratio led to increased cytochrome oxidase and H+/Na+ antiport activities in Synechococcus 6301 under salt stress (Peschek et al., 1994; Srivastava et al., 2013), which also reduced the damage of photosynthetic apparatus.
The extent of the desaturation of individual fatty acids is regulated genetically and environmentally, and the content of unsaturated fatty acids will influence the photosynthetic machinery under salt stress. Synechocystis 6803 contains four acyl lipid desaturases, DesA, DesB, DesC, and DesD, which catalyze the desaturation at the Δ12, Δ15, Δ9, and Δ6 positions of C18 fatty acyl chains in membrane lipids, respectively (Murata and Wada, 1995; Chintalapati et al., 2006). The oxygen-evolving machinery in thylakoid membranes isolated from the desA–/desD– cells of Synechocystis 6803 (the double mutant of desA and desD genes) was more sensitive to NaCl than that of the WT cells, indicating that the desaturation of membrane lipid fatty acids may directly protect the oxygen-evolving machinery against salt-induced inactivation (Allakhverdiev et al., 1999). In order to further study on the function of desA, Allakhverdiev et al. (2001) constructed desA+ cells by overexpressing the desA gene in Synechococcus 7942 that do not have the desA gene. The results showed that WT cells are more sensitive to NaCl and have the reduced capacity of recovery than desA+ cells (Allakhverdiev et al., 2001). These studies showed that the increase of unsaturated fatty acids in membrane lipids enhances the tolerance of the photosynthetic and Na+/H+ anti-port systems in cyanobacteria to salt stress (Allakhverdiev et al., 2001; Singh et al., 2002).
The desaturation of fatty acids in membrane lipids may protect the oxygen-evolving machinery by stimulating the synthesis of the protein(s) in the Na+/H+ antiport system (Allakhverdiev et al., 1999). The energy generated by photosynthesis and respiration overcomes the inhibition of protein synthesis by NaCl, thus enabling the repair of the oxygen-releasing complex. The following scheme might explain why light or glucose can restore the oxygen-evolving activity (Allakhverdiev et al., 1999). The lack of an ATP supply under the dark and salt conditions indirectly inhibited the activities of the Na+/H+ antiport system and caused sodium ions to flow into the thylakoid cavity, leading to the deactivation of the oxygen-releasing complex. The presence of NaCl in the dark impeded the synthesis of certain proteins, whereas exposure to light has the opposite effect. The above-mentioned results implied that the affected proteins belong to the Na+/H+ antiport system. Compared with the WT Na+/H+ antiport system, the mutant was more sensitive to salt and its repair rate was slower (Allakhverdiev et al., 1999). Therefore, unsaturation of fatty acids might also stimulate the synthesis of protein(s) in the Na+/H+ antiport system.
The rbp3 gene, which encodes a type II RNA-binding protein, is involved in maintaining the transcript levels of acyl-lipid desaturase genes (desA, desB, and desD) and the substantial abundance of unsaturated membrane lipids (Tang et al., 2010). It was shown that 2-day treatment with 1 M NaCl decreased the photosynthetic activity of Δrbp3 to 8.4% of the WT level (Wang and Xu, 2013). The complementation with rbp3 fully restored the photosynthetic activity, whereas the overexpression of desA only partially restored the activity (Wang and Xu, 2013). Thus, rbp3 may maintain the extent of unsaturated lipids in the cell membrane by enhancing the stability of des mRNAs, thereby stabilizing the photosynthesis of cyanobacteria under salt stress conditions.
Conclusion and Perspectives
Salt stress inhibits protein synthesis, especially the D1 protein. Osmotic stress may directly inhibit protein synthesis. Moreover, it seems that the accumulated ROS caused by salt stress mainly inhibits the translation process. However, whether salt stress affects the modification processing of pre-D1 in cyanobacteria remains unclear.
Aquaporins may be able to stabilize photosystems by transferring ROS to other regions, since some plant aquaporins expressed in yeast can transport H2O2 molecules (Bienert et al., 2007). However, the function of aquaporins and the associated mechanisms in cyanobacteria remits further characterization.
Cyanobacteria represent an excellent model system for studying the relationship between membrane systems and photosynthesis under stress conditions. Several studies have revealed the protective effects of membrane lipids and unsaturated degree of fatty acids on photosystems under salt stress, but the protective effects of the composition of different lipids on the photosynthetic apparatus of cyanobacteria under salt stress remains to be further studied. Whether salt stress affects the lipid-assisted PSI oligomerization and affects energy transfer between phycobilisomes and the photosystem also requires further exploration. Although there is increasing interest in uncovering the effects of salt stress on photosynthetic activities in cyanobacteria, the underlying mechanism remains to be elucidated.
Author Contributions
WY initiated preparation of the manuscript. NS, FW, and L-NL conceptualized the idea and revised the manuscript. All authors have read and approved the final manuscript.
Funding
We are grateful for the financial support from the National Key R&D Program of China (2018YFD1000700 and 2018YFD1000704), the National Natural Science Foundation of China (U1906204 and 31871538), Shandong Province Key Research and Development Program (2019GSF107079), the Development Plan for Youth Innovation Team of Shandong Provincial (2019KJE012), the Opening Foundation of Shandong Key Laboratory of Crop Genetic Improvement, Ecology and Physiology (SDKL2018008-3), Royal Society University Research Fellowship (UF120411 and URF\R\180030, L-NL), Royal Society Grants (RGF\EA\181061 and RGF\EA\180233), and Biotechnology and Biological Sciences Research Council Grant (BB/R003890/1, L-NL).
Conflict of Interest
The authors declare that the research was conducted in the absence of any commercial or financial relationships that could be construed as a potential conflict of interest.
References
Ahanger, M. A., Tomar, N. S., Tittal, M., Argal, S., and Agarwal, R. M. (2017). Plant growth under water/salt stress: ROS production; antioxidants and significance of added potassium under such conditions. Physiol. Mol. Biol. Plants 23, 731–744. doi: 10.1007/s12298-017-0462-7
Akai, M., Onai, K., Kusano, M., Sato, M., Redestig, H., Toyooka, K., et al. (2011). Plasma membrane aquaporin aqpz protein is essential for glucose metabolism during photomixotrophic growth of Synechocystis sp. pcc 6803. J. Biol. Chem. 286, 25224–25235. doi: 10.1074/jbc.M111.236380
Allahverdiyeva, Y., Ermakova, M., Eisenhut, M., Zhang, P., Richaud, P., Hagemann, M., et al. (2011). Interplay between flavodiiron proteins and photorespiration in Synechocystis sp. PCC 6803. J. Biol. Chem. 286, 24007–24014. doi: 10.1074/jbc.m111.223289
Allahverdiyeva, Y., Mustila, H., Ermakova, M., Bersanini, L., Richaud, P., Ajlani, G., et al. (2013). Flavodiiron proteins Flv1 and Flv3 enable cyanobacterial growth and photosynthesis under fluctuating light. Proc. Natl. Acad. Sci. U.S.A. 110, 4111–4116. doi: 10.1073/pnas.1221194110
Allakhverdiev, S. I., Kinoshita, M., Inaba, M., Suzuki, I., and Murata, N. (2001). Unsaturated fatty acids in membrane lipids protect the photosynthetic machinery against salt-induced damage in Synechococcus. Plant Physiol. 125, 1842–1853. doi: 10.1104/pp.125.4.1842
Allakhverdiev, S. I., and Murata, N. (2004). Environmental stress inhibits the synthesis de novo of proteins involved in the photodamage-repair cycle of photosystem II in Synechocystis sp. PCC 6803. Biochim. Biophys. Acta Bioenerg. 1657, 23–32. doi: 10.1016/j.bbabio.2004.03.003
Allakhverdiev, S. I., and Murata, N. (2008). Salt stress inhibits photosystems II and I in cyanobacteria. Photosynth. Res. 98, 529–539. doi: 10.1007/s11120-008-9334-x
Allakhverdiev, S. I., Nishiyama, Y., Miyairi, S., Yamamoto, H., Inagaki, N., Kanesaki, Y., et al. (2002). Salt stress inhibits the repair of photodamaged photosystem II by suppressing the transcription and translation of psbA Genes in Synechocystis. Plant Physiol. 130, 1443–1453. doi: 10.1104/pp.011114
Allakhverdiev, S. I., Nishiyama, Y., Suzuki, I., Tasaka, Y., and Murata, N. (1999). Genetic engineering of the unsaturation of fatty acids in membrane lipids alters the tolerance of Synechocystis to salt stress. Proc. Natl. Acad. Sci U.S.A. 96, 5862–5867. doi: 10.1073/pnas.96.10.5862
Allakhverdiev, S. I., Nishiyama, Y., Takahashi, S., Miyairi, S., Suzuki, I., and Murata, N. (2005). Systematic analysis of the relation of electron transport and ATP synthesis to the photodamage and repair of photosystem II in Synechocystis. Plant Physiol. 137, 263–273. doi: 10.1104/pp.104.054478
Allakhverdiev, S. I., Sakamoto, A., Nishiyama, Y., Inaba, M., and Murata, N. (2000). Ionic and osmotic effects of NaCl-induced inactivation of photosystem I and II in Synechococcus sp. Plant Physiol. 123, 1047–1056. doi: 10.1104/pp.123.3.1047
Asada, K. (1999). The water-water cycle in chloroplasts: scavenging of active oxygens and dissipation of excess photons. Annu. Rev. Plant Physiol. Plant Mol. Biol. 50, 601–639. doi: 10.1146/annurev.arplant.50.1.601
Asada, K. (2000). The water-water cycle as alternative photon and electron sinks. Philos. Trans. R. Soc. Lond. B 355, 1419–1431. doi: 10.1098/rstb.2000.0703
Backasch, N., Schulz-Friedrich, R., and Appel, J. (2005). Influences on tocopherol biosynthesis in the cyanobacterium Synechocystis sp. PCC 6803. J. Plant Physiol. 162, 758–766. doi: 10.1016/j.jplph.2005.04.006
Bienert, G. P., Møller, A. L. B., Kristiansen, K. A., Schulz, A., Møller, I. M., Schjoerring, J. K., et al. (2007). Specific aquaporins facilitate the diffusion of hydrogen peroxide across membranes. J. Biol. Chem. 282, 1183–1192. doi: 10.1074/jbc.M603761200
Chen, Y., Han, Y., Kong, X., Kang, H., Ren, Y., and Wang, W. (2017). Ectopic expression of wheat expansin gene TaEXPA2 improved the salt tolerance of transgenic tobacco by regulating Na+/K+ and antioxidant competence. Physiol. Plant. 159, 161–177. doi: 10.1111/ppl.12492
Chintalapati, S., Prakash, J. S. S., Gupta, P., Ohtani, S., Suzuki, I., Sakamoto, T., et al. (2006). A novel Δ9 acyl-lipid desaturase, DesC2, from cyanobacteria acts on fatty acids esterified to the sn-2 position of glycerolipids. Biochem. J. 398, 207–214. doi: 10.1042/BJ20060039
del RÍo, L. A., Corpas, F. J., Sandalio, L. M., Palma, J. M., GÓmez, M., and Barroso, J. B. (2002). Reactive oxygen species, antioxidant systems and nitric oxide in peroxisomes. J. Exp. Bot. 53, 1255–1272. doi: 10.1093/jxb/53.372.1255
Desplats, P., Folco, E., and Salerno, G. L. (2005). Sucrose may play an additional role to that of an osmolyte in Synechocystis sp. PCC 6803 salt-shocked cells. Plant Physiol. Biochem. 43, 133–138. doi: 10.1016/j.plaphy.2005.01.008
Dominguez-Martin, M. A., and Kerfeld, C. A. (2019). Engineering the orange carotenoid protein for applications in synthetic biology. Curr. Opin. Struct. Biol. 57, 110–117. doi: 10.1016/j.sbi.2019.01.023
Fan, Y., Yin, X., Xie, Q., Xia, Y., Wang, Z., Song, J., et al. (2019). Co-expression of SpSOS1 and SpAHA1 in transgenic Arabidopsis plants improves salinity tolerance. BMC Plant Biol. 19:74. doi: 10.1186/s12870-019-1680-7
Feng, Z. T., Deng, Y. Q., Fan, H., Sun, Q. J., Sui, N., and Wang, B. S. (2014). Effects of NaCl stress on the growth and photosynthetic characteristics of Ulmus pumila L. seedlings in sand culture. Photosynthetica 52, 313–320. doi: 10.1007/s11099-014-0032-y
Feng, Z. T., Deng, Y. Q., Zhang, S. C., Liang, X., Yuan, F., Hao, J. L., et al. (2015). K+ accumulation in the cytoplasm and nucleus of the salt gland cells of Limonium bicolor accompanies increased rates of salt secretion under NaCl treatment using NanoSIMS. Plant Sci. 238, 286–296. doi: 10.1016/j.plantsci.2015.06.021
Foyer, C. H., and Noctor, G. (2003). Redox sensing and signalling associated with reactive oxygen in chloroplasts, peroxisomes and mitochondria. Physiol. Plant. 119, 355–364. doi: 10.1034/j.1399-3054.2003.00223.x
Frank, H. A., and Cogdell, R. J. (1996). Carotenoids in photosynthesis. Photochem. Photobiol. 63, 257–264. doi: 10.1111/j.1751-1097.1996.tb03022.x
Fryer, M. J. (1992). The antioxidant effects of thylakoid Vitamin E (α-tocopherol). Plant Cell Environ. 15, 381–392. doi: 10.1111/j.1365-3040.1992.tb00988.x
Fulda, S., Mikkat, S., Huang, F., Huckauf, J., and Hagemann, M. (2006). Proteome analysis of salt stress response in the cyanobacterium synechocystis sp. strain pcc 6803. Proteomics 6, 2733–2745. doi: 10.1002/pmic.200500538
Gill, S. S., and Tuteja, N. (2010). Reactive oxygen species and antioxidant machinery in abiotic stress tolerance in crop plants. Plant Physiol. Biochem. 48, 909–930. doi: 10.1016/j.plaphy.2010.08.016
Gorman, A. A., and Rodgers, M. A. (1992). Current perspectives of singlet oxygen detection in biological environments. J. Photochem. Photobiol. B Biol. 14, 159–176. doi: 10.1016/1011-1344(92)85095-C
Han, N., Lan, W., He, X., Shao, Q., Wang, B., and Zhao, X. (2012). Expression of a Suaeda salsa vacuolar H+/Ca2+ transporter gene in Arabidopsis contributes to physiological changes in salinity. Plant Mol. Biol. Rep. 30, 470–477. doi: 10.1007/s11105-011-0353-y
Haselkorn, R. (1991). Genetic systems in cyanobacteria. Methods Enzymol. 204, 418–430. doi: 10.1016/0076-6879(91)04022-G
Helman, Y., Barkan, E., Eisenstadt, D., Luz, B., and Kaplan, A. (2005). Fractionation of the three stable oxygen isotopes by oxygen-producing and oxygen-consuming reactions in photosynthetic organisms. Plant Physiol. 138, 2292–2298. doi: 10.1104/pp.105.063768
Helman, Y., Tchernov, D., Reinhold, L., Shibata, M., Ogawa, T., Schwarz, R., et al. (2003). Genes encoding A-type flavoproteins are essential for photoreduction of O2 in cyanobacteria. Curr. Biol. 13, 230–235. doi: 10.1016/s0960-9822(03)00046-0
Hu, D. G., Ma, Q. J., Sun, C. H., Sun, M. H., You, C. X., and Hao, Y. J. (2016). Overexpression of MdSOS2L1, a CIPK protein kinase, increases the antioxidant metabolites to enhance salt tolerance in apple and tomato. Physiol. Plant. 156, 201–214. doi: 10.1111/ppl.12354
Huang, F., Fulda, S., Hagemann, M., and Norling, B. (2006). Proteomic screening of salt-stress-induced changes in plasma membranes of Synechocystis sp. strain PCC 6803. Proteomics 6, 910–920. doi: 10.1002/pmic.200500114
Huflejt, M. E., Tremolieres, A., Pineau, B., Lang, J. K., Hatheway, J., and Packer, L. (1990). Changes in membrane lipid composition during saline growth of the fresh water cyanobacterium Synechococcus 6311. Plant Physiol. 94, 1512–1521. doi: 10.1104/pp.94.4.1512
Jantaro, S., Mulo, P., Jansén, T., Incharoensakdi, A., and Mäenpää, P. (2005). Effects of long-term ionic and osmotic stress conditions on photosynthesis in the cyanobacterium Synechocystis sp. PCC 6803. Funct. Plant Biol. 32, 807–815. doi: 10.1071/FP04219
Jensen, P. E., and Leister, D. (2014). Cyanobacteria as an experimental platform for modifying bacterial and plant photosynthesis. Front. Bioeng. Biotechnol. 2:7. doi: 10.3389/fbioe.2014.00007
Jimbo, H., Yutthanasirikul, R., Nagano, T., Hisabori, T., Hihara, T., and Nishiyama, Y. (2018). Oxidation of translation factor EF-Tu inhibits the repair of photosystem II. Plant Physiol. 176, 2691–2699. doi: 10.1104/pp.18.00037
Joset, F., Jeanjean, R., and Hagemann, M. (1996). Dynamics of the response of cyanobacteria to salt stress: deciphering the molecular events. Physiol. Plant. 96, 738–744. doi: 10.1111/j.1399-3054.1996.tb00251.x
Kanesaki, Y., Suzuki, I., Allakhverdiev, S. I., Mikami, K., and Murata, N. (2002). Salt stress and hyperosmotic stress regulate the expression of different sets of genes in Synechocystis sp. PCC 6803. Biochem. Biophys. Res. Commun. 290, 339–348. doi: 10.1006/bbrc.2001.6201
Karandashova, I. V., and Elanskaya, I. V. (2005). Genetic control and mechanisms of salt and hyperosmotic stress resistance in cyanobacteria. Russ. J. Genet. 41, 1311–1321. doi: 10.1007/s11177-006-0001-z
Kawasaki, S., Mizuguchi, K., Sato, M., Kono, T., and Shimizu, H. (2013). A novel astaxanthin-binding photooxidative stress-inducible aqueous carotenoprotein from a eukaryotic microalga isolated from asphalt in midsummer. Plant Cell Physiol. 54, 1027–1040. doi: 10.1093/pcp/pct080
Kirilovsky, D., and Kerfeld, C. A. (2013). The Orange Carotenoid Protein: a blue-green light photoactive protein. Photochem. Photobiol. 12, 1135–1143. doi: 10.1039/C3PP25406B
Kirilovsky, D., and Kerfeld, C. A. (2016). Cyanobacterial photoprotection by the orange carotenoid protein. Nat. Plants 2:16180. doi: 10.1038/nplants.2016.180
Kojima, K., Motohashi, K., Morota, T., Oshita, M., Hisabori, T., Hayashi, H., et al. (2009). Regulation of translation by the redox state of elongation factor G in the cyanobacterium Synechocystis sp. PCC 6803. J. Biol. Chem. 284, 18685–18691. doi: 10.1074/jbc.M109.015131
Kojima, K., Oshita, M., Nanjo, Y., Kasai, K., Tozawa, Y., Hayashi, H., et al. (2007). Oxidation of elongation factor G inhibits the synthesis of the D1 protein of photosystem II. Mol. Microbiol. 65, 936–947. doi: 10.1111/j.1365-2958.2007.05836.x
Latifi, A., Ruiz, M., and Zhang, C. C. (2009). Oxidative stress in cyanobacteria. FEMS Microbiol. Rev. 33, 258–278. doi: 10.1111/j.1574-6976.2008.00134.x
Li, H. M., Kaneko, Y., and Keegstra, K. (1994). Molecular cloning of a chloroplastic protein associated with both the envelope and thylakoid membranes. Plant Mol. Biol. 25, 619–632. doi: 10.1007/BF00029601
Liu, X. G., Zhao, J. J., and Wu, Q. Y. (2005). Oxidative stress and metal ions effects on the cores of phycobilisomes in Synechocystis sp. PCC6803. FEBS Lett. 579, 4571–4576. doi: 10.1016/j.febslet.2005.07.020
Los, D. A., and Murata, N. (2004). Membrane fluidity and its roles in the perception of environmental signals. Biochim. Biophys. Acta 1666, 142–157. doi: 10.1016/j.bbamem.2004.08.002
Los, D. A., Zorina, A., Sinetova, M., Kryazhov, S., Mironov, K., and Zinchenko, V. V. (2010). Stress sensors and signal transducers in cyanobacteria[J]. Sensors 10, 2386–2415. doi: 10.3390/s100302386
Lu, C., and Vonshak, A. (1999). Characterization of PSII photochemistry in salt-adapted cells of cyanobacterium Spirulina platensis. New Phytol. 141, 231–239. doi: 10.1046/j.1469-8137.1999.00340.x
Lu, C., and Vonshak, A. (2002). Effects of salinity stress on photosystem II function in cyanobacterial Spirulina platensis cells. Physiol. Plant. 114, 405–413. doi: 10.1034/j.1399-3054.2002.1140310.x
Lu, C., and Zhang, J. (2000). Role of light in the response of PSII photochemistry to salt stress in the cyanobacterium Spirulina platensis. J. Exp. Bot. 51, 911–917. doi: 10.1093/jexbot/51.346.911
Ma, Q. J., Sun, M. H., Kang, H., Lu, J., You, C. X., and Hao, Y. J. (2019). A CIPK protein kinase targets sucrose transporter MdSUT2. 2 at Ser254 for phosphorylation to enhance salt tolerance. Plant Cell Environ. 42, 918–930. doi: 10.1111/pce.13349
Maeda, H., Sakuragi, Y., Bryant, D. A., and DellaPenna, D. (2005). Tocopherols protect Synechocystis sp. strain PCC 6803 from lipid peroxidation. Plant Physiol. 138, 1422–1435. doi: 10.1104/pp.105.061135
Marin, K., Kanesaki, Y., Los, D. A., Murata, N., Suzuki, I., and Hagemann, M. (2004). Gene expression profiling reflects physiological processes in salt acclimation of Synechocystis sp. strain PCC 6803. Plant Physiol. 136, 3290–3300. doi: 10.1104/pp.104.045047
Maurel, C. (2007). Plant aquaporins: novel functions and regulation properties. FEBS Lett. 581, 2227–2236. doi: 10.1016/j.febslet.2007.03.021
Mehler, A. H. (1951). Studies on reactions of illuminated chloroplasts: I. Mechanism of the reduction of oxygen and other Hill reagents. Arch. Biochem. Biophys. 33, 65–77. doi: 10.1016/0003-9861(51)90082-3
Mehler, A. H., and Brown, A. H. (1952). Studies on reactions of illuminated chloroplasts: III. Simultaneous photoproduction and consumption of oxygen studied with oxygen isotopes. Arch. Biochem. Biophys. 38, 365–370. doi: 10.1016/0003-9861(52)90042-8
Mikami, K., and Murata, N. (2003). Membrane fluidity and the perception of environmental signals in cyanobacteria and plants. Prog. Lipid Res. 42, 527–543. doi: 10.1016/S0163-7827(03)00036-5
Mittler, R. (2002). Oxidative stress, antioxidants and stress tolerance. Trends Plant Sci. 7, 405–410. doi: 10.1016/S1360-1385(02)02312-9
Moller, I. M. (2001). Plant mitochondria and oxidative stress: electron transport, nadph turnover, and metabolism of reactive oxygen species. Annu. Rev. Plant Physiol. Plant Mol. Biol. 52, 561–591. doi: 10.1146/annurev.arplant.52.1.561
Montillet, J. L., Chamnongpol, S., Rustérucci, C., Dat, J., van de Cotte, B., Agnel, J. P., et al. (2005). Fatty acid hydroperoxides and H2O2 in the execution of hypersensitive cell death in tobacco leaves. Plant Physiol. 138, 1516–1526. doi: 10.1104/pp.105.059907
Mullineaux, C. W. (2014). Co-existence of photosynthetic and respiratory activities in cyanobacterial thylakoid membranes. Biochim. Biophys. Acta Bioenerg. 1837, 503–511. doi: 10.1016/j.bbabio.2013.11.017
Murata, N., Takahashi, S., Nishiyama, Y., and Allakhverdiev, S. I. (2007). Photoinhibition of photosystem II under environmental stress. Biochim. Biophys. Acta Bioenerg. 1767, 414–421.
Murata, N., and Wada, H. (1995). Acyl-lipid desaturases and their importance in the tolerance and acclimatization to cold of cyanobacteria. Biochem. J. 308, 1–8. doi: 10.1042/bj3080001
Nishiyama, Y., Allakhverdiev, S. I., Yamamoto, H., Hayashi, H., and Murata, N. (2004). Singlet oxygen inhibits the repair of photosystem II by suppressing the translation elongation of the D1 protein in Synechocystis sp. PCC 6803. Biochemistry 43, 11321–11330. doi: 10.1021/bi036178q
Nishiyama, Y., and Murata, N. (2014). Revised scheme for the mechanism of photoinhibition and its application to enhance the abiotic stress tolerance of the photosynthetic machinery. Appl. Microbiol. Biotechnol. 98, 8777–8796. doi: 10.1007/s00253-014-6020-0
Nishiyama, Y., Yamamoto, H., Allakhverdiev, S. I., Inaba, M., Yokota, A., and Murata, N. (2001). Oxidative stress inhibits the repair of photodamage to the photosynthetic machinery. EMBO J. 20, 5587–5594. doi: 10.1093/emboj/20.20.5587
Ohnishi, N., and Murata, N. (2006). Glycinebetaine counteracts the inhibitory effects of salt stress on the degradation and synthesis of D1 protein during photoinhibition in Synechococcus sp. PCC 7942. Plant Physiol. 141, 758–765. doi: 10.1104/pp.106.076976
Öquist, G., Campbell, D., Clarke, A. K., and Gustafsson, P. (1995). The cyanobacterium Synechococcus modulates Photosystem II function in response to excitation stress through D1 exchange. Photosynth. Res. 46, 151–158. doi: 10.1007/BF00020425
Peschek, G. A., Obinger, C., Fromwald, S., and Bergman, B. (1994). Correlation between immuno-gold labels and activities of the cytochrome-c oxidase (aa3-type) in membranes of salt stressed cyanobactria. FEMS Microbiol. Lett. 124, 431–437. doi: 10.1111/j.1574-6968.1994.tb07320.x
Qiao, J. J., Huang, S. Q., Te, R. G., Wang, J. X., Chen, L., and Zhang, W. W. (2013). Integrated proteomic and transcriptomic analysis reveals novel genes and regulatory mechanisms involved in salt stress responses in Synechocystis sp. PCC 6803. Appl. Microbiol. Biotechnol. 97, 8253–8264. doi: 10.1007/s00253-013-5139-8
Ritter, D., and Yopp, J. H. (1993). Plasma membrane lipid composition of the halophilic cyanobacterium Aphanothece halophytica. Arch. Microbiol. 159, 435–439. doi: 10.1007/BF00288590
Rodriguez-Ezpeleta, N., Brinkmann, H., Burey, S. C., Roure, B., Burger, G., Loffelhardt, W., et al. (2005). Monophyly of primary photosynthetic eukaryotes: green plants, red algae, and glaucophytes. Curr. Biol. 15, 1325–1330. doi: 10.1016/j.cub.2005.06.040
Scarpeci, T. E., Zanor, M. I., Carrillo, N., Mueller-Roeber, B., and Valle, E. M. (2008). Generation of superoxide anion in chloroplasts of Arabidopsis thaliana during active photosynthesis: a focus on rapidly induced genes. Plant Mol. Biol. 66, 361–378. doi: 10.1007/s11103-007-9274-4
Sedoud, A., Lopez-Igual, R., ur Rehman, A., Wilson, A., Perreau, F., Boulay, C., et al. (2014). The cyanobacterial photoactive orange carotenoid protein is an excellent singlet oxygen quencher. Plant Cell 26, 1781–1791. doi: 10.1105/tpc.114.123802
Serrano, R., and Rodrigueznavarro, A. (2001). Ion homeostasis during salt stress in plants. Curr. Opin. Cell Biol. 13, 399–404. doi: 10.1016/S0955-0674(00)00227-1
Shabala, S. (2013). Learning from halophytes: physiological basis and strategies to improve abiotic stress tolerance in crops. Ann. Bot. 112, 1209–1221. doi: 10.1093/aob/mct205
Shao, Q., Han, N., Ding, T., Zhou, F., and Wang, B. (2014). SsHKT1; 1 is a potassium transporter of the C3 halophyte Suaeda salsa that is involved in salt tolerance. Funct. Plant Biol. 41, 790–802. doi: 10.1071/FP13265
Singh, S. C., Sinha, R. P., and Hader, D. P. (2002). Role of lipids and fatty acids in stress tolerance in cyanobacteria. Acta Protozool. 41, 297–308. doi: 10.1016/S0378-1097(02)01030-3
Solomon, A., Beer, S., Waisel, Y., Jones, G. P., and Paleg, L. G. (1994). Effects of NaCl on the carboxylating activity of Rubisco from Tamarix jordanis in the presence and absence of proline-related compatible solutes. Physiol. Plant. 90, 198–204. doi: 10.1111/j.1399-3054.1994.tb02211.x
Song, J., and Wang, B. S. (2014). Using euhalophytes to understand salt tolerance and to develop saline agriculture: Suaeda salsa as a promising model. Ann. Bot. 115, 541–553. doi: 10.1093/aob/mcu194
Song, Y., Li, J., Sui, Y., Han, G., Zhang, Y., Guo, S., et al. (2020). The sweet sorghum SbWRKY50 is negatively involved in salt response by regulating ion homeostasis. Plant Mol. Biol. 102, 603–614. doi: 10.1007/s11103-020-00966-4
Sozer, O., Komenda, J., Ughy, B., Domonkos, I., Laczkó-Dobos, H., Malec, P., et al. (2010). Involvement of carotenoids in the synthesis and assembly of protein subunits of photosynthetic reaction centers of Synechocystis sp. PCC 6803. Plant Cell Physiol. 51, 823–835. doi: 10.1093/pcp/pcq031
Srivastava, A. K., Rai, A. N., and Neilan, B. A. (2013). Stress Biology of Cyanobacteria: Molecular Mechanisms to Cellular Responses. Boca Raton, FL: CRC Press, 172–173. doi: 10.1111/jpy.12201
Sudhir, P., Pogoryelov, D., Kovacs, L., Garab, G., and Murthy, S. (2005). The effects of salt stress on photosynthetic electron transport and thylakoid membrane proteins in the cyanobacterium Spirulina platensis. J. Biochem. Mol. Biol. 38, 481–485. doi: 10.5483/BMBRep.2005.38.4.481
Sui, N., and Han, G. L. (2014). Salt-induced photoinhibition of PSII is alleviated in halophyte Thellungiella halophila by increases of unsaturated fatty acids in membrane lipids. Acta Physiol. Plant. 36, 983–992. doi: 10.1007/s11738-013-1477-5
Sui, N., Tian, S., Wang, W., Wang, M., and Fan, H. (2017). Overexpression of glycerol-3-phosphate acyltransferase from Suaeda salsa improves salt tolerance in Arabidopsis. Front. Plant Sci. 8:1337. doi: 10.3389/fpls.2017.01337/full
Sui, N., Wang, Y., Liu, S., Yang, Z., Wang, F., and Wan, S. B. (2018). Transcriptomic and physiological evidence for the relationship between unsaturated fatty acid and salt stress in peanut. Front. Plant Sci. 9:7. doi: 10.3389/fpls.2018.00007
Tandeau de Marsac, N., and Houmard, J. (1993). Adaptation of cyanobacteria to environmental stimuli: new steps towards molecular mechanisms. FEMS Microbiol. Rev. 10, 119–189. doi: 10.1111/j.1574-6968.1993.tb05866.x
Tang, Q., Tan, X. M., and Xu, X. D. (2010). Effects of a type-II RNA-binding protein on fatty acid composition in Synechocystis sp. PCC 6803. Chin. Sci. Bull. 55, 2416–2421. doi: 10.1007/s11434-010-3254-9
Tester, M., and Davenport, R. (2003). Na? tolerance and Na? transport in higher plants. Ann. Bot. 91, 503–527. doi: 10.1093/aob/mcg058
Van Thor, J. J., Jeanjean, R., Havaux, M., Sjollema, K. A., Joset, F., Hellingwerf, K. J., et al. (2000). Salt shock-inducible photosystem I cyclic electron transfer in Synechocystis PCC6803 relies on binding of ferredoxin: NADP(+) reductase to the thylakoidmembranes via its CpcD phycobilisome-linker homologous N-terminal domain. Biochim. Biophys. Acta 1457, 129–144. doi: 10.1016/s0005-2728(00)00072-4
Vicente, J. B., Gomes, C. M., Wasserfallen, A., and Teixeira, M. (2002). Module fusion in an A-type flavoprotein from the cyanobacterium Synechocystis condenses a multiple-component pathway in a single polypeptide chain. Biochem. Biophys. Res. Commun. 294, 82–87. doi: 10.1016/S0006-291X(02)00434-5
Vothknecht, U. C., Otters, S., Hennig, R., and Schneider, D. (2012). Vipp1: a very important protein in plastids?! J. Exp. Bot. 63, 1699–1712. doi: 10.1093/jxb/err357
Wada, N., Sakamoto, T., and Matsugo, S. (2013). Multiple roles of photosynthetic and sunscreen pigments in cyanobacteria focusing on the oxidative stress. Metabolites 3, 463–483. doi: 10.3390/metabo3020463
Wang, Y., and Xu, X. D. (2013). Effects of Rbp3 on lipid peroxidation and salt tolerance in Synechocystis sp. PCC 6803. FEBS Lett. 587, 1446–1451. doi: 10.1016/j.febslet.2013.03.028
Wei, D., Zhang, W., Wang, C., Meng, Q., Li, G., Chen, T. H., et al. (2017). Genetic engineering of the biosynthesis of glycinebetaine leads to alleviate salt-induced potassium efflux and enhances salt tolerance in tomato plants. Plant Sci. 257, 74–83. doi: 10.1016/j.plantsci.2017.01.012
Wicke, B., Sikkema, R., Dornburg, V., and Faaij, A. (2011). Exploring land use changes and the role of palm oil production in Indonesia and Malaysia. Land Use Policy 28, 193–206. doi: 10.1016/j.landusepol.2010.06.001
Wilson, A., Ajlani, G., Verbavatz, J. M., Vass, I., Kerfeld, C. A., and Kirilovsky, D. (2006). A soluble carotenoid protein involved in phycobilisome-related energy dissipation in cyanobacteria. Plant Cell 18, 992–1007. doi: 10.1105/tpc.105.040121
Yang, Y., Yin, C., Li, W., and Xu, X. (2008). Alpha-tocopherol is essential for acquired chill-light tolerance in the cyanobacterium Synechocystis sp strain PCC 6803. J. Bacteriol. 190, 1554–1560. doi: 10.1128/JB.01577-07
Yang, Z., Li, J. L., Liu, L. N., Xie, Q., and Sui, N. (2020). Photosynthetic regulation under salt stress and salt-tolerance mechanism of sweet sorghum. Front. Plant Sci. 10:1722. doi: 10.3389/fpls.2019.01722
Yuan, F., Leng, B. Y., and Wang, B. S. (2016). Progress in studying salt secretion from the salt glands in recretohalophytes: how do plants secrete salt? Front. Plant Sci. 7:977. doi: 10.3389/fpls.2016.00977
Yutthanasirikul, R., Nagano, T., Jimbo, H., Hihara, Y., Kanamori, T., Ueda, T., et al. (2016). Oxidation of a cysteine residue in elongation factor EF-Tu reversibly inhibits translation in the cyanobacterium Synechocystis sp. PCC 6803. J. Biol. Chem. 291, 5860–5870. doi: 10.1074/jbc.M115.706424
Zak, E., Norling, B., Maitra, R., Huang, F., Andersson, B., and Pakrasi, H. B. (2001). The initial steps of biogenesis of cyanobacterial photosystems occur in plasma membranes. Proc. Natl. Acad. Sci. U.S.A. 98, 13443–13448. doi: 10.1073/pnas.241503898
Zhang, D., Jiang, S., Pan, J., Kong, X., Zhou, Y., Liu, Y., et al. (2014). The overexpression of a maize mitogen-activated protein kinase gene (Z m MPK 5) confers salt stress tolerance and induces defence responses in tobacco. Plant Biol. 16, 558–570. doi: 10.1111/plb.12084
Zhang, Q. Y., Wang, L. Y., Kong, F. Y., Deng, Y. S., Li, B., and Meng, Q. W. (2012). Constitutive accumulation of zeaxanthin in tomato alleviates salt stress-induced photoinhibition and photooxidation. Physiol. Plant. 146, 363–373. doi: 10.1111/j.1399-3054.2012.01645.x
Zhao, Y. Q., Ma, Y. C., Duan, H. M., Liu, R. R., and Song, J. (2019). Traits of fatty acid accumulation in dimorphic seeds of the euhalophyte Suaeda salsa in saline conditions. Plant Biosyst. 153, 514–520. doi: 10.1080/11263504.2018.1508090
Zhu, J. K. (2003). Regulation of ion homeostasis under salt stress. Curr. Opin. Plant Biol. 6, 441–445. doi: 10.1016/S1369-5266(03)00085-2
Zhu, Y. H., Graham, J. E., Ludwig, M., Xiong, W., Alvey, R. M., Shen, G. Z., et al. (2010). Roles of xanthophyll carotenoids in protection against photoinhibition and oxidative stress in the cyanobacterium Synechococcus sp. strain PCC 7002. Arch. Biochem. Biophys. 504, 86–99. doi: 10.1016/j.abb.2010.07.007
Keywords: cyanobacteria, membranes lipids, photosynthetic apparatus, photosynthesis, salt stress
Citation: Yang W, Wang F, Liu L-N and Sui N (2020) Responses of Membranes and the Photosynthetic Apparatus to Salt Stress in Cyanobacteria. Front. Plant Sci. 11:713. doi: 10.3389/fpls.2020.00713
Received: 22 February 2020; Accepted: 05 May 2020;
Published: 05 June 2020.
Edited by:
Yoshitaka Nishiyama, Saitama University, JapanReviewed by:
Martin Hagemann, University of Rostock, GermanyKoichiro Awai, Shizuoka University, Japan
Copyright © 2020 Yang, Wang, Liu and Sui. This is an open-access article distributed under the terms of the Creative Commons Attribution License (CC BY). The use, distribution or reproduction in other forums is permitted, provided the original author(s) and the copyright owner(s) are credited and that the original publication in this journal is cited, in accordance with accepted academic practice. No use, distribution or reproduction is permitted which does not comply with these terms.
*Correspondence: Na Sui, suina@sdnu.edu.cn; suina800101@163.com