- Research Center for Environmental Pollution Control Technology, School of Chemical and Environmental Engineering, Hunan Institute of Technology, Hengyang, China
To date, Cd contamination of cropland and crops is receiving more and more attention around the world. As a plant hormone, abscisic acid (ABA) plays an important role in Cd stress response, but its effect on plant Cd uptake and translocation varies among plant species. In some species, such as Arabidopsis thaliana, Oryza sativa, Brassica chinensis, Populus euphratica, Lactuca sativa, and Solanum lycopersicum, ABA inhibits Cd uptake and translocation, while in other species, such as Solanum photeinocarpum and Boehmeria nivea, ABA severs the opposite effect. Interestingly, differences in the methods and concentrations of ABA addition also triggered the opposite result of Cd uptake and translocation in Sedum alfredii. The regulatory mechanism of ABA involved in Cd uptake and accumulation in plants is still not well-established. Therefore, we summarized the latest studies on the ABA synthesis pathway and comparatively analyzed the physiological and molecular mechanisms related to ABA uptake, translocation, and detoxification of Cd in plants at different ABA concentrations or among different species. We believe that the control of Cd uptake and accumulation in plant tissues can be achieved by the appropriate ABA application methods and concentrations in plants.
Introduction
An increasing amount of heavy metals have been released into the environment on account of anthropogenic activities, and these heavy metals are posing a serious impact on crop yields, ecosystem functions, and human health, thus causing significant expense to the global economy (Vareda et al., 2019). Among the heavy metals, cadmium (Cd) ranks as one of the top toxic substances, owing to its high mobility, biotoxicity, and prevalence (Zhang et al., 2020). Cd is prone to accumulate in plants through root uptake and further threatens human health through the food chain (Wang et al., 2017). To date, Cd contamination of cropland and crops has received increasing attention worldwide. Therefore, measures are urgently needed to reduce the serious problems caused by Cd pollution.
Over the past few decades, numerous reports on the uptake, translocation and detoxification of Cd in plants have been published, covering crops such as potato (Ye et al., 2020), sweet potato (Huang et al., 2019), maize (Mahmoud et al., 2021), and rice (Yang J. et al., 2020), as well as model plants such as Arabidopsis thaliana (Szopiński et al., 2020) and tobacco (Siemianowski et al., 2014). There are various methods to control Cd accumulation in plants, including: (1) Agronomic methods (through plant rotation or co-cropping, etc.) (Rizwan et al., 2016); (2) Genetic engineering (by altering the expression of certain genes) (Wang et al., 2019; Raza et al., 2020); (3) Pollution safe cultivars (by screening low Cd accumulation cultivars) (Huang et al., 2017; Wang et al., 2021); (4) Soil remediation strategy (through the application amendments or physicochemical methods to control the Cd bioavailability) (Hamid et al., 2020; Bilias et al., 2021). For the better implementation of these methods, it is valuable to study the molecular mechanisms related to Cd uptake and accumulation in plants.
Abscisic acid (ABA), a water and ether soluble hormone, was first identified in potatoes and shown to inhibit the growth of buds (Hemberg, 1949). It was later found that ABA can control the abscission of cotton fruits, which is the origin of the name of ABA (Addicott et al., 1968). Recent studies have established that ABA is closely related to abiotic stresses such as drought (Lim et al., 2015), salinity (Hussain et al., 2021), nutrient deficiency (Cutler et al., 2010), and heavy metals (Hu et al., 2020). According to certain studies, ABA was found to promote the uptake and translocation of Cd in plants (Wang et al., 2016; Liu L. et al., 2017; Chen et al., 2021), while other studies showed that ABA inhibited Cd uptake and translocation (Tao et al., 2019; Zhang P. et al., 2019; Leng et al., 2021). To date, the mechanism of ABA in response to the Cd stress of plants is still not well-established. Therefore, it is crucial to reveals some insights into the regulatory mechanism of ABA on Cd uptake and translocation in plants. Although considerable reviews on ABA and heavy metal stress in plants are available, reviews addressing the effects of ABA on plant Cd uptake and accumulation are still rare. Here, we mainly summarize the latest studies on ABA regulatory pathways, the molecular and physiological mechanism of ABA’s effect on plant Cd uptake and translocation, as well as the functions of ABA in plant Cd stress response. It is expected that a reasonable explanation can be put forward for the different effects of ABA on plants Cd uptake and translocation. Also, we are awaiting this review to provide help for the strategies on low-Cd crops cultivation and the phytoextraction technology of soil remediation.
Synthesis, metabolism, and transport of abscisic acid
Abscisic acid is one of the secondary metabolites of plants, which is synthesized from carotene through the interaction of various enzymes along multiple regulatory pathways. Zeaxanthin is an important intermediate for carotenoids to generate ABA and can be synthesized in two pathways (Vishwakarma et al., 2017; Chen et al., 2020; Figure 1). The first pathway is the conversion of β-carotene to zeaxanthin by β-carotene hydroxylase, and the second is the reversible reaction of converting violaxanthin to antheraxanthin and then to zeaxanthin. With the catalysis of zeaxanthin epoxidase (ZEP), zeaxanthin could be converted to all-trans-violaxanthin. The downstream products of all-trans-violaxanthin are divided into two pathways, one is catalyzed by an indeterminate enzyme to produce 9′-cis-violaxanthin, the other is that all-trans-violaxanthin is enzymatically converted into trans-neoxanthin under the process of ABA-deficient 4 (ABA4) and subsequently transformed to 9′-cis-neoxanthin with the help of another uncertain enzyme (Schwartz et al., 1997). Under the oxidative cleavage of 9-cis-epoxycarotenoid dioxygenase (NCEDs), both 9-cis-neoxanthin and 9-cis-violaxanthin are modified to xanthoxin and transported out of the plastid. In cytoplasm, the synthesis of ABA takes place in only two steps, the first step is to reform xanthoxin into ABA-aldehyde at the present of ABA-deficient 2 (ABA2), and the second step is to alter ABA-aldehyde into ABA through the mediation of ABA-aldehyde oxidase 3 (AAO3) (Bittner et al., 2001).
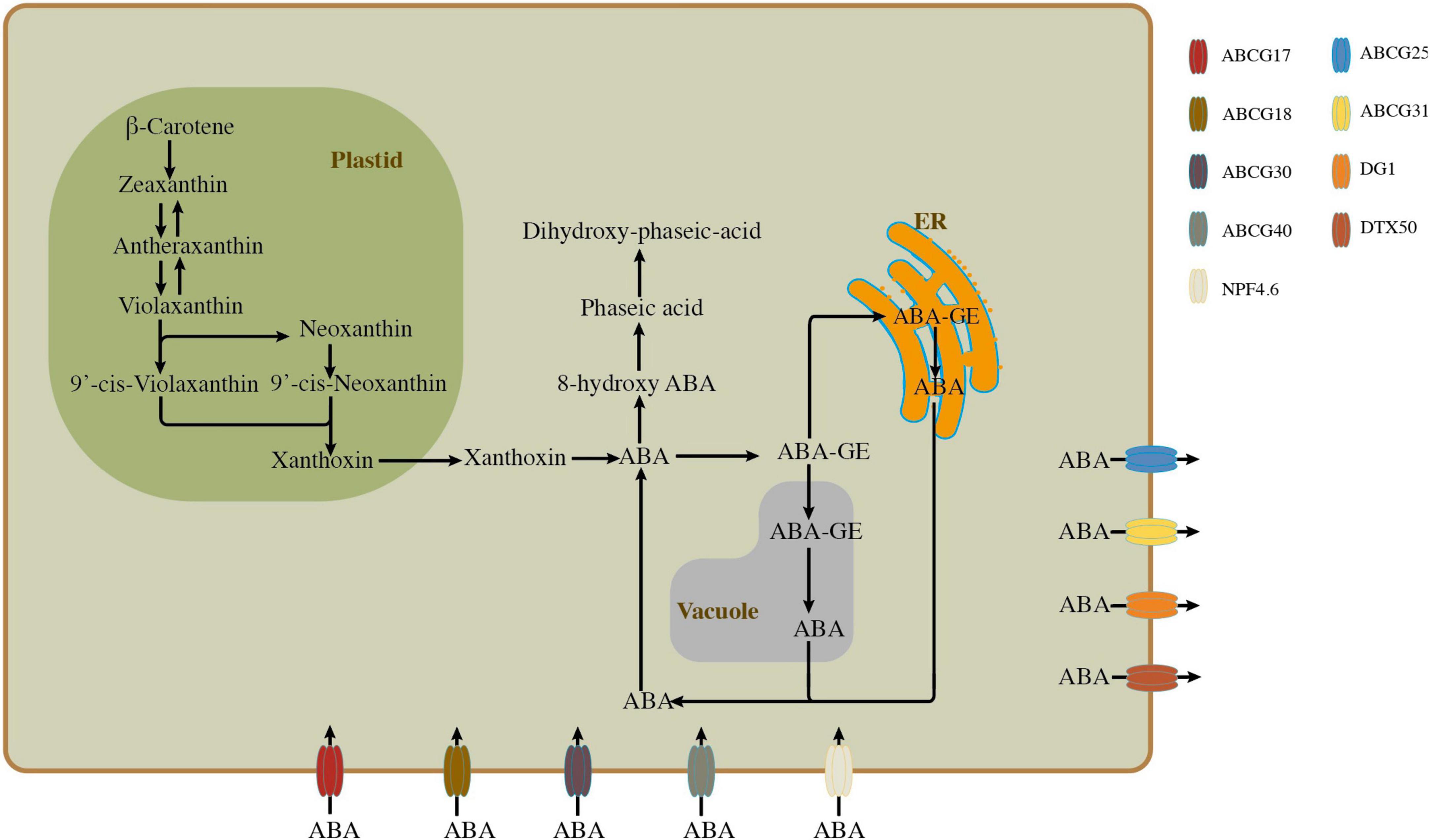
Figure 1. Overall scheme for abscisic acid (ABA) biosynthesis, catabolism, and transport in plant. The pathways of ABA biosynthesis, catabolism, and directions of ABA transport are shown with black arrows. The ABA transporter proteins located on the plasma membrane are represented by different colors.
Regarding the metabolic pathway of ABA, it is relatively simple. The surplus ABA can be inactivated and preserved in vacuole and endoplasmic reticulum through the formation of ABA-glucose ester, a process catalyzed by UDP-glucosyltransferase (UGT) encoded by UGT71C5 (Liu et al., 2015). Also, when ABA deficiency occurs, the preserved ABA-glucose ester in vacuole and endoplasmic reticulum gets activated and released into the cytoplasm via β-glucosidases, which is encoded by Arabidopsis thalianaβ-glucosidase (AtBG1 and AtBG2), respectively. In addition to being inactivated and preserved, ABA can also be converted into phaseic acid (PA), then to dihydrophaseic acid (DPA), and finally to DPA-4-O-β-D-glucoside (DPAG) at the presence of cytochrome P450 monooxygenase (P450), PA reductase (PAR), and glycosyltransferase (GT), respectively (Vishwakarma et al., 2017; Chen et al., 2020; Figure 1).
Abscisic acid transportation is often observed in plants to regulate plant growth and development or in response to various stresses. The long-distance transport of ABA between the shoot and root of plants is achieved through xylem and phloem (Kuromori et al., 2014). The xylem is mainly responsible for the transport of ABA from root to shoot, while the phloem accountable for ABA transport in the opposite direction, and the amount of ABA transported by the xylem is considerably higher than that of the phloem (Peuke, 2016; Anfang and Shani, 2021). Short-distance transport of ABA happens mainly between cells, including vascular cells and guard cells, etc. The identification of a number of ABA transporters contributes to the investigation of ABA transport mechanisms. In plants, ABA transporters such as ABCG25 (ATP-binding cassette transporters), ABCG31, DG1 (multidrug and toxin efflux transporter), and DTX50 (multidrug and toxin efflux transporter) are responsible for the efflux of ABA, while ABCG17, ABCG18, ABCG30, ABCG40, and NPF4.6 (nitrate transporter 1/peptide transporter) are involved in the import of ABA (Kang et al., 2010, 2015; Kuromori et al., 2010; Kanno et al., 2012; Zhang et al., 2014; Anfang and Shani, 2021; Figure 1).
Cd stress is similar to other abiotic stresses, such as drought and ultraviolet rays, etc., which can induce the level of endogenous ABA synthesis in plants (Guo et al., 2019). Plants rely on ABA to mimic the impacts of stressful situations and may continuously modify the endogenous ABA contents in response to environmental stresses (Vishwakarma et al., 2017). In addition, the effects of ABA on plant Cd uptake, translocation, and Cd tolerance have been investigated in numerous studies by applying exogenous ABA (Wang et al., 2013; Tao et al., 2019; Hu et al., 2020). Therefore, the molecular and physiological mechanisms of the effects of ABA on plant Cd uptake, translocation and tolerance will be discussed below.
Abscisic acid affects Cd uptake by regulating signaling pathways and root development
Root uptake is the most important way for the entry of Cd into plants, and ABA manages to influence Cd accumulation in plants by affecting the Cd uptake of root (Yang Y. et al., 2020; Shen et al., 2022). Through the review of numerous studies, it was demonstrated that the effect of ABA on Cd uptake of plant roots is not constant (Table 1). For some plant species and cultivars, ABA significantly promotes Cd uptake, while for other species and cultivars, it exhibits an inhibitory effect on Cd uptake. In addition, the difference in the concentration of ABA treatment might either promote or inhibit the uptake of Cd in some specific plant species and cultivars (Table 1).
It was shown that the application of ABA providing a feasible solution for the reduction of Cd uptake in plant roots. When subjected to 1.5 mM of Cd treatment, the endogenous ABA concentration was considerably elevated in Cd-tolerant Oryza sativa (rice) cultivar (TNG67), but no significant changes were observed in the Cd-sensitive rice cultivar (TN1). And the increase of endogenous ABA biosynthesis level can reduce the Cd uptake of rice (Hsu and Kao, 2008). After pretreated with 5 mM ABA, Cd accumulation in TN1 was significantly reduced by 44%, whereas no significant change of Cd accumulation was observed in TNG67 (Hsu and Kao, 2003). The application with 10 μM of ABA not only markedly reduced (p < 0.05) the Cd contents in the roots and stems of Vigna radiata (mung bean) seedlings by 23.5 and 25.3% respectively, but also significantly elevated (p < 0.05) the chlorophyll and carotenoid content (Leng et al., 2021). In species such as Brassica chinensis, Populus euphratica, Lactuca sativa, and Solanum lycopersicum, ABA content was also negatively correlated with the Cd accumulation levels (Han et al., 2016; Pompeu et al., 2017; Pan et al., 2019; Tang et al., 2020). Only the mechanisms of Brassica chinensis and Populus euphratica were explicated as that ABA elevated antioxidant enzyme activity and reduced the intracellular H2O2 content by enhancing the antioxidant capacity of plants, which was responsible for regulating the entry of Cd through the calcium ion channel, thus leading to a reduction in the level of Cd uptake (Han et al., 2016; Pan et al., 2019). However, in Solanum photeinocarpum and Boehmeria nivea, ABA enhanced the Cd uptake mainly by increasing the chlorophyll level and biomass of the plants (Wang et al., 2016; Chen et al., 2021). Apart from species, different ABA concentrations showed different effects on the Cd uptake capacity of the same plant. In Boehmeria nivea and Solanum photeinocarpum, the strongest Cd uptake was observed at the application of 5 and 20 μM of ABA, respectively, however, the relevant mechanisms involved are not clearly explored (Wang et al., 2016; Chen et al., 2021). Although application of ABA also promoted Cd uptake of Bidens Pilosa, it diminished the biomass and chlorophyll content (Liu L. et al., 2017), which was the exact contrast to its effect on stimulating the growth of Solanum photeinocarpum and Boehmeria nivea and other spices.
Therefore, further studies are needed to fully reveal the mechanism by which ABA affects Cd uptake among species, and whether ABA has any effect on root Cd uptake by impacting root development also needs to be investigated.
Molecular mechanism of abscisic acid affecting Cd uptake among species
As a model plant, Arabidopsis thaliana has been well-studied for the ABA effect on Cd uptake and accumulation. Firstly, application of exogenous ABA elevated the ABA levels in Arabidopsis thaliana and simultaneously suppressed the expression of iron-regulated transporter 1 (IRT1), a key transporter responsible for Cd uptake in roots, thereby leading to the reduction of Cd uptake and accumulation in Arabidopsis thaliana (Vert et al., 2002). Under Cd stress, ABI5 could bind to the transcription factor MYB49 and block the function of the bHLH38 and bHLH101 promoters that required for IRT1 expression, thereby reducing IRT1 concentration (Wu et al., 2012; Wang et al., 2013). Zhang et al. (2019) revealed that the application of ABA stimulated the expression of ABI5 and thus reduce the Cd uptake in Arabidopsis thaliana by regulating the expression level of IRT1. ABA-importing transporters (AIT1) contributed to the entry of ABA into the plant roots and then inhibited the expression of IRT1, thus limiting the uptake of Cd of Arabidopsis thaliana (Pan et al., 2020). Furthermore, inoculation with ABA-generating bacteria strains and ABA-catabolizing bacteria strains resulted in corresponding increases and decreases of ABA levels in Arabidopsis thaliana, respectively, which in turn inhibited or promoted Cd uptake and accumulation by affecting the expression levels of IRT1 in Arabidopsis thaliana (Xu et al., 2018; Lu et al., 2020a). In addition, some transgenes and mutant experiments on Arabidopsis thaliana have investigated the functions of ABA synthesis-related genes or ABA signaling-related genes in Cd uptake and accumulation. Multiple studies using Arabidopsis thaliana transgenic plants and mutants have demonstrated that ABA synthesis-related genes (ABA-1, ABA-3, ABA-4, and nced3) and ABA signaling-related genes (ABI2, ABI3, ABI5, SnRK2s, and PP2Cs) are involved in Cd uptake and accumulation (Umezawa et al., 2009; McLoughlin et al., 2012; Zhang et al., 2019). In general, the accumulation of Cd in Arabidopsis thaliana was negatively correlated with the concentration of ABA (Figure 2).
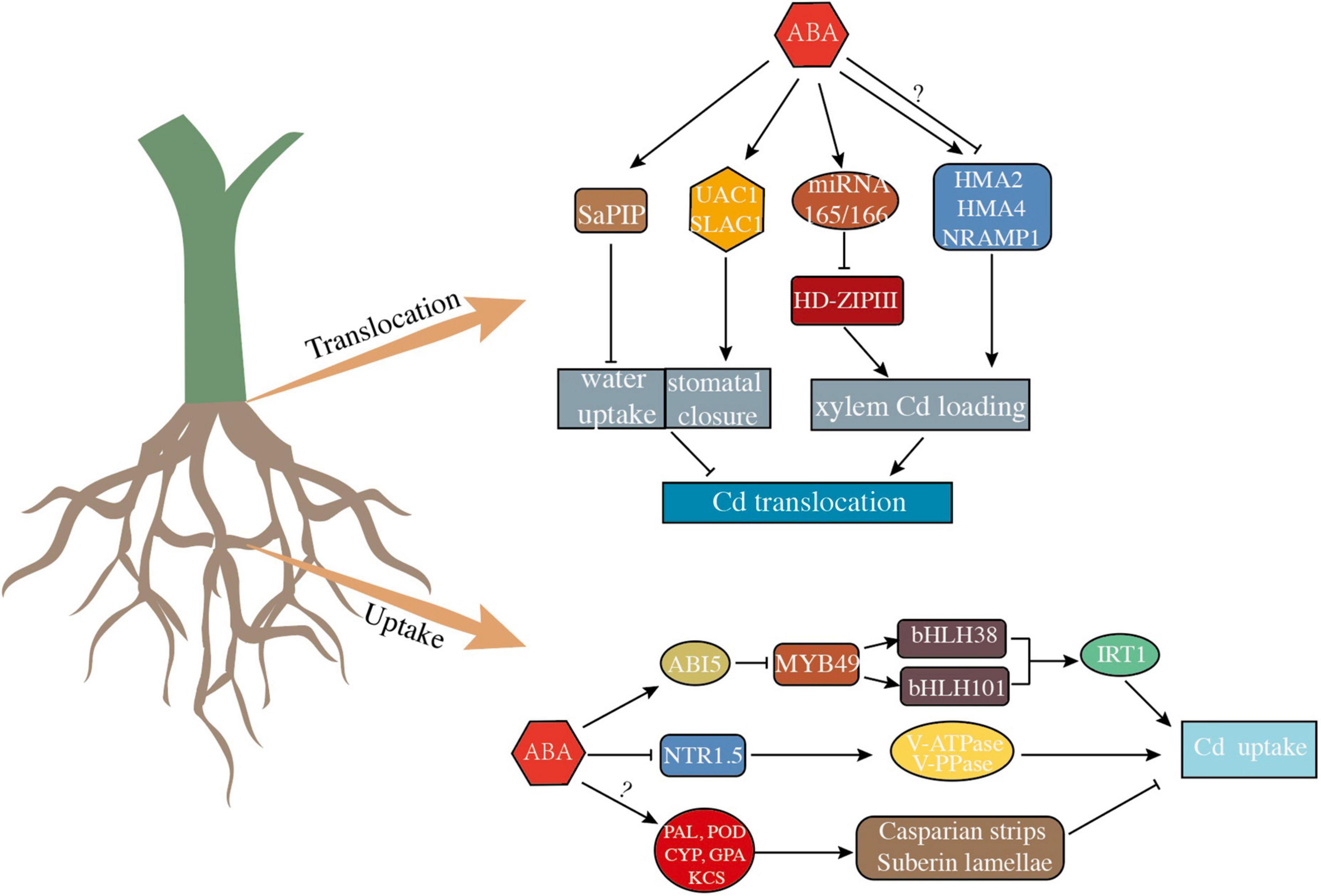
Figure 2. A proposed model of the effect of abscisic acid (ABA) on Cd uptake and translocation in plants. Arrows indicate positive regulation of ABA and its corresponding targets, bars indicate negative regulation of ABA and its corresponding targets, question marks represent regulatory pathways that remain unclear.
In Sedum alfredii, ABA showed opposite effects on plant Cd uptake in different experiments. On one hand, ABA inhibited the uptake of Cd, unlike non-hyperaccumulating ecotype (NHE) in which the endogenous ABA content was significantly elevated, the endogenous ABA in hyperaccumulating ecotype (HE) remained at a relatively minimum level in response to Cd stress. The elevated endogenous ABA level should be attributed to the significant up-regulation of ABA2 and NECD expression levels of NHE under Cd treatment (Tao et al., 2019, 2021). Studies firmly indicate that the root apical zone is the most dynamic part for Cd uptake (Lux et al., 2011; Redjala et al., 2011). Casparian strips (CSs) and suberin lamellae are important barriers preventing Cd from entering the plant root via the apoplastic pathway (Vaculík et al., 2009; Ricachenevsky et al., 2018). The distance from the CSs to the root tip (LTIP-CSs) and the proportion of the suberin lamellae deposited in the root were significantly related to the ability of Cd uptake by plants. Studies have shown that ABA exerts a catalytic effect on suberin biosynthesis and deposition (Barberon et al., 2016; Tao et al., 2017). By confocal laser scanning microscope, Tao et al. (2019) found that the LTIP-CSs and non-suberized portions in the root were statistically inversely correlated with the root ABA content, indicating that ABA reduced Cd uptake by regulating the development of apoplastic barriers in roots of NHE. ABA may enhance the deposition of CSs and suberin by elevating the expression of PAL, POD, SaCYP86A1, SaGPAT5, and SaKCS20 that related to CSs and suberin synthesis in Sedum alfredii. Du et al. (2022) demonstrated that ABA-catabolizing bacterium R. qingshengii was able to enhance the expression of Cd uptake related transporters by reducing the ABA content in Sedum alfredii, thus enhancing the Cd uptake capacity. On the other hand, it is interesting to note that the study of Chen et al. (2022) and Lu et al. (2020b) on Cd uptake of Sedum alfredii. Conflicted with the previous studies. It was found that Cd uptake of Sedum alfredii was significantly enhanced by foliar spraying of ABA, which was attributed to the fact that ABA enhanced the expression of HsfA4c and NAS thus promoted the tolerance and uptake of Cd by plants. Such a contradictory situation of Sedum alfredii should ascribe to the difference in ABA application methods and concentrations (Figure 2).
Application of exogenous ABA inhibited the Cd uptake in Brassica napus, which was due to the inhibition of NTR1.5 (a long-distance transporters of NO3–) expression by ABA, causing a decrease in proton pump activity of V-ATPase and V-PPase, which resulted in lower Cd uptake in the roots (Wang et al., 2018). It is intriguing that endogenous ABA in Brassica napus is just the contrary of exogenous ABA, which affects the function of proton pump to enhance root Cd accumulation, but the specific mechanism still needs to be further investigated. Cd tolerant bacterium NC16 could also elevate the ABA content and subsequently diminish the Cd uptake of Zea mays by inhibiting the expression of a zinc/iron transporter (Zhou et al., 2019; Figure 2).
The effects of abscisic acid on Cd uptake through regulating root development
The root system is the main passage for Cd to enter plants, therefore, the relationships between Cd uptake and root morphology among different plant species have been widely investigated (Ge et al., 2016; Deng et al., 2020; Guo et al., 2020). Variations in root morphology and structure may affect Cd uptake and xylem loading, leading to differences in the Cd accumulation and distribution among plant tissues (Vaculík et al., 2012). In the hyperaccumulating ecotype of Sedum alfredii, the root length, area, and volume were improved under Cd treatment, which exhibited a significant and positive correlation with its Cd uptake and accumulation (Li et al., 2009). The comparative study of high- and low-Cd cultivar of pakchoi, tomato, hot pepper, peanut, and wheat revealed that high-Cd cultivar possessed longer root length, more root tips, larger root surface area, and greater root volume, which should contribute to its higher Cd uptake and translocation (Kubo et al., 2011; Zhang et al., 2013; Huang et al., 2015; Xia et al., 2016; Xin et al., 2017). Through the study of 18 herbaceous plants, Yu et al. (2017) observed that longer and slimmer roots facilitated the translocation of Cd from to shoot, while coarser roots (diameter of 0.6–0.8 mm) contributed to the immobilization of Cd in roots and thus inhibited its translocation to shoot.
According to the review of Harris (2015), in which ABA was ascribed as “Hidden Architect of Root System Structure,” indicating the crucial role of ABA in root development. ABA regulates root development mainly by working on stem cells and controlling the activity of quiescent centers in the root meristem, and it has been widely accepted that low concentrations of ABA promote root development, while high concentrations inhibit root development (Sun et al., 2018; Brookbank et al., 2021). The root growth promotion at low concentration of ABA was mainly achieved through the regulation of auxin pathway and auxin efflux carrier (PIN2/EIR1), while the root inhibition by high concentration of ABA was mediated by the combination of auxin efflux protein (AUX1) and ethylene pathway (Li et al., 2017; Brookbank et al., 2021). In addition, ABA is also observed to inhibit root development by evoking ROS and elevating cytoplasmic Ca2+ content in root (Jiao et al., 2013; Tsukagoshi, 2016). However, the sensitivity of different plants to ABA varies according to their growth environment and genetic diversity, therefore the same concentration of ABA may promote root development in some plants but inhibit root development in others. Concentration of ABA amounting to 1 μM was sufficient to inhibit lateral root (LR) development in Arabidopsis thaliana, whereas for M. truncatula, ABA concentrations in the range of 0.1–10 μM were found to promote LR development, and LR formation was inhibited only at ABA concentrations above 50 μM (Ariel et al., 2010; Gonzalez et al., 2015). According to the study of Chen et al. (2006), ABA is able to promote lateral root production in rice like it does in most legumes including M. truncatula, and the calcium, calmodulin and de novo protein synthesis are required during the stimulation process.
Through statistical analysis of studies related to plant root development and Cd uptake capacity, we discovered that the level of Cd accumulation in plants under the effect of ABA was not necessarily positively correlated with their root development. In some plant species, ABA is capable of enhancing the level of root development, while reducing the ability of plants to accumulate Cd. The application of 0.5 μM ABA increased root biomass by 16%, but resulted in a 27% decrease in Cd uptake and accumulation in Arabidopsis thaliana (Fan et al., 2014; Pan et al., 2020). The use of 10 μM ABA also significantly increased root biomass and decreased Cd content in mung bean (Leng et al., 2021). Besides, abscisic acid-deficient sit tomato mutant showed stunted root development and significantly increased Cd content (Pompeu et al., 2017). When co-cultured with ABA-generating bacteria, biomass was significantly increased and Cd content was also decreased in pakchoi (Pan et al., 2019). In Sedum alfredii, the higher ABA concentration (0.2 mg/L) inhibited the root biomass, but promoted the uptake and accumulation of Cd (Lu et al., 2020b). However, in other plants, such as ramie (Boehmeria nivea L.), purple flowering stalk and Solanum poteinocarpum, lower level of ABA can simultaneously promote root development and Cd uptake and accumulation (Wang et al., 2016; Shen et al., 2017; Chen et al., 2021). In addition, the significantly root biomass improvement and higher Cd uptake capacity were observed in Sedum alfredii with the application of ABA-catabolizing bacterium Rhodococcus qingshengii (Wu et al., 2020; Du et al., 2022).
Therefore, ABA affects the development of plant roots through auxin and ethylene regulatory pathways, and a large number of studies have shown that the development of plant roots helps plants to improve Cd uptake to a certain extent. However, the effect of ABA on plant Cd uptake capacity is not only achieved by the level of root development, but also depends on various factors such as the effect of ABA on the expression level of Cd-related transporters.
Abscisic acid influences Cd translocation by regulating transpiration, xylem loading, and Cd transporters
In general, the effect of ABA on plant Cd translocation is basically similar to its effect on plant Cd uptake capacity. To date, ABA is known to regulate Cd translocation in plants in three ways: (1) affecting plant transpiration; (2) xylem loading (apoplast pathway); (3) regulating the expression of Cd transporters (Mendoza-Cózatl et al., 2011; Zhao and Wang, 2020; Khanna et al., 2022).
Transpiration pull is the main driving force for Cd transport from roots to shoots in plants, and studies have shown that the difference in Cd translocation among different plants is mediated by the changes of transpiration rates (Uraguchi et al., 2009; Khanna et al., 2022). Plants have been found to regulate transpiration by controlling root water uptake (Cai et al., 2022), regulating xylem conduits (Schenk et al., 2021) and moderating the density and closure of stomata (Agurla et al., 2018; Tao et al., 2021). ABA was revealed to regulate plant stomatal closure through kinase-mediated phosphorylation of guard cell membrane-localized transporters, such as R-type anion channel QUAC1 and S-type anion channel SLAC1, which should hinder the translocation of metal ions from root to shoot (Pornsiriwong et al., 2017; Hsu et al., 2021). According to the study of Kuromori et al. (2016), overexpression of ABA transporter (AtABCG25) in Arabidopsis thaliana resulted in stomatal closure and reduced transpiration. In Sedum alfredii, ABA regulates the transpiration rate by decreasing the expression of root aquaporin (SaPIP), stomatal density and size, thereby leading to the reduction of Cd translocation from root to shoot (Tao et al., 2021). Likewise, transpiration in rice was reduced by 64–72% under 100 mM ABA treatment, while the shoot Cd content was correspondingly reduced by about 10-fold (Hsu and Kao, 2003). When pretreated with 5 μM ABA, Cd concentrations in shoots of Phytolacca americana were significantly reduced, which was attributed to the inhibition of transpiration (Liu et al., 2010). In lettuce, studies have also shown that exogenous ABA can reduce Cd transport by inhibiting transpiration (Aroca et al., 2008; Dawuda et al., 2020). Therefore, it is speculated that ABA mainly regulates Cd translocation in plants by inhibiting transpiration, however, most of the studies are only at the physiological level, and the related molecular mechanisms remain to be investigated.
Root xylem loading of Cd is one of the most important steps for its translocation to shoot, and the Cd concentration in xylem sap is significantly and positively correlated with Cd concentration of shoot (Uraguchi et al., 2009; Mendoza-Cózatl et al., 2011; Song et al., 2017). The miRNA165 and miRNA166 are known signaling molecules that determine the development of xylem cells in plant stele (Carlsbecker et al., 2010; Miyashima et al., 2011). In endodermis, ABA elevated the expression level of miRNA165, which in turn negatively affected HD-ZIPIII TF (transcription factors in the stele) levels and led to higher xylem hydrophobicity (Ryu et al., 2016; Ramachandran et al., 2018). According to the study of Bloch et al. (2019) in Arabidopsis thaliana and tomato, ABA was revealed to affect HD-ZIPIII levels by upregulating miRNA165a/166b expression, enhancing xylem lignin deposition, and limiting lateral root formation and elongation, which apparently reduced Cd loading into xylem of the plant root. Moreover, the master regulators for xylem differentiation, VASCULAR-RELATED NAC DOMAIN (VND) 1-3 and 7, are regulated by ABA in response to environmental stress (Ramachandran et al., 2021). Strikingly, the reduction of miRNA165/166 levels in Arabidopsis thaliana plants by using artificial miRNA-target (STTM165/166) resulted in elevated expression of ABA and its related genes (Yan et al., 2016), whereas application of STTM166 did not affect ABA content in rice (Zhang et al., 2018), suggesting the signaling pathways and functions of miRNA166 are inconsistent between rice and Arabidopsis thaliana. Furthermore, ABA was observed to have no significant effect on plant xylem vessel length and diameter, but it greatly reduced the vessel count of xylem, limiting Cd loading and transpiration, thereby inhibiting Cd transport to shoot (Campbell et al., 2018; Tao et al., 2021). Studies on ABA regulation of xylem differentiation and Cd loading are relatively rare, therefore the future studies concerning the effect of ABA on Cd transport in plants through regulation of miRNA166 and VND will be interesting.
Membrane transporters serve a critical function in the loading of Cd into root vascular tissue and subsequent translocation in the plants (Zhao and Wang, 2020). ABA was shown to induce the expression of a number of Cd transporters, thereby influencing Cd translocation in plants (He et al., 2020). The heavy metal ATPases HMA2 and HMA4, localized on the plasma membrane, contribute to the xylem Cd loading, thus facilitating the translocation of Cd from root to shoot (Papoyan and Kochian, 2004; Takahashi et al., 2012). NRAMP1 (resistance-associated macrophage protein 1) contributes to the translocation of Cd from root to shoot as well (Yang Y. et al., 2020). In contrast, HMA3, localized on the tonoplast, is able to promote the sequestration of Cd in vacuole and inhibit the translocation of Cd in plants (Liu H. et al., 2017; Begum et al., 2019). However, there are few studies on Cd transporters involved with ABA treatment showed inconsistent results. Chen et al. (2022) and Lu et al. (2020b) demonstrated that endogenous ABA promoted the loading of Cd into the xylem by inducing the expression of HMA2 and HMA4, thus elevating the Cd content in the shoot of S. alfredii and leading to increased Cd translocation factor (TF) values. The transcription factor NAC895 was able to elevate the loading of Cd into the xylem by promoting the expression of HMA2 in the presence of ABA (Liu et al., 2022). Under the gradient treatment of ABA from 0 to 40 μmol/L, the TF values of Cd were maximally elevated by 16.8 and 11.4% in Solanum photeinocarpum and Bidens Pilosa, respectively, however, the specific mechanism by which ABA enhanced the Cd translocation was not interpreted (Wang et al., 2016; Liu L. et al., 2017). Conversely, ABA-induced reduction of Cd translocation in Arabidopsis can be achieved through overexpression of MhNCED3 and inhibition of HMA2 and NRAMP1 expression levels (Pan et al., 2019). When cultured with ABA-degrading bacteria, the expression of HMA2, HMA3, and HMA4 were up-regulated and resulted in elevated Cd content in the shoot of Arabidopsis thaliana (Lu et al., 2020a).
In conclusion, the role of ABA on Cd translocation by reducing transpiration and decreasing xylem length, number and hydrophilicity is relatively clear and consistent, but the function of ABA in regulating the expression of Cd transporters varies widely. We propose that the translocation of Cd should be achieved through the combination of multiple aspects, rather than explained in only one aspect. Therefore, some indepth research work is still needed to investigate how ABA regulates the translocation of Cd from roots to shoots (Figure 2).
Abscisic acid enhances Cd tolerance by regulating antioxidant systems
Reactive oxygen species (ROS), including superoxide anion (O2–), hydrogen peroxide (H2O2), singlet oxygen (1O2), and hydroxyl radical (OH•), is a kind of oxygen-containing molecules, which are mainly produced by transferring electrons to molecular oxygen (O2) during photosynthesis and aerobic respiration in plants (Qi et al., 2017; Waszczak et al., 2018). ROS acts as signaling molecules, which participate in plant growth, development, and adaptation to biotic and abiotic stresses (Baxter et al., 2014; Choudhury et al., 2017). However, heavy metals (such as Cd) will lead to the surge of ROS, which can cause oxidative damage to cellular biological macromolecules (including lipid, proteins, DNA, and RNA), even resulting in cell death (Mittler, 2002; Shahid et al., 2014; Hu et al., 2016). Therefore, maintaining ROS homeostasis has great importance for plants to adapt to stresses (Miller et al., 2010).
Abscisic acid (ABA) plays key roles in plant tolerance to multiple stresses, including salinity, drought, and heavy metals (Bari and Jones, 2009; Hauser et al., 2017; Mega et al., 2019; Hu et al., 2020; Tao et al., 2021). Many studies showed that Cd stress promoted the production of endogenous ABA, and exogenous ABA increased Cd tolerance through scavenging Cd-induced ROS by regulating antioxidant enzymes and/or non-enzymatic constituents in plants. In tomato (Solanum lycopersicum), the ABA-deficient mutant was less tolerant to Cd stress and displayed higher antioxidant activity than its wild type, suggesting that the responses to Cd stress in tomato was enhanced by ABA deficiency (Pompeu et al., 2017). Exogenous ABA application reduced malondialdehyde (MDA), H2O2, and O2– contents in purple flowering stalk (Brassica campestris L. ssp. chinensis) by activating the antioxidant enzymes, including superoxide dismutase (SOD), peroxidase (POD), ascorbate peroxidase (APX), and glutathione reductase (GR), thus relieving Cd toxicity (Shen et al., 2017). In a Cd-sensitive cultivar of lettuce (Lactuca sativa L.), foliar application of ABA alleviated Cd-induced oxidative damage by increasing SOD, catalase (CAT), POD activities, and chlorophyll and carotenoids contents (Dawuda et al., 2020). ABA reduced Cd-elicited H2O2 by increasing CAT, POD, and APX activities, contributing to the improved Cd tolerance of Cd-hypersensitive Populus euphratica (Han et al., 2016). Foliar application of ABA increased Cd tolerance of mung bean seedlings [Vigna radiata (L.) R. Wilczek] and largely recovered Cd-induced changes in antioxidant systems, including APX, CAT, and POD activities, MDA, proline, and ascorbic acid (AsA) contents (Leng et al., 2021). Under 100 μM Cd treatment, 0.2 mg/L ABA promoted the growth of Sedum alfredii Hance (a Cd hyperaccumulator) and reduced its MDA content (Lu et al., 2020b).
Several studies have shown that ABA affects antioxidant enzymes by regulating transcription factors (TFs), such as WRKYs (Hong et al., 2016). WRKYs participate in the signal transduction processes responding to stresses by binding to the WRKY binding sites (W-boxes, C/TTGACC/T) in the promoters of the defense-related genes and could be regulated by ABA (Eulgem and Somssich, 2007; Yang et al., 2016). Several studies have proved that WRKYs are key links in ABA signaling networks and play crucial roles in plant responses to different stresses by maintaining ROS homeostasis (Chen et al., 2010; Rushton et al., 2012; Sun et al., 2022). For instance, the overexpression of ZmWRKY4 in maize (Zea mays) increased the levels and activities of SOD and APX, while the RNAi of ZmWRKY4 inhibited the increase of SOD and APX expression levels and activities induced by ABA, suggesting that ZmWRKY4 was necessary for ABA regulating antioxidant enzyme systems under stress (Hong et al., 2016). In Tamarix hispida, ThWRKY4 was highly induced by ABA, drought and salt stresses, and overexpression of ThWRKY4 decreased ROS (O2– and H2O2) levels in Arabidopsis thaliana by increasing SOD and POD activities (Zheng et al., 2013). Over expression of Tamarix hispida ThWRKY7 significantly enhanced Cd tolerance in Arabidopsis thaliana by increasing SOD and POD activities (Yang et al., 2016).
Conclusion and perspective
In this review, we analyzed and summarized the anabolism of ABA, effect of ABA on the Cd uptake and translocation, correlation between ABA on plant root development and Cd accumulation, and the effect of ABA on ROS mitigation in plants. We believe that the Cd uptake and translocation affected by ABA is closely related to plant species, the application methods and concentrations of ABA. In most cases, ABA serves the inhibitory function of Cd uptake mainly through: (1) regulating the ABI5-MYB49-IRT1 pathway; (2) facilitating the deposition of CSs and suberin lamellae; (3) suppressing the proton pump activity of V-ATPase and V-PPase by inhibiting the expression of NTR1.5. The effect of ABA on Cd translocation is primarily through: (1) inhibiting transpiration by modulating the phosphorylation of QUAC1 and SLAC1, the expression of SaPIP and stomatal density and size; (2) elevating xylem hydrophobicity by affecting expression levels of miRNA165/166, HD-ZIPIII TF, and VND; (3) regulating the expression Cd transporters such as HMA2, HMA3, HMA4, and NRAMP1. ABA is capable of affecting root development through auxin and ethylene pathway, but its correlation with plant Cd uptake and accumulation capacity is not significant. Since the effects of ABA on Cd uptake and translocation vary distinctly among species and the concentrations of ABA, future work should focus on: (1) mechanistic differences between endogenous and exogenous ABA on Cd uptake and accumulation in plants; (2) mechanisms underlying the effects of exogenous ABA (including concentration and application method) on the differences in Cd uptake and accumulation among species; (3) application of ABA for low cadmium accumulation in crops to ensure food safety and for high cadmium uptake in phytoremediation.
Author contributions
CS and Y-YH conceptualized the review, wrote the manuscript, and prepared the figures. Y-MY, Y-FS, MZ, and X-JC helped to collect data and write the manuscript. All authors contributed to the article and approved the submitted version.
Funding
This research was supported by National Natural Science Foundation of China (Grant No. 42007144) and Scientific Research Foundation of Hunan Provincial Education Department (Grant No. 20C0576).
Conflict of interest
The authors declare that the research was conducted in the absence of any commercial or financial relationships that could be construed as a potential conflict of interest.
Publisher’s note
All claims expressed in this article are solely those of the authors and do not necessarily represent those of their affiliated organizations, or those of the publisher, the editors and the reviewers. Any product that may be evaluated in this article, or claim that may be made by its manufacturer, is not guaranteed or endorsed by the publisher.
References
Addicott, F. T., Lyon, J. L., Ohkuma, K., Thiessen, W. E., Carns, H. R., Smith, O. E., et al. (1968). ‘Abscisic acid: a new name for abscisin II (dormin)’. Science 159:1493. doi: 10.1126/science.159.3822.1493-b
Agurla, S., Gahir, S., Munemasa, S., Murata, Y., and Raghavendra, A. S. (2018). ‘Mechanism of stomatal closure in plants exposed to drought and cold stress’. Adv. Exp. Med. Biol. 1081, 215–232. doi: 10.1007/978-981-13-1244-1_12
Anfang, M., and Shani, E. (2021). ‘Transport mechanisms of plant hormones’. Curr. Opin. Plant Biol. 63:102055. doi: 10.1016/j.pbi.2021.102055
Ariel, F., Diet, A., Verdenaud, M., Gruber, V., Frugier, F., Chan, R., et al. (2010). ‘Environmental regulation of lateral root emergence in Medicago truncatula requires the HD-Zip I transcription factor HB1’. Plant Cell 22, 2171–2183. doi: 10.1105/tpc.110.074823
Aroca, R., Vernieri, P., and Ruiz-Lozano, J. M. (2008). ‘Mycorrhizal and non-mycorrhizal Lactuca sativa plants exhibit contrasting responses to exogenous ABA during drought stress and recovery’. J. Exp. Bot. 59, 2029–2041. doi: 10.1093/jxb/ern057
Barberon, M., Vermeer, J. E., De Bellis, D., Wang, P., Naseer, S., Andersen, T. G., et al. (2016). ‘Adaptation of root function by nutrient-induced plasticity of endodermal differentiation’. Cell 164, 447–459. doi: 10.1016/j.cell.2015.12.021
Bari, R., and Jones, J. D. G. (2009). ‘Role of plant hormones in plant defence responses’. Plant Mol. Biol. 69, 473–488. doi: 10.1007/s11103-008-9435-0
Baxter, A., Mittler, R., and Suzuki, N. (2014). ‘ROS as key players in plant stress signalling’. J. Exp. Bot. 65, 1229–1240. doi: 10.1093/jxb/ert375
Begum, N., Hu, Z., Cai, Q., and Lou, L. (2019). ‘Influence of PGPB Inoculation on HSP70 and HMA3 Gene Expression in Switchgrass under Cadmium Stress’. Plants (Basel) 8:504. doi: 10.3390/plants8110504
Bilias, F., Nikoli, T., Kalderis, D., and Gasparatos, D. (2021). ‘Towards a soil remediation strategy using biochar: effects on soil chemical properties and bioavailability of potentially toxic elements’. Toxics 9:184. doi: 10.3390/toxics9080184
Bittner, F., Oreb, M., and Mendel, R. R. (2001). ‘ABA3 is a molybdenum cofactor sulfurase required for activation of aldehyde oxidase and xanthine dehydrogenase in Arabidopsis thaliana’. J. Biol. Chem. 276, 40381–40384. doi: 10.1074/jbc.C100472200
Bloch, D., Puli, M. R., Mosquna, A., and Yalovsky, S. (2019). ‘Abiotic stress modulates root patterning via ABA-regulated microRNA expression in the endodermis initials’. Development 146:dev177097. doi: 10.1242/dev.177097
Brookbank, B. P., Patel, J., Gazzarrini, S., and Nambara, E. (2021). ‘Role of basal ABA in Plant growth and development’. Genes (Basel) 12:1936. doi: 10.3390/genes12121936
Cai, G., Ahmed, M. A., Abdalla, M., and Carminati, A. (2022). ‘Root hydraulic phenotypes impacting water uptake in drying soils’. Plant Cell Environ. 45, 650–663. doi: 10.1111/pce.14259
Campbell, L., Etchells, J. P., Cooper, M., Kumar, M., and Turner, S. R. (2018). ‘An essential role for abscisic acid in the regulation of xylem fibre differentiation’. Development 145:dev161992. doi: 10.1242/dev.161992
Carlsbecker, A., Lee, J. Y., Roberts, C. J., Dettmer, J., Lehesranta, S., Zhou, J., et al. (2010). ‘Cell signalling by microRNA165/6 directs gene dose-dependent root cell fate’. Nature 465, 316–321. doi: 10.1038/nature08977
Chen, C. W., Yang, Y. W., Lur, H. S., Tsai, Y. G., and Chang, M. C. (2006). ‘A novel function of abscisic acid in the regulation of rice (Oryza sativa L.) root growth and development’. Plant Cell Physiol. 47, 1–13. doi: 10.1093/pcp/pci216
Chen, H., Lai, Z., Shi, J., Xiao, Y., Chen, Z., and Xu, X. (2010). ‘Roles of Arabidopsis WRKY18, WRKY40 and WRKY60 transcription factors in plant responses to abscisic acid and abiotic stress’. BMC Plant Biol. 10:281. doi: 10.1186/1471-2229-10-281
Chen, K., Chen, P., Qiu, X., Chen, J., Gao, G., Wang, X., et al. (2021). ‘Regulating role of abscisic acid on cadmium enrichment in ramie (Boehmeria nivea L.)’. Sci. Rep. 11:22045. doi: 10.1038/s41598-021-00322-6
Chen, K., Li, G. J., Bressan, R. A., Song, C. P., Zhu, J. K., and Zhao, Y. (2020). ‘Abscisic acid dynamics, signaling, and functions in plants’. J. Integr. Plant Biol. 62, 25–54. doi: 10.1111/jipb.12899
Chen, Z., Liu, Q., Chen, S., Zhang, S., Wang, M., Mujtaba Munir, M. A., et al. (2022). ‘Roles of exogenous plant growth regulators on phytoextraction of Cd/Pb/Zn by Sedum alfredii Hance in contaminated soils’. Environ. Pollut. 293:118510. doi: 10.1016/j.envpol.2021.118510
Choudhury, F. K., Rivero, R. M., Blumwald, E., and Mittler, R. (2017). ‘Reactive oxygen species, abiotic stress and stress combination’. Plant J. Cell Mol. Biol. 90, 856–867. doi: 10.1111/tpj.13299
Cutler, S. R., Rodriguez, P. L., Finkelstein, R. R., and Abrams, S. R. (2010). ‘Abscisic acid: emergence of a core signaling network’. Annu. Rev. Plant Biol. 61, 651–679. doi: 10.1146/annurev-arplant-042809-112122
Dawuda, M. M., Liao, W., Hu, L., Yu, J., and Tang, Z. (2020). ‘Foliar application of abscisic acid mitigates cadmium stress and increases food safety of cadmium-sensitive lettuce (Lactuca sativa L.) genotype’. PeerJ. 8:e9270. doi: 10.7717/peerj.9270
Deng, X., Yang, Y., Zeng, H., Chen, Y., and Zeng, Q. (2020). ‘Variations in iron plaque, root morphology and metal bioavailability response to seedling establishment methods and their impacts on Cd and Pb accumulation and translocation in rice (Oryza sativa L.)’. J. Hazard. Mater. 384:121343. doi: 10.1016/j.jhazmat.2019.121343
Du, S., Lu, Q., Liu, L., Wang, Y., and Li, J. (2022). ‘Rhodococcus qingshengii facilitates the phytoextraction of Zn, Cd, Ni, and Pb from soils by Sedum alfredii Hance’. J. Hazard. Mater. 424(Pt C), 127638. doi: 10.1016/j.jhazmat.2021.127638
Eulgem, T., and Somssich, I. E. (2007). ‘Networks of WRKY transcription factors in defense signaling’. Curr. Opin. Plant Biol. 10, 366–371. doi: 10.1016/j.pbi.2007.04.020
Fan, S. K., Fang, X. Z., Guan, M. Y., Ye, Y. Q., Lin, X. Y., Du, S. T., et al. (2014). ‘Exogenous abscisic acid application decreases cadmium accumulation in Arabidopsis plants, which is associated with the inhibition of IRT1-mediated cadmium uptake’. Front. Plant Sci. 5:721. doi: 10.3389/fpls.2014.00721
Ge, L., Cang, L., Yang, J., and Zhou, D. (2016). ‘Effects of root morphology and leaf transpiration on Cd uptake and translocation in rice under different growth temperature’. Environ. Sci. Pollut. Res. Int. 23, 24205–24214. doi: 10.1007/s11356-016-7696-8
Gonzalez, A. A., Agbévénou, K., Herrbach, V., Gough, C., and Bensmihen, S. (2015). ‘Abscisic acid promotes pre-emergence stages of lateral root development in Medicago truncatula’. Plant Signal Behav. 10:e977741. doi: 10.4161/15592324.2014.977741
Guo, J., Guo, Y., Yang, J., Yang, J., Zheng, G., Chen, T., et al. (2020). ‘Effects and interactions of cadmium and zinc on root morphology and metal translocation in two populations of Hylotelephium spectabile (Boreau) H. Ohba, a potential Cd-accumulating species’. Environ. Sci. Pollut. Res. Int. 27, 21364–21375. doi: 10.1007/s11356-020-08660-0
Guo, J., Qin, S., Rengel, Z., Gao, W., Nie, Z., Liu, H., et al. (2019). ‘Cadmium stress increases antioxidant enzyme activities and decreases endogenous hormone concentrations more in Cd-tolerant than Cd-sensitive wheat varieties’. Ecotoxicol. Environ. Saf. 172, 380–387. doi: 10.1016/j.ecoenv.2019.01.069
Hamid, Y., Tang, L., Hussain, B., Usman, M., Lin, Q., Rashid, M. S., et al. (2020). ‘Organic soil additives for the remediation of cadmium contaminated soils and their impact on the soil-plant system: a review’. Sci. Total Environ. 707, 136121. doi: 10.1016/j.scitotenv.2019.136121
Han, Y., Shaojie, W., Nan, Z., Shurong, D., Chenjing, Z., Nianfei, L., et al. (2016). ‘Exogenous abscisic acid alleviates cadmium toxicity by restricting cd2+ influx in populus euphratica cells’. J. Plant Growth Regul. 35, 827–837. doi: 10.1007/s00344-016-9585-2
Harris, J. M. (2015). ‘Abscisic acid: hidden architect of root system structure’. Plants (Basel) 4, 548–572. doi: 10.3390/plants4030548
Hauser, F., Li, Z., Waadt, R., and Schroeder, J. I. (2017). ‘SnapShot: abscisic acid signaling’. Cell 171:1708. doi: 10.1016/j.cell.2017.11.045
He, J., Zhou, J., Wan, H., Zhuang, X., Li, H., Qin, S., et al. (2020). ‘Rootstock-Scion interaction affects cadmium accumulation and tolerance of malus’. Front. Plant Sci. 11:1264. doi: 10.3389/fpls.2020.01264
Hemberg, T. (1949). ‘Growth-Inhibiting substances in terminal buds of fraxinus’. Physiol. Plant. 2, 37–44. doi: 10.1111/j.1399-3054.1949.tb07646.x
Hong, C., Dan, C., Zhang, G., Zhu, D., Chen, Y., and Tan, M. (2016). ‘The role of ZmWRKY4 in regulating maize antioxidant defense under cadmium stress’. Biochem. Biophys. Res. Commun. 482:1504. doi: 10.1016/j.bbrc.2016.12.064
Hsu, P. K., Dubeaux, G., Takahashi, Y., and Schroeder, J. I. (2021). ‘Signaling mechanisms in abscisic acid-mediated stomatal closure’. Plant J. 105, 307–321. doi: 10.1111/tpj.15067
Hsu, Y. T., and Kao, C. H. (2008). ‘Distinct roles of abscisic acid in rice seedlings during cadmium stress at high temperature’. Bot. Stud. 49, 335–342.
Hsu, Y. T., and Kao, C. H. (2003). ‘Role of abscisic acid in cadmium tolerance of rice (Oryza sativa L.) seedlings’. Plant Cell Environ. 26, 867–874. doi: 10.1046/j.1365-3040.2003.01018.x
Hu, B., Deng, F., Chen, G., Chen, X., Gao, W., Long, L., et al. (2020). ‘Evolution of abscisic acid signaling for stress responses to toxic metals and metalloids’. Front. Plant Sci. 11:909. doi: 10.3389/fpls.2020.00909
Hu, T., Zhu, S., Tan, L., Qi, W., He, S., and Wang, G. (2016). ‘Overexpression of OsLEA4 enhances drought, high salt and heavy metal stress tolerance in transgenic rice (Oryza sativa L.)’. Environ. Exp. Bot. 123, 68–77. doi: 10.1016/j.envexpbot.2015.10.002
Huang, B., Dai, H., Zhou, W., Peng, L., Li, M., Wan, R., et al. (2019). ‘Characteristics of Cd accumulation and distribution in two sweet potato cultivars’. Int. J. Phytoremediation 21, 391–398. doi: 10.1080/15226514.2018.1524846
Huang, B., Xin, J., Dai, H., Liu, A., Zhou, W., Yi, Y., et al. (2015). ‘Root morphological responses of three hot pepper cultivars to Cd exposure and their correlations with Cd accumulation’. Environ. Sci. Pollut. Res. Int. 22, 1151–1159. doi: 10.1007/s11356-014-3405-7
Huang, Y., He, C., Shen, C., Guo, J., Mubeen, S., Yuan, J., et al. (2017). ‘Toxicity of cadmium and its health risks from leafy vegetable consumption’. Food Funct. 8, 1373–1401. doi: 10.1039/C6FO01580H
Hussain, Q., Asim, M., Zhang, R., Khan, R., Farooq, S., and Wu, J. (2021). ‘Transcription Factors Interact with ABA through gene expression and signaling pathways to mitigate drought and salinity stress’. Biomolecules 11:1159. doi: 10.3390/biom11081159
Jan, R., Khan, M. A., Asaf, S., Lee, I. J., and Kim, K. M. (2019). ‘Metal resistant endophytic bacteria reduces cadmium, nickel toxicity, and enhances expression of metal stress related genes with improved growth of Oryza Sativa, via regulating its antioxidant machinery and endogenous hormones’. Plants (Basel) 8:363. doi: 10.3390/plants8100363
Jiao, Y., Sun, L., Song, Y., Wang, L., Liu, L., Zhang, L., et al. (2013). ‘AtrbohD and AtrbohF positively regulate abscisic acid-inhibited primary root growth by affecting Ca2+ signalling and auxin response of roots in Arabidopsis’. J. Exp. Bot. 64, 4183–4192. doi: 10.1093/jxb/ert228
Kang, J., Hwang, J. U., Lee, M., Kim, Y. Y., Assmann, S. M., Martinoia, E., et al. (2010). ‘PDR-type ABC transporter mediates cellular uptake of the phytohormone abscisic acid’. Proc. Natl. Acad. Sci. U.S.A. 107, 2355–2360. doi: 10.1073/pnas.0909222107
Kang, J., Yim, S., Choi, H., Kim, A., Lee, K. P., Lopez-Molina, L., et al. (2015). ‘Abscisic acid transporters cooperate to control seed germination’. Nat. Commun. 6:8113. doi: 10.1038/ncomms9113
Kanno, Y., Hanada, A., Chiba, Y., Ichikawa, T., Nakazawa, M., Matsui, M., et al. (2012). ‘Identification of an abscisic acid transporter by functional screening using the receptor complex as a sensor’. Proc. Natl. Acad. Sci. U.S.A. 109, 9653–9658. doi: 10.1073/pnas.1203567109
Khanna, K., Kohli, S. K., Ohri, P., Bhardwaj, R., and Ahmad, P. (2022). ‘Agroecotoxicological aspect of Cd in soil-plant system: uptake, translocation and amelioration strategies. Environ. Sci. Pollut. Res. Int. 29, 30908–30934. doi: 10.1007/s11356-021-18232-5
Kubo, K., Watanabe, Y., Matsunaka, H., Seki, M., Fujita, M., Kawada, N., et al. (2011). ‘Differences in cadmium accumulation and root morphology in seedlings of japanese wheat varieties with distinctive grain cadmium concentration’. Plant Prod. Sci. 14, 148–155. doi: 10.1626/pps.14.148
Kuromori, T., Fujita, M., Urano, K., Tanabata, T., Sugimoto, E., and Shinozaki, K. (2016). ‘Overexpression of AtABCG25 enhances the abscisic acid signal in guard cells and improves plant water use efficiency’. Plant Sci. 251, 75–81. doi: 10.1016/j.plantsci.2016.02.019
Kuromori, T., Miyaji, T., Yabuuchi, H., Shimizu, H., Sugimoto, E., Kamiya, A., et al. (2010). ‘ABC transporter AtABCG25 is involved in abscisic acid transport and responses’. Proc. Natl. Acad. Sci. U.S.A. 107, 2361–2366. doi: 10.1073/pnas.0912516107
Kuromori, T., Sugimoto, E., and Shinozaki, K. (2014). ‘Intertissue signal transfer of abscisic acid from vascular cells to guard cells’. Plant Physiol. 164, 1587–1592. doi: 10.1104/pp.114.235556
Leng, Y., Li, Y., Ma, Y. H., He, L. F., and Li, S. W. (2021). ‘Abscisic acid modulates differential physiological and biochemical responses of roots, stems, and leaves in mung bean seedlings to cadmium stress’. Environ. Sci. Pollut. Res. Int. 28, 6030–6043. doi: 10.1007/s11356-020-10843-8
Li, T., Yang, X., Lu, L., Islam, E., and He, Z. (2009). ‘Effects of zinc and cadmium interactions on root morphology and metal translocation in a hyperaccumulating species under hydroponic conditions’. J. Hazard. Mater. 169, 734–741. doi: 10.1016/j.jhazmat.2009.04.004
Li, X., Chen, L., Forde, B. G., and Davies, W. J. (2017). ‘The biphasic root growth response to abscisic acid in Arabidopsis involves interaction with ethylene and auxin signalling pathways’. Front. Plant Sci. 8:1493. doi: 10.3389/fpls.2017.01493
Lim, C. W., Baek, W., Jung, J., Kim, J. H., and Lee, S. C. (2015). ‘Function of ABA in stomatal defense against biotic and drought stresses’. Int. J. Mol. Sci. 16, 15251–15270. doi: 10.3390/ijms160715251
Liu, L., Ma, Q., Lin, L., Tang, Y., Wang, J., Lv, X., et al. (2017). ‘Effects of exogenous abscisic acid on cadmium accumulation in two ecotypes of hyperaccumulator Bidens pilosa. Environ. Prog. Sustain. Energy 36, 1643–1649. doi: 10.1002/ep.12619
Liu, H., Zhao, H., Wu, L., Liu, A., Zhao, F. J., and Xu, W. (2017). ‘Heavy metal ATPase 3 (HMA3) confers cadmium hypertolerance on the cadmium/zinc hyperaccumulator Sedum plumbizincicola’. New Phytol. 215, 687–698. doi: 10.1111/nph.14622
Liu, S., Kracher, B., Ziegler, J., Birkenbihl, R. P., and Somssich, I. E. (2015). ‘Negative regulation of ABA signaling by WRKY33 is critical for Arabidopsis immunity towards Botrytis cinerea 2100’. Elife 4:e07295. doi: 10.7554/eLife.07295.033
Liu, S., Li, L., Deng, Y., Bai, Y., Sun, C., Huang, S., et al. (2022). ‘BrpNAC895 and BrpABI449 coregulate the transcription of the afflux-type Cd transporter BrpHMA2 in Brassica parachinensis’. Hortic Res. 19:004. doi: 10.1093/hr/uhac044
Liu, X., Peng, K., Wang, A., Lian, C., and Shen, Z. (2010). ‘Cadmium accumulation and distribution in populations of Phytolacca americana L. and the role of transpiration’. Chemosphere 78, 1136–1141. doi: 10.1016/j.chemosphere.2009.12.030
Lu, Q., Weng, Y., You, Y., Xu, Q., Li, H., Li, Y., et al. (2020a). ‘Inoculation with abscisic acid (ABA)-catabolizing bacteria can improve phytoextraction of heavy metal in contaminated soil’. Environ. Pollut. 257:113497. doi: 10.1016/j.envpol.2019.113497
Lu, Q., Chen, S., Li, Y., Zheng, F., He, B., and Gu, M. (2020b). ‘Exogenous abscisic acid (ABA) promotes cadmium (Cd) accumulation in Sedum alfredii Hance by regulating the expression of Cd stress response genes’. Environ. Sci. Pollut. Res. Int. 27, 8719–8731. doi: 10.1007/s11356-019-07512-w
Lux, A., Martinka, M., Vaculík, M., and White, P. J. (2011). ‘Root responses to cadmium in the rhizosphere: a review’. J. Exp. Bot. 62, 21–37. doi: 10.1093/jxb/erq281
Mahmoud, A., AbdElgawad, H., Hamed, B. A., Beemster, G. T. S., and El-Shafey, N. M. (2021). ‘Differences in cadmium accumulation, detoxification and antioxidant defenses between contrasting maize cultivars implicate a role of superoxide dismutase in cd tolerance. Antioxidants (Basel) 10:1812. doi: 10.3390/antiox10111812
McLoughlin, F., Galvan-Ampudia, C. S., Julkowska, M. M., Caarls, L., van der Does, D., Laurière, C., et al. (2012). ‘The Snf1-related protein kinases SnRK2.4 and SnRK2.10 are involved in maintenance of root system architecture during salt stress’. Plant J. 72, 436–449. doi: 10.1111/j.1365-313X.2012.05089.x
Mega, R., Abe, F., Kim, J.-S., Tsuboi, Y., Tanaka, K., Kobayashi, H., et al. (2019). ‘Tuning water-use efficiency and drought tolerance in wheat using abscisic acid receptors’. Nat. plants 5, 153–159. doi: 10.1038/s41477-019-0361-8
Mendoza-Cózatl, D. G., Jobe, T. O., Hauser, F., and Schroeder, J. I. (2011). ‘Long-distance transport, vacuolar sequestration, tolerance, and transcriptional responses induced by cadmium and arsenic’. Curr. Opin. Plant Biol. 14, 554–562. doi: 10.1016/j.pbi.2011.07.004
Miller, G., Suzuki, N., Ciftci-Yilmaz, S., and Mittler, R. (2010). ‘Reactive oxygen species homeostasis and signalling during drought and salinity stresses’. Plant Cell Environ. 33, 453–467. doi: 10.1111/j.1365-3040.2009.02041.x
Mittler, R. (2002). ‘Oxidative stress, antioxidants and stress tolerance’. Trends Plant Sci. 7, 405–410. doi: 10.1016/S1360-1385(02)02312-9
Miyashima, S., Koi, S., Hashimoto, T., and Nakajima, K. (2011). ‘Non-cell-autonomous microRNA165 acts in a dose-dependent manner to regulate multiple differentiation status in the Arabidopsis root’. Development 138, 2303–2313. doi: 10.1242/dev.060491
Pan, W., Lu, Q., Xu, Q. R., Zhang, R. R., Li, H. Y., Yang, Y. H., et al. (2019). ‘Abscisic acid-generating bacteria can reduce Cd concentration in pakchoi grown in Cd-contaminated soil’. Ecotoxicol. Environ. Saf. 177, 100–107. doi: 10.1016/j.ecoenv.2019.04.010
Pan, W., You, Y., Shentu, J. L., Weng, Y. N., Wang, S. T., Xu, Q. R., et al. (2020). ‘Abscisic acid (ABA)-importing transporter 1 (AIT1) contributes to the inhibition of Cd accumulation via exogenous ABA application in Arabidopsis’. J. Hazard. Mater. 391:122189. doi: 10.1016/j.jhazmat.2020.122189
Papoyan, A., and Kochian, L. V. (2004). ‘Identification of Thlaspi caerulescens genes that may be involved in heavy metal hyperaccumulation and tolerance characterization of a novel heavy metal transporting ATPase’. Plant Physiol. 136, 3814–3823. doi: 10.1104/pp.104.044503
Peuke, A. D. (2016). ‘ABA flow modelling in Ricinus communis exposed to salt stress and variable nutrition’. J. Exp. Bot. 67, 5301–5311. doi: 10.1093/jxb/erw291
Pompeu, G. B., Vilhena, M. B., Gratão, P. L., Carvalho, R. F., Rossi, M. L., Martinelli, A. P., et al. (2017). ‘Abscisic acid-deficient sit tomato mutant responses to cadmium-induced stress’. Protoplasma 254, 771–783. doi: 10.1007/s00709-016-0989-4
Pornsiriwong, W., Estavillo, G. M., Chan, K. X., Tee, E. E., Ganguly, D., Crisp, P. A., et al. (2017). ‘A chloroplast retrograde signal, 3’-phosphoadenosine 5’-phosphate, acts as a secondary messenger in abscisic acid signaling in stomatal closure and germination’. Elife 6:e23361. doi: 10.7554/eLife.23361.026
Qi, J., Wang, J., Gong, Z., and Zhou, J. M. (2017). ‘Apoplastic ROS signaling in plant immunity’. Curr. Opin. Plant Biol. 38, 92–100. doi: 10.1016/j.pbi.2017.04.022
Ramachandran, P., Augstein, F., Mazumdar, S., Nguyen, T. V., Minina, E. A., Melnyk, C. W., et al. (2021). ‘Abscisic acid signaling activates distinct VND transcription factors to promote xylem differentiation in Arabidopsis’. Curr. Biol. 31, 3153–3161. doi: 10.1016/j.cub.2021.04.057
Ramachandran, P., Wang, G., Augstein, F., de Vries, J., and Carlsbecker, A. (2018). ‘Continuous root xylem formation and vascular acclimation to water deficit involves endodermal ABA signalling via miR165’. Development 145:dev159202. doi: 10.1242/dev.159202
Raza, A., Habib, M., Kakavand, S. N., Zahid, Z., Zahra, N., Sharif, R., et al. (2020). ‘Phytoremediation of cadmium: physiological, biochemical, and molecular mechanisms. Biology (Basel) 9:177. doi: 10.3390/biology9070177
Redjala, T., Zelko, I., Sterckeman, T., Legué, V., and Lux, A. (2011). ‘Relationship between root structure and root cadmium uptake in maize’. Environ. Exp. Bot. 71, 241–248. doi: 10.1016/j.envexpbot.2010.12.010
Ricachenevsky, F. K., de Araújo, A. T. Jr., Fett, J. P., and Sperotto, R. A. (2018). ‘You shall not pass: root vacuoles as a symplastic checkpoint for metal translocation to shoots and possible application to grain nutritional quality’. Front. Plant Sci. 9:412. doi: 10.3389/fpls.2018.00412
Rizwan, M., Ali, S., Abbas, T., Zia-Ur-Rehman, M., Hannan, F., Keller, C., et al. (2016). ‘Cadmium minimization in wheat: A critical review’. Ecotoxicol. Environ. Saf. 130, 43–53. doi: 10.1016/j.ecoenv.2016.04.001
Rushton, D. L., Tripathi, P., Rabara, R. C., Lin, J., Ringler, P., Boken, A. K., et al. (2012). ‘WRKY transcription factors: key components in abscisic acid signalling’. Plant Biotechnol. J. 10, 2–11. doi: 10.1111/j.1467-7652.2011.00634.x
Ryu, J., Hwang, B. G., Kim, Y. X., and Lee, S. J. (2016). ‘Direct observation of local xylem embolisms induced by soil drying in intact Zea mays leaves’. J. Exp. Bot. 67, 2617–2626. doi: 10.1093/jxb/erw087
Schenk, H. J., Jansen, S., and Hölttä, T. (2021). ‘Positive pressure in xylem and its role in hydraulic function’. New Phytol. 230, 27–45. doi: 10.1111/nph.17085
Schwartz, S. H., Tan, B. C., Gage, D. A., Zeevaart, J. A., and McCarty, D. R. (1997). ‘Specific oxidative cleavage of carotenoids by VP14 of maize’. Science 276, 1872–1874. doi: 10.1126/science.276.5320.1872
Shahid, M., Pourrut, B., Dumat, C., Nadeem, M., Aslam, M., and Pinelli, E. (2014). ‘Heavy-metal-induced reactive oxygen species: phytotoxicity and physicochemical changes in plants’. Rev. Environ. Contaminat. Toxicol. 232, 1–44. doi: 10.1007/978-3-319-06746-9_1
Shen, C., Huang, Y. Y., Xin, J. L., He, C. T., and Yang, Z. Y. (2022). ‘A novel microRNA IamiR-4-3p from water spinach (Ipomoea aquatica Forsk.) increased Cd uptake and translocation in Arabidopsis thaliana’. Environ. Sci. Pollut. Res. Int. 29, 41375–41385. doi: 10.1007/s11356-022-18875-y
Shen, G., Niu, J., and Deng, Z. (2017). ‘Abscisic acid treatment alleviates cadmium toxicity in purple flowering stalk (Brassica campestris L. ssp. chinensis var. purpurea Hort.) seedlings’. Plant Physiol. Biochem. 118, 471–478. doi: 10.1016/j.plaphy.2017.07.018
Siemianowski, O., Barabasz, A., Kendziorek, M., Ruszczynska, A., Bulska, E., Williams, L. E., et al. (2014). ‘HMA4 expression in tobacco reduces Cd accumulation due to the induction of the apoplastic barrier’. J. Exp. Bot. 65, 1125–1139. doi: 10.1093/jxb/ert471
Song, Y., Jin, L., and Wang, X. (2017). ‘Cadmium absorption and transportation pathways in plants’. Int. J. Phytoremediation 19, 133–141. doi: 10.1080/15226514.2016.1207598
Sun, L. R., Wang, Y. B., He, S. B., and Hao, F. S. (2018). ‘Mechanisms for abscisic acid inhibition of primary root growth’. Plant Signal Behav. 13:e1500069. doi: 10.1080/15592324.2018.1500069
Sun, S., Li, X., Gao, S., Nie, N., Zhang, H., Yang, Y., et al. (2022). ‘A Novel WRKY Transcription Factor from, ItfWRKY70, confers drought tolerance in sweet potato’. Int. J. Mol. Sci. 23:686. doi: 10.3390/ijms23020686
Szopiński, M., Sitko, K., Rusinowski, S., Zieleźnik-Rusinowska, P., Corso, M., Rostański, A., et al. (2020). ‘Different strategies of Cd tolerance and accumulation in Arabidopsis halleri and Arabidopsis arenosa’. Plant Cell Environ. 43, 3002–3019. doi: 10.1111/pce.13883
Takahashi, R., Bashir, K., Ishimaru, Y., Nishizawa, N. K., and Nakanishi, H. (2012). ‘The role of heavy-metal ATPases, HMAs, in zinc and cadmium transport in rice’. Plant Signal Behav. 7, 1605–1607. doi: 10.4161/psb.22454
Tang, Y., Wang, L., Xie, Y., Yu, X., Li, H., Lin, L., et al. (2020). ‘Effects of exogenous abscisic acid on the growth and cadmium accumulation of lettuce under cadmium-stress conditions’. Int. J. Environ. Analyt. Chem. 100, 720–731. doi: 10.1080/03067319.2019.1639686
Tao, Q., Jupa, R., Dong, Q., Yang, X., Liu, Y., Li, B., et al. (2021). ‘Abscisic acid-mediated modifications in water transport continuum are involved in cadmium hyperaccumulation in Sedum alfredii’. Chemosphere 268:129339. doi: 10.1016/j.chemosphere.2020.129339
Tao, Q., Jupa, R., Liu, Y., Luo, J., Li, J., Kováè, J., et al. (2019). ‘Abscisic acid-mediated modifications of radial apoplastic transport pathway play a key role in cadmium uptake in hyperaccumulator Sedum alfredii’. Plant Cell Environ. 42, 1425–1440. doi: 10.1111/pce.13506
Tao, Q., Jupa, R., Luo, J., Lux, A., Kováè, J., Wen, Y., et al. (2017). ‘The apoplasmic pathway via the root apex and lateral roots contributes to Cd hyperaccumulation in the hyperaccumulator Sedum alfredii’”. J. Exp. Bot. 68, 739–751. doi: 10.1093/jxb/erw453
Tsukagoshi, H. (2016). ‘Control of root growth and development by reactive oxygen species’. Curr. Opin. Plant Biol. 29, 57–63. doi: 10.1016/j.pbi.2015.10.012
Umezawa, T., Sugiyama, N., Mizoguchi, M., Hayashi, S., Myouga, F., Yamaguchi-Shinozaki, K., et al. (2009). ‘Type 2C protein phosphatases directly regulate abscisic acid-activated protein kinases in Arabidopsis’. Proc. Natl. Acad. Sci. U.S.A. 106, 17588–17593. doi: 10.1073/pnas.0907095106
Uraguchi, S., Mori, S., Kuramata, M., Kawasaki, A., Arao, T., and Ishikawa, S. (2009). ‘Root-to-shoot Cd translocation via the xylem is the major process determining shoot and grain cadmium accumulation in rice’. J. Exp. Bot. 60, 2677–2688. doi: 10.1093/jxb/erp119
Vaculík, M., Konlechner, C., Langer, I., Adlassnig, W., Puschenreiter, M., Lux, A., et al. (2012). ‘Root anatomy and element distribution vary between two Salix caprea isolates with different Cd accumulation capacities’. Environ. Pollut. 163, 117–126. doi: 10.1016/j.envpol.2011.12.031
Vaculík, M., Lux, A., Luxová, M., Tanimoto, E., and Lichtscheidl, I. (2009). ‘Silicon mitigates cadmium inhibitory effects in young maize plants’. Environ. Exp. Bot. 67, 52–58. doi: 10.1016/j.envexpbot.2009.06.012
Vareda, J. P., Valente, A. J. M., and Durães, L. (2019). ‘Assessment of heavy metal pollution from anthropogenic activities and remediation strategies: a review’. J. Environ. Manage. 246, 101–118. doi: 10.1016/j.jenvman.2019.05.126
Vert, G., Grotz, N., Dédaldéchamp, F., Gaymard, F., Guerinot, M. L., Briat, J. F., et al. (2002). ‘IRT1, an Arabidopsis transporter essential for iron uptake from the soil and for plant growth’. Plant Cell 14, 1223–1233. doi: 10.1105/tpc.001388
Vishwakarma, K., Upadhyay, N., Kumar, N., Yadav, G., Singh, J., Mishra, R. K., et al. (2017). ‘Abscisic acid signaling and abiotic stress tolerance in plants: a review on current knowledge and future prospects’. Front Plant Sci. 8:161. doi: 10.3389/fpls.2017.00161
Wang, J., Lin, L., Luo, L., Liao, M., Lv, X., Wang, Z., et al. (2016). ‘The effects of abscisic acid (ABA) addition on cadmium accumulation of two ecotypes of Solanum photeinocarpum’. Environ. Monit. Assess. 188:182. doi: 10.1007/s10661-016-5194-6
Wang, L., Zhang, Q., Liao, X., Li, X., Zheng, S., and Zhao, F. (2021). ‘Phytoexclusion of heavy metals using low heavy metal accumulating cultivars: a green technology’. J. Hazard. Mater. 413:125427. doi: 10.1016/j.jhazmat.2021.125427
Wang, N., Cui, Y., Liu, Y., Fan, H., Du, J., Huang, Z., et al. (2013). ‘Requirement and functional redundancy of Ib subgroup bHLH proteins for iron deficiency responses and uptake in Arabidopsis thaliana’. Mol. Plant 6, 503–513. doi: 10.1093/mp/sss089
Wang, S., Wu, W., Liu, F., Liao, R., and Hu, Y. (2017). ‘Accumulation of heavy metals in soil-crop systems: a review for wheat and corn’. Environ. Sci. Pollut. Res. Int. 24, 15209–15225. doi: 10.1007/s11356-017-8909-5
Wang, T., Hua, Y., Chen, M., Zhang, J., Guan, C., and Zhang, Z. (2018). ‘Mechanism enhancing Arabidopsis resistance to cadmium: the role of nrt1.5 and proton pump’. Front. Plant Sci. 9:1892. doi: 10.3389/fpls.2018.01892
Wang, T., Li, Y., Fu, Y., Xie, H., Song, S., Qiu, M., et al. (2019). ‘Mutation at different sites of metal transporter gene osnramp5 affects cd accumulation and related agronomic traits in rice (Oryza sativa L.)’. Front. Plant Sci. 10:1081. doi: 10.3389/fpls.2019.01081
Waszczak, C., Carmody, M., and Kangasjärvi, J. (2018). ‘Reactive oxygen species in plant signaling’. Ann. Rev. Plant Biol. 69, 209–236. doi: 10.1146/annurev-arplant-042817-040322
Wu, H., Chen, C., Du, J., Liu, H., Cui, Y., Zhang, Y., et al. (2012). ‘Co-overexpression FIT with AtbHLH38 or AtbHLH39 in Arabidopsis-enhanced cadmium tolerance via increased cadmium sequestration in roots and improved iron homeostasis of shoots’. Plant Physiol. 158, 790–800. doi: 10.1104/pp.111.190983
Wu, Y., Ma, L., Liu, Q., Vestergård, M., Topalovic, O., Wang, Q., et al. (2020). ‘The plant-growth promoting bacteria promote cadmium uptake by inducing a hormonal crosstalk and lateral root formation in a hyperaccumulator plant Sedum alfredii’. J. Hazard. Mater. 395:122661. doi: 10.1016/j.jhazmat.2020.122661
Xia, S., Deng, R., Zhang, Z., Liu, C., and Shi, G. (2016). ‘Variations in the accumulation and translocation of cadmium among pak choi cultivars as related to root morphology’. Environ. Sci. Pollut. Res. Int. 23, 9832–9842. doi: 10.1007/s11356-016-6210-7
Xin, J., Huang, B., Dai, H., and Mu, Y. (2017). ‘Characterization of root morphology and root-derived low molecular weight organic acids in two sweet potato cultivars exposed to cadmium’. Arch. Agronomy Soil Sci. 63, 723–734. doi: 10.1080/03650340.2016.1234041
Xu, Q., Pan, W., Zhang, R., Lu, Q., Xue, W., Wu, C., et al. (2018). ‘Inoculation with Bacillus subtilis and Azospirillum brasilense produces abscisic acid that reduces irt1-mediated cadmium uptake of roots’. J. Agric. Food Chem. 66, 5229–5236. doi: 10.1021/acs.jafc.8b00598
Yan, J., Zhao, C., Zhou, J., Yang, Y., Wang, P., Zhu, X., et al. (2016). ‘The miR165/166 Mediated regulatory module plays critical roles in aba homeostasis and response in Arabidopsis thaliana’. PLoS Genet. 12:e1006416. doi: 10.1371/journal.pgen.1006416
Yang, G., Wang, C., Wang, Y., Guo, Y., Zhao, Y., Yang, C., et al. (2016). ‘Overexpression of ThVHAc1 and its potential upstream regulator, ThWRKY7, improved plant tolerance of Cadmium stress’. Sci. Rep. 6:18752. doi: 10.1038/srep18752
Yang, J., Chen, X., Lu, W., Chen, R., Liu, M., Yao, H., et al. (2020). ‘Reducing Cd accumulation in rice grain with foliar application of glycerol and its mechanisms of Cd transport inhibition’. Chemosphere 258:127135. doi: 10.1016/j.chemosphere.2020.127135
Yang, Y., Xiong, J., Tao, L., Cao, Z., Tang, W., Zhang, J., et al. (2020). ‘Regulatory mechanisms of nitrogen (N) on cadmium (Cd) uptake and accumulation in plants: a review’. Sci. Total Environ. 708:135186. doi: 10.1016/j.scitotenv.2019.135186
Ye, Y., Dong, W., Luo, Y., Fan, T., Xiong, X., Sun, L., et al. (2020). ‘Cultivar diversity and organ differences of cadmium accumulation in potato (Solanum tuberosum L.) allow the potential for Cd-safe staple food production on contaminated soils’. Sci. Total Environ. 711, 134534. doi: 10.1016/j.scitotenv.2019.134534
Yu, R., Xia, S., Liu, C., Zhang, Z., and Shi, G. (2017). ‘Variations in root morphology among 18 herbaceous species and their relationship with cadmium accumulation’. Environ. Sci. Pollut. Res. Int. 24, 4731–4740. doi: 10.1007/s11356-016-8210-z
Zhang, H., Zhu, H., Pan, Y., Yu, Y., Luan, S., and Li, L. (2014). ‘A DTX/MATE-type transporter facilitates abscisic acid efflux and modulates ABA sensitivity and drought tolerance in Arabidopsis’. Mol. Plant. 7, 1522–1532. doi: 10.1093/mp/ssu063
Zhang, J., Zhang, H., Srivastava, A. K., Pan, Y., Bai, J., Fang, J., et al. (2018). ‘Knockdown of Rice MicroRNA166 confers drought resistance by causing leaf rolling and altering stem xylem development’. Plant Physiol. 176, 2082–2094. doi: 10.1104/pp.17.01432
Zhang, P., Wang, R., Ju, Q., Li, W., Tran, L. P., and Xu, J. (2019). ‘The R2R3-MYB transcription factor MYB49 regulates cadmium accumulation’. Plant Physiol. 180, 529–542. doi: 10.1104/pp.18.01380
Zhang, W., Long, J., Zhang, X., Shen, W., and Wei, Z. (2020). ‘Pollution and Ecological Risk evaluation of heavy metals in the soil and sediment around the htm tailings pond, northeastern China’. Int. J. Environ. Res. Public Health 17:7072. doi: 10.3390/ijerph17197072
Zhang, W., Wang, Z., Song, J., Yue, S., and Yang, H. (2019). ‘Cd2+ uptake inhibited by MhNCED3 from Malus hupehensis alleviates Cd-induced cell death’. Environ. Exp. Bot. 166:103802. doi: 10.1016/j.envexpbot.2019.103802
Zhang, Z., Liu, C., Wang, X., and Shi, G. (2013). ‘Cadmium-induced alterations in morpho-physiology of two peanut cultivars differing in cadmium accumulation’. Acta Physiol. Plant. 35, 2105–2112. doi: 10.1007/s11738-013-1247-4
Zhao, F.-J., and Wang, P. (2020). ‘Arsenic and cadmium accumulation in rice and mitigation strategies’. Plant Soil 446, 1–21. doi: 10.1007/s11104-019-04374-6
Zheng, L., Liu, G., Meng, X., Liu, Y., Ji, X., Li, Y., et al. (2013). ‘A WRKY gene from Tamarix hispida, ThWRKY4, mediates abiotic stress responses by modulating reactive oxygen species and expression of stress-responsive genes’. Plant Mol. Biol. 82, 303–320. doi: 10.1007/s11103-013-0063-y
Keywords: ABA synthesis, Cd uptake, Cd translocation, root development, ROS
Citation: Shen C, Yang Y-M, Sun Y-F, Zhang M, Chen X-J and Huang Y-Y (2022) The regulatory role of abscisic acid on cadmium uptake, accumulation and translocation in plants. Front. Plant Sci. 13:953717. doi: 10.3389/fpls.2022.953717
Received: 26 May 2022; Accepted: 19 July 2022;
Published: 13 September 2022.
Edited by:
Luis E. Hernandez, Autonomous University of Madrid, SpainReviewed by:
Jie Zhang, Foshan University, ChinaAryadeep Roychoudhury, St. Xavier’s College (Autonomous), Kolkata, India
Copyright © 2022 Shen, Yang, Sun, Zhang, Chen and Huang. This is an open-access article distributed under the terms of the Creative Commons Attribution License (CC BY). The use, distribution or reproduction in other forums is permitted, provided the original author(s) and the copyright owner(s) are credited and that the original publication in this journal is cited, in accordance with accepted academic practice. No use, distribution or reproduction is permitted which does not comply with these terms.
*Correspondence: Ying-Ying Huang, huangyy@hnit.edu.cn