- 1State Key Laboratory for Conservation and Utilization of Subtropical Agro-Bioresources, College of Life Sciences, South China Agricultural University, Guangzhou, China
- 2Shaanxi Key Laboratory of Chinese Jujube, Yan’an University, Yan’an, China
- 3College of Agriculture, Fujian Agriculture and Forestry University, Fuzhou, China
Cadmium (Cd) is a harmful heavy metal that is risky for plant growth and human health. The zinc-finger transcription factor ZAT10 is highly conserved with ZAT6 and ZAT12, which are involved in Cd tolerance in plants. However, the definite function of ZAT10 in Cd tolerance remains uncertain. Here, we demonstrated that ZAT10 negatively regulated Cd uptake and enhanced Cd detoxification in Arabidopsis. The expression of ZAT10 in plants is induced by Cd treatment. The zat10 mutant plants exhibited a greater sensitivity to Cd stress and accumulated more Cd in both shoot and root. Further investigations revealed that ZAT10 repressed the transcriptional activity of IRT1, which encodes a key metal transporter involved in Cd uptake. Meanwhile, ZAT10 positively regulated four heavy metal detoxification-related genes: NAS1, NAS2, IRT2, and MTP3. We further found that ZAT10 interacts with FIT, but their regulatory relationship is still unclear. In addition, ZAT10 directly bound to its own promoter and repressed its transcription as a negative feedback regulation. Collectively, our findings provided new insights into the dual functions of ZAT10 on Cd uptake and detoxification in plants and pointed to ZAT10 as a potential gene resource for Cd tolerance improvement in plants.
Introduction
Cadmium (Cd) is toxic to plants and poses a significant risk to food production and food safety. The accumulation of cadmium in agricultural soils has increased as a result of human activities and modern industrial practices (Antoniadis et al., 2017; Geng et al., 2019; Wang et al., 2019; Palansooriya et al., 2020). Many studies have shown that Cd inhibits chlorophyll synthesis and root development, leading to new leaf chlorosis, root elongation inhibition, and even plant death (Verbruggen et al., 2009; Bae et al., 2016; Dang et al., 2019). Plants have evolved several potential mechanisms to cope with Cd stress, such as avoiding cadmium toxicity by limiting Cd uptake and enhancing Cd tolerance through accumulation, storage, and immobilization of Cd elements in certain plant tissues (El Rasafi et al., 2020).
However, no specific transporter or channel for the uptake of Cd into plant cells has been reported. The uptake of Cd is mostly attributed to cationic transporters or channels, such as the Iron Regulated Transporter 1 (IRT1), Zinc-regulated/Iron-regulated transporter-like family Proteins (ZIPs), Natural Resistance-Associated Macrophage Protein family (NRAMP), and metal pump ATPase (Gallego et al., 2012; Antoniadis et al., 2017; Zheng et al., 2018; Spielmann et al., 2020). In our previous study, we pointed out that Cd exposure caused Fe deficiency-induced chlorosis in emerging leaves of pepper plants (Dang et al., 2019). Several studies have shown that IRT1 is involved in the regulation of Cd uptake from soil (Lombi et al., 2002; Vert et al., 2002; Fan et al., 2014). These studies suggested that Cd competed with Fe in the process of root uptake of metal elements. This competition, in turn, exacerbates the Fe deficiency response in plants and results in the up-regulation of IRT1 transcript level, further leading to more Cd uptake. In addition, the FER-like iron deficiency-induced transcription factor (FIT), a basic helix loop helix (bHLH) transcription factor, is the pivotal regulator of Fe-deficiency responses and Fe homeostasis in Arabidopsis. It has been reported that FIT interacts with various proteins, such as bHLH038, bHLH039, bHLH100, bHLH101, bHLH18, bHLH19, bHLH20, bHLH25, and EIN3/EIL1, to regulate the expression of IRT1 and thus the uptake of Cd (Yuan et al., 2008; Lingam et al., 2011; Zhu et al., 2013; Wu and Ling, 2019).
On the other hand, plants up-regulate the expression of genes relevant to heavy metal sequestration and chelation to prevent heavy metal toxicity, such as the vacuolar membrane-localized metal transporters Heavy Metal Associated 3 (HMA3), Heavy Metal Associated 4 (HMA4), Metal Tolerance Protein 1 (MTP1), Metal Tolerance Protein 3 (MTP3), Iron Regulated Gene 2 (IREG2), and the intracellular vesicle membrane protein IRT2 (Arrivault et al., 2006; Morel et al., 2009; Vert et al., 2009; Wu et al., 2012; Yao et al., 2018). Previous studies have shown that these genes participate in cytoplasmic detoxification through transporting heavy metals into vacuoles and vesicles of cells. It was also found that Nicotianamine Synthase (NAS) confers Cd tolerance by increasing NA content (Kim et al., 2005; Klatte et al., 2009). The bHLH104 has been shown to participate in Cd tolerance through regulating heavy metal sequestration and detoxification-associated genes, such as MTP3, HMA3, IREG2, and NAS4 (Yao et al., 2018). Likewise, the zinc-finger transcription factor ZAT6 is induced by Cd stress and positively regulates the expression of GSH1 and PC biosynthesis-related genes, which consequently enhances Cd tolerance (Chen et al., 2016). In addition, besides affecting Cd uptake, FIT also regulates Cd tolerance by interacting with bHLH38 or bHLH39 to form FIT/bHLH38 and FIT/bHLH39 heterodimers. These modules increase the expression of MTP3, HMA3, IREG2, IRT2, NAS1, and NAS2, to enhance Cd sequestration in plant cells (Wu et al., 2012). However, while these studies have identified some of the major genes involved in Cd detoxification and tolerance in plants, a more comprehensive understanding of the transcriptional networks that regulate Cd tolerance is still required.
The zinc-finger transcription factor ZAT10 is highly conserved with ZAT6 and ZAT12, which are involved in the responses to Cd stress and abiotic stress in plants (Mittler et al., 2006; Opdenakker et al., 2012; Shi et al., 2014; Chen et al., 2016). However, the definite function of ZAT10 in Cd tolerance is still unknown. In the present study, we revealed the novel function of ZAT10 in response to Cd stress in plants. ZAT10 negatively regulated Cd uptake by repressing the expression of IRT1 and enhanced Cd detoxification by promoting the expression of Cd sequestration genes.
Materials and methods
Sequences alignment and phylogenetic analysis
The amino acid sequences used for sequence alignment and phylogenetic analysis were obtained from NCBI protein database. The amino acid sequence alignment was conducted using the DNAMAN software. The phylogenetic tree was performed by MEGA 10 software using the maximum likelihood method with default settings.
Plant material, growth conditions
The Arabidopsis thaliana ecotype Col-0 was used as the wild type in this study. The ZAT10 T-DNA insertion line (zat10, SALK_054092C) obtained from the Arabidopsis Biological Resource Center (ABRC1) was used (Alonso et al., 2003). ZAT10 primers and the T-DNA left-border primer (LBb1.3) were used for the identification of T-DNA lines (Supplementary Table 1). Surface-sterilized seeds were exposed to 4°C for 2 days before germinated on culture. For phenotype assays, WT and zat10 mutant seeds were sown on 1/2 Murashige and Skoog (MS) medium containing 0.8% agar (Sigma, no. A1296) with 0, 30, 60, or 90 μM CdSO4 for 9 days. For gene expression analysis under Cd stress, about 12 seedlings were cultured in 1 ml of the 1/2 MS liquid medium in the six-well tissue culture plate (Sangon Biotech, Shanghai, China) for 5 days. Then, seedlings were washed three times with 1 mL 1/2 MS medium and were transferred to 1/2 MS liquid medium containing the indicated concentration of CdSO4 for a specific period of time. Seedlings were harvested for RNA extraction using RNAprep Pure Plant Kit (Tiangen Biotech, Beijing, China). Arabidopsis seedlings were grown at 22 ± 2°C in the growth chamber under a 16 h light/8 h dark cycle with a light intensity of about 100 μmol photons m–2s–1. Nicotiana benthamiana was used for transient expression experiments in this study, and plants were grown in growth room at 28 ± 2°C under a 16 h light/8 h dark cycle.
Molecular cloning and plasmid construction
To generate complementary lines of zat10 mutant, a genomic DNA fragment of ZAT10 with its own promoter (∼2,000 bp upstream of the ATG) was amplified using primers listed in Supplementary Table 1 and cloned into SacI and SalI sites of pCAMBIA1300 vector without 35S promoter. Agrobacterium-mediated Arabidopsis transformation was performed by the floral dip method.
For molecular analysis, the full-length coding fragments of ZAT10, ZAT12, FIT, and the promoters of ZAT6, ZAT10, FIT, IRT1, ZIP1, ZIP3, ZIP4, ZIP5, ZIP9, IREG1, IRGE2, NAS1, NAS2, MTP3, and HMA3 were amplified by PCR using the specific primers listed in Supplementary Table 1. And then these fragments were cloned into the pCE-Zero vector using the ClonExpress Ultra One Step Cloning Kit (Vazyme, Nanjing, China) and sequenced by Sangon Biotech (Shanghai, China). Then the ZAT10 coding region was inserted into the plant expression vector (35S-GFP-NOS plasmid) to generate the 35S:ZAT10-GFP construct. Promoters of target genes were fused into the plant reporter vector (35S:REN-NOS-promoter:LUC-NOS plasmid) to generate the 35S:REN-promoter:LUC construct.
Histochemical staining
Hydrogen peroxide (H2O2) and superoxide radicals (O2–) were analyzed using 3, 3′-diaminobenzidine (DAB) and NitroBlue Tetrazolium (NBT), respectively, as described previously (Dang et al., 2013, 2019; Shi et al., 2013). In brief, the seedlings were immersed in DAB solution (pH 3.8, 1 mg/ml) or NBT solution (1 mg/ml) at 25°C for 12 h. The seedlings were then completely bleached by 70% ethanol (v/v) and photographed by a Stereo Microscope (Leica, Wetzlar, Germany).
Measurements of cd and hydrogen peroxide content
Measurements of Cd and H2O2 content were performed as described previously (Dang et al., 2019; Ding et al., 2021, 2022). In brief, 7-day-old Arabidopsis seedlings were transferred to 1/2 MS medium containing 30 μM CdSO4 for 3 and 5 days, then samples were harvested and used for Cd and H2O2 measurements. For Cd measurement, samples were analyzed via the inductively coupled plasma-atomic emission spectrometer (IRIS/AP Optical Emission Spectrometer, Thermo Scientific Pierce, Waltham, MA, United States). For H2O2 measurement, approximately 80 mg of samples were analyzed by an Amplex Red H2O2 assay Kit (Invitrogen, Thermo Scientific Pierce, Waltham, MA, United States).
Yeast one-hybrid and yeast two-hybrid assays
For the Y1H assay, the AD fusion construct (pB42AD) was co-transformed into yeast (EGY48) with the pLacZ2μ construct containing a specific promoter, and then were selected on SD/-Trp-Ura agar plates for 72–96 h at 30°C. Subsequently, transformants of yeast were carried out using the selective medium containing raffinose, galactose, and X-gal (Amresco, Solon, OH, United States). For the Y2H assay, the AD-fusion (pGADT7) was co-transformed into yeast (AH109) with the BD-fusion (pGBKT7). Yeast (Y2H) transformants were then selected on selective medium (SD-LWHA) with or without 10 mM 3-amino-1,2,4-triazole (3-AT).
Electrophoretic mobility shift assay
For the EMSA assay, the CDS fragment of ZAT10 was cloned into the pMAL-c4X vector. The recombinant plasmid or empty vectors were then transformed into Escherichia coli BL21. 0.4 mM IPTG was added to induce the fusion proteins of MBP-ZAT10 for 16–20 h at 16°C with 200 rpm shaking in liquid LB medium. The MBP-ZAT10 protein was purified using Amylose Resin (NEB, Beijing, China). Briefly, the protein-bound beads were washed three times with CB buffer (20 mM Tris–HCl, 100 mM NaCl, 10 mM EDTA, pH 7.4). The reaction mixture was then incubated for 3 h at 4°C. Then, the proteins were eluted with elution buffer (20 mM Tris–HCl, 100 mM NaCl, 10 mM EDTA, 0.5 mM maltose, pH 7.4). Subsequently, the MBP-ZAT10 protein (2 μg) was incubated with probes using EMSA/gel-shift binding 5 × Buffer (Beyotime Biotechnology, Shanghai, China) in 20 μL reaction mixtures at 25°C for 30 min. To generate the Cy5-labeled probes, the promoter fragment of ZAT10 was synthesized (Supplementary Table 1). After that, samples were separated by 12% native polyacrylamide gels. Then the gel was visualized using a LI-COR Odyssey Infrared Imaging System to detect the fluorescent label (LI-COR, Lincoln, NE, United States).
Transient expression assays
Transient expression assays in Arabidopsis mesophyll protoplasts were performed as described previously (Yoo et al., 2007). In brief, the plasmids were isolated and purified using the EndoFree Maxi Plasmid Kit (Tiangen Biotech, Beijing, China) according to the manufacturer instructions. And then analysis of relative luciferase activity driven by indicated promoter was carried out in mesophyll protoplasts. The protoplasts were transfected with 20 μg 35S:REN-promoters:LUC and 20 μg p19-ZAT10-GFP or p19-GFP plasmids and then incubated in WI buffer for 10 h. To investigate the functional relationship between ZAT10 and FIT protein, the protoplasts were transfected with 20 μg 35S:REN-promoters:LUC plasmids, and 10 μg of p19-ZAT10-GFP, p19-FIT-GFP, or p19-GFP plasmids in different combinations to reach a total of 20 μg per transfection reaction, and then incubated in WI buffer for 10 h. The activity of firefly and control Renilla luciferase (REN) was analyzed by the Dual-Glo Luciferase Assay System (Promega, Beijing, China) after evaluating the GFP signal using fluorescence microscopy. The firefly luciferase activity was normalized to the REN luciferase activity (LUC/REN).
Transient transcription assays of N. benthamiana plants were performed as described (Liu et al., 2019a,b). In brief, the promoter fragments of ZAT6, ZAT10, FIT, IRT1, ZIP1, ZIP3, ZIP4, ZIP5, ZIP9, IREG1, IRGE2, NAS1, NAS2, MTP3, or HMA3 were cloned into the HindIII and BamHI digested pGreen-0800-LUC vector, and the CDS fragment of ZAT10 was inserted into BamHI and HindIII digested pGreenII 62-SK vector. These constructs were transformed into Agrobacterium EHA105 (pSoup), and leaves of 6 to 8-week-old N. benthamiana plants were infiltrated with suspension of Agrobacterium containing the indicated constructs (OD600 = 0.5), and then plants were transferred to a growth room for 48 h at 28 ± 2°C. The firefly luciferase luminescence was photographed after infiltrating with 1 mM luciferin (88294, Thermo Scientific Pierce, Waltham, MA, United States), and quantified using the Night SHADE LB985 system (Berthold, Stuttgart, Germany).
Bimolecular fluorescence complementation assay
Bimolecular fluorescence complementation assays were performed on Arabidopsis protoplasts. In brief, the coding fragment of FIT was inserted into the p19-YFPN vector, and the coding fragments of ZAT10 and ZAT12 were inserted into the p19-YFPC vector, respectively. Subsequently, constructs were co-transformed into Arabidopsis protoplasts and incubated for 10 h. YFP fluorescence signals were captured using a fluorescence microscope (Nikon, Tokyo, Japan).
Split luciferase complementation imaging assay
As described previously, the LCI assay was performed on N. benthamiana. In brief, the coding fragments of ZAT10 and ZAT12 were inserted into the KpnI and SalI digested pCAMBIA-nLUC vector, respectively. The coding sequence of FIT was inserted into the KpnI and SalI digested pCAMBIA-cLUC vector. Then the ZAT10-nLUC, ZAT12-nLUC, cLUC-FIT, nLUC, and cLUC constructs were transformed into Agrobacterium EHA105 as indicated combinations. The suspension (OD600 = 0.5) of Agrobacterium containing the indicated constructs was infiltrated into the leaves of 6 to 8-week-old N. benthamiana plants using a needleless syringe. After infiltration, plants were grown under 16 h light/8 h dark for 36–48 h. Leaves were then infiltrated with 1 mM luciferin (88294, Thermo Scientific Pierce, Waltham, MA, United States), and the LUC signal was captured using the LB985 Night SHADE system (Berthold, Stuttgart, Germany) with a 30 s exposure.
Gene expression analysis
For gene expression assays, RNA was extracted using the RNAprep Pure Plant Kit (Tiangen Biotech, Beijing, China). The TaKaRa PrimeScript RT-PCR Kit (TaKaRa Bio, Beijing, China) was used to synthesize cDNA following the reverse transcription protocol. Expression levels of genes were analyzed by the CFX96 Real-Time PCR System (Bio-Rad, Hercules, CA, United States). Arabidopsis UBIQUITIN 10 (UBQ10) was used as an internal control. Primers for qRT-PCR assay were listed in Supplementary Table 1.
Statistical analysis
All experiments were performed using three biological replicates. All the data were analyzed by Student’s t-test or by Tukey’s multiple comparison test. Statistically significant differences were indicated by asterisks or different letters.
Accession numbers
Sequence data from this article can be found in the Arabidopsis Information Resource (TAIR2) or GenBank/EMBL data libraries under the following accession numbers: ZAT10 (AT1G27730), ZAT6 (AT5G04340), ZAT12 (AT5G59820), ZF1 (AT5G67450), ZF2 (AT3G19580), ZF3 (AT5G43170), FIT (AT5G59820), IRT1 (AT4G19690), ZIP1 (AT3G12750), ZIP2 (AT5G59520), ZIP3 (AT2G17790), ZIP4 (AT1G10970), ZIP5 (AT1G05300), ZIP9 (AT4G33020), IRT2 (AT4G19680), NAS1 (AT5G04950), NAS2 (AT5G56080), IREG1 (AT2G38460), IREG2(AT5G03570), MTP3 (AT3G58810), HMA3 (AT4G30120), UBQ10 (AT4G05320), GmZAT10 (Glyma.04G044900), SlZAT10 (Solyc04g077980), CaZAT10 (CA00g39910), OsZAT10 (LOC_Os12g39400), and ZmZAT10 (GRMZM2G069176).
Results
The expression of ZAT10 is induced by cadmium treatment
ZAT10, a zinc-finger transcription factor, contains two C2H2-type zinc-finger domains, which are highly conserved in ZAT10 sequences from diverse plant species (Figure 1A and Supplementary Figure 1). The phylogenetic analysis of ZAT10 and its homologs in Arabidopsis showed that ZAT10 has a high degree of similarity and conservation with ZAT6 and ZAT12, which are involved in the responses to Cd stress in plants (Figure 1B; Opdenakker et al., 2012; Chen et al., 2016). Therefore, we hypothesized that ZAT10 has a potential function in the regulation of Cd tolerance in plants. To verify this hypothesis, we first investigated whether ZAT10 responds to Cd stress in plants. The qRT-PCR analysis revealed that the transcript level of ZAT10 was induced in Arabidopsis seedlings under different concentrations of Cd treatment (Figure 1C). We also examined the temporal expression pattern of ZAT10 in response to Cd exposure. As shown in Figure 1D, ZAT10 was a fast-responding gene that was induced by 1 h of Cd exposure and peaked at 6 h, followed by a significant decrease after 12 h of treatment. These results indicate that ZAT10 is involved in regulating the response to Cd stress in plants.
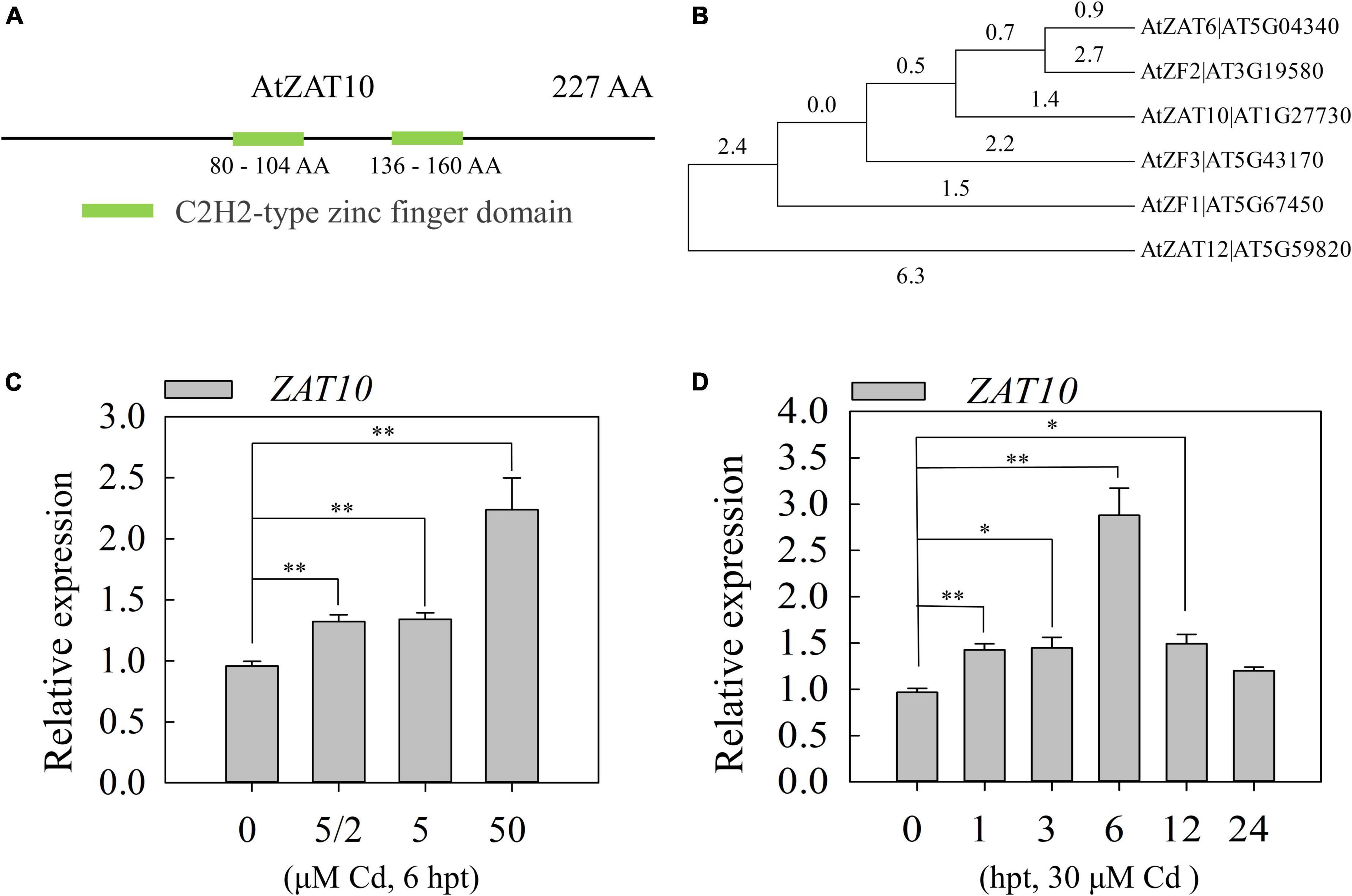
Figure 1. Molecular characterization of ZAT10 and the expression patterns of ZAT10 under cadmium stress. (A) The sites of the predicted two C2H2-type zinc-finger domains in the ZAT10 protein. (B) Phylogenetic tree of ZAT10 and its homologous members in Arabidopsis. (C,D) Relative expression levels of ZAT10 in 5-day-old wild type seedlings (C) under CdSO4 (0, 2.5, 5, and 50 μM) treatment for 6 h and (D) at 0, 1, 3, 6, 12, 24 h post treatment (hpt) with 30 μM CdSO4. Means ± SD, n = 3; *P < 0.05 or **P < 0.01, Student’s t-test.
Loss-of-function of ZAT10 lead to increased cadmium sensitivity
To characterize the function of ZAT10 in plant Cd-tolerance, we obtained the Arabidopsis T-DNA insertional mutant zat10 (SALK_054092C) from the SALK T-DNA collection (Figure 2A; Mittler et al., 2006) and generated complementary lines expressing ZAT10pro:ZAT10 in the zat10 background. Seeds of the wild type, zat10 mutant and two independent complementary lines were germinated on 1/2 MS agar plates with or without supplementary 30, 60, or 90 μM Cd for 9 days. Plants grown on 1/2 MS without Cd displayed similar plant size and root length, whereas those with Cd treatment showed suppressed plant growth and root elongation (Figures 2B–D). Compared to the wild type, the zat10 mutants were more sensitive to Cd stress (Figures 2B–D). Meanwhile, both complementary lines showed comparable root length and biomass as the wild type, demonstrating that ZAT10 is functional in Cd tolerance and can rescue the zat10 phenotype under Cd exposure (Figures 2B–D). We further tested the Cd content in the shoot and root of plants that were exposed to Cd. The wild-type and zat10 mutant plants grown on 1/2 MS agar plates without Cd for 7 days were transferred to 1/2 MS agar plates with 30 μM Cd. At indicated days post treatment (DPT), Cd concentration in the shoot and root was measured, respectively. The results showed that more Cd was accumulated in the shoot and root of zat10 seedlings compared with that in the wild type (Figures 2E,F). These results suggest that ZAT10 plays an important role in regulating Cd accumulation and tolerance in Arabidopsis.
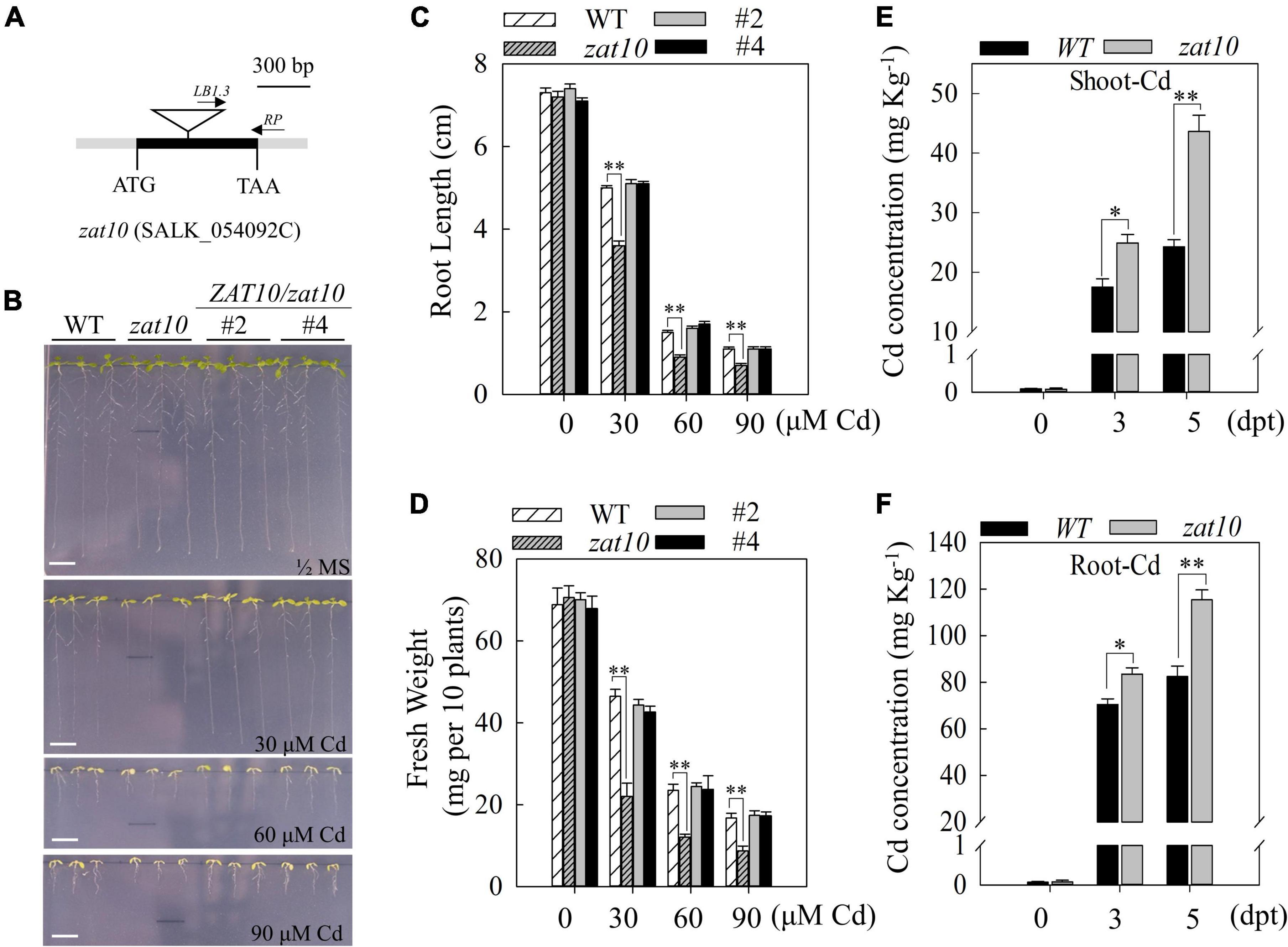
Figure 2. The zat10 loss-of-function mutant is sensitive to Cadmium stress. (A) Schematic diagram of T-DNA insertion site on the locus of ZAT10 in the zat10 (SALK_054092C) mutant. (B) Phenotypic analysis of the wild type, zat10 mutant and ZAT10pro:ZAT10/zat10 complementary lines (ZAT10/zat10 #2, #4) under Cd stress. Nine-day-old seedlings grown on 1/2 MS medium without or with CdSO4 (30, 60, and 90 μM). Bar = 1 cm. Three independent experiments were done with similar results. (C,D) Root length (C) and fresh weight (D) of the wild type, zat10 mutant, and ZAT10/zat10 #2, #4 lines under the condition described in B. Means ± SD, n = 3; **P < 0.01, Student’s t-test. (E,F) Cd concentration in shoot (E) and root (F) of the wild type and zat10 mutant under Cd stress. Seven-day-old seedlings grown on 1/2 MS medium were transferred to 1/2 MS medium without or with 30 μM CdSO4 for 0, 3, and 5 days. Means ± SD, n = 3; *P < 0.05 or **P < 0.01, Student’s t-test.
Cadmium induces more reactive oxygen species in zat10 plants
In general, Cd stress induces the accumulation of reactive oxygen species (ROS), such as superoxide radicals (O2⋅–), hydrogen peroxide (H2O2), and hydroxyl radicals (OH⋅) (Tran and Popova, 2013; Perez-Chaca et al., 2014). Thus, we applied NBT staining and DAB staining to detect the accumulation of O2⋅– and H2O2 in seedlings or leaves under Cd stress. The results showed that zat10 mutant seedlings accumulated much higher levels of O2⋅– and H2O2 than the wild type under Cd stress, while the accumulation levels of O2⋅– and H2O2 in zat10 mutant and wild type seedlings in the absence of Cd were similar (Figures 3A–C). Quantification of H2O2 content in the seedlings of wild type and zat10 plants also confirmed these results (Figure 3D). These results demonstrate that Cd treatment induces a higher level of ROS burst in zat10 seedlings, suggesting that ZAT10 is involved in the mitigation of Cd-induced ROS stress in plants. To further investigate whether Cd stress or Cd-induced ROS stress induces the expression of ZAT10, we examined the transcript level of ZAT10 in plants treated with H2O2. Interestingly, the expression level of ZAT10 in plants sharply decreased after 1 h of H2O2 treatment, implying that Cd-induced H2O2 may rapidly and significantly inhibit the expression of ZAT10 as a feedback regulation (Figure 3E).
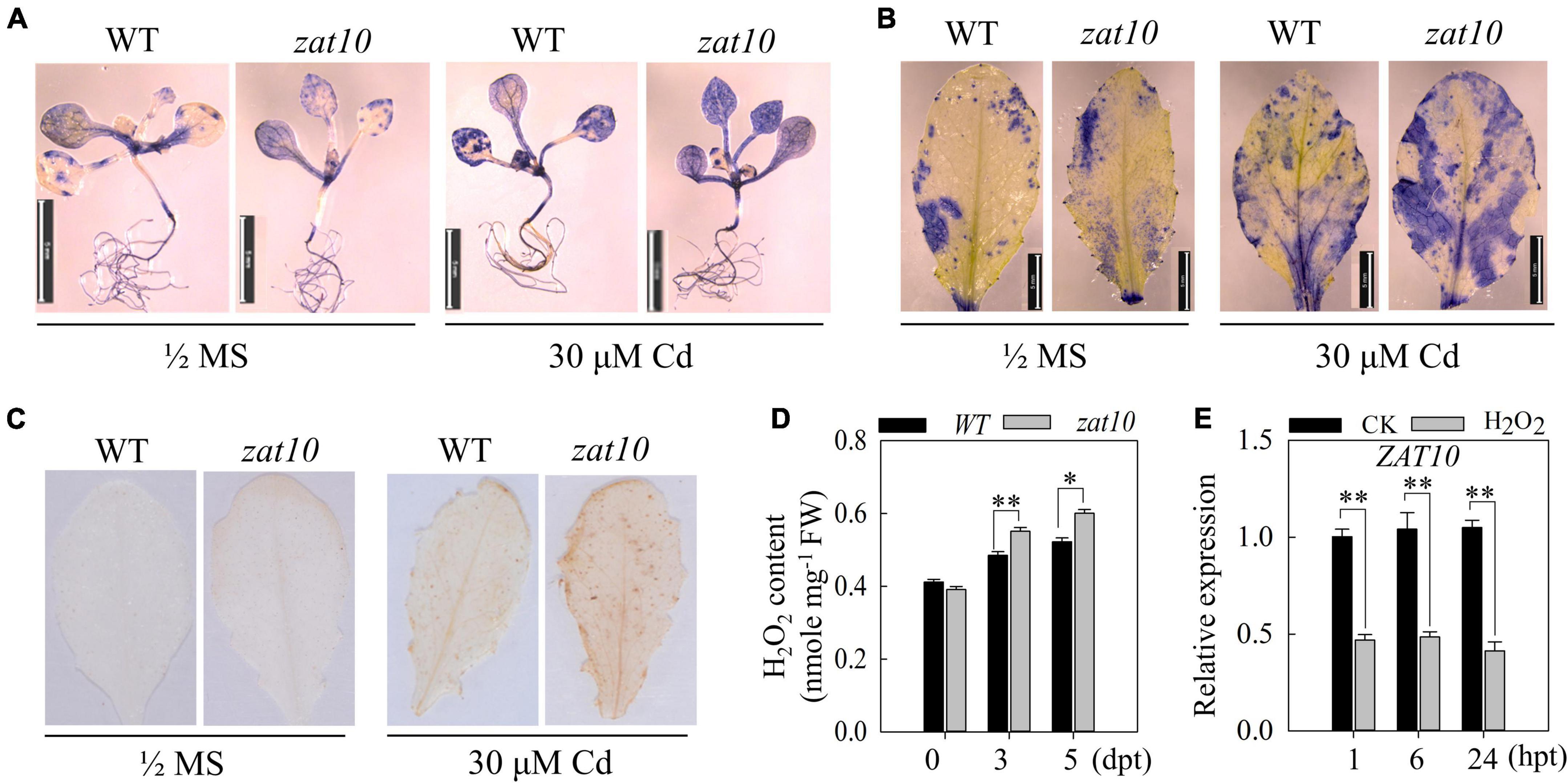
Figure 3. zat10 plants accumulate more reactive oxygen species under cadmium stress. (A,B) The 2-week-old (A) and 3-week-old (B) wild-type and zat10 plants were treated with 30 μM CdSO4 for 24 h, and then the accumulation of ROS in plants (A) and leaves (B) was detected by NBT staining. Bar = 5 mm. (C) Accumulation of H2O2 was detected in the wild type and zat10 leaves under 30 μM CdSO4 treatment for 24 h by diaminobenzidine staining. (D) H2O2 concentration in seedlings of the wild type and zat10 mutant under Cd stress. Seven-day-old seedlings grown on 1/2 MS medium were transferred to 1/2 MS medium without or with 30 μM CdSO4 for 0, 3, and 5 days, and then were harvested for H2O2 concentration measurements. Means ± SD, n = 3; *P < 0.05 or **P < 0.01, Student’s t-test. (E) Expression of ZAT10 was analyzed by qRT-PCR in the wild-type plants at 0, 6, and 24 h post treatment (hpt) with 10 μM H2O2. Means ± SD, n = 3; **P < 0.01, Student’s t-test.
ZAT10 regulates the expression of heavy metal uptake genes
To reveal how ZAT10 affects plant tolerance to Cd stress, we investigated whether ZAT10, as a transcription factor, regulates some downstream genes that may be involved in the response to Cd stress. It was previously reported that one of the main strategies for plants to defend against heavy metal stress is to reduce the uptake of heavy metals from the soil by the root system (El Rasafi et al., 2020). Therefore, we first investigated the action of ZAT10 on genes related to metal uptake in plants. Considering that some ZIP family members are involved in the uptake of metals ion, both essential metal nutrients and toxic heavy metals (Grotz et al., 1998; Pence et al., 2000; Milner et al., 2013; Zheng et al., 2018; Spielmann et al., 2020), we tested the well-studied metal transporter IRT1, which plays a critical role in plant Cd uptake (Rogers et al., 2000; Vert et al., 2002), and some ZIP genes mainly expressed in roots, such as ZIP3, ZIP4, ZIP5, and ZIP9 (Zheng et al., 2018). We applied the dual-luciferase assay in Arabidopsis protoplasts to examine the transcriptional regulation of ZAT10 on the promoters of these genes. Out of the 5 genes tested, the IRT1p-LUC reporter showed a dramatic decrease in luciferase activity when ZAT10 was co-expressed (Figure 4A). Next, a transient expression assay in N. benthamiana leaves was carried out to verify this result. Similarly, ZAT10 suppressed the transcriptional activity of the IRT1 promoter, but had no effect on the other four promoters (Figure 4B). Furthermore, the transcript levels of these genes in the wild type or zat10 mutant plants under Cd stress were tested by qRT-RCR. Consistently, it was shown that IRT1, but not other tested ZIP genes, was rapidly induced by Cd treatment in zat10 mutant plants compared with those in the wild type (Figure 4C and Supplementary Figure 2A). Together, these results suggest that ZAT10 down regulates the expression level of IRT1 in plants under Cd stress.
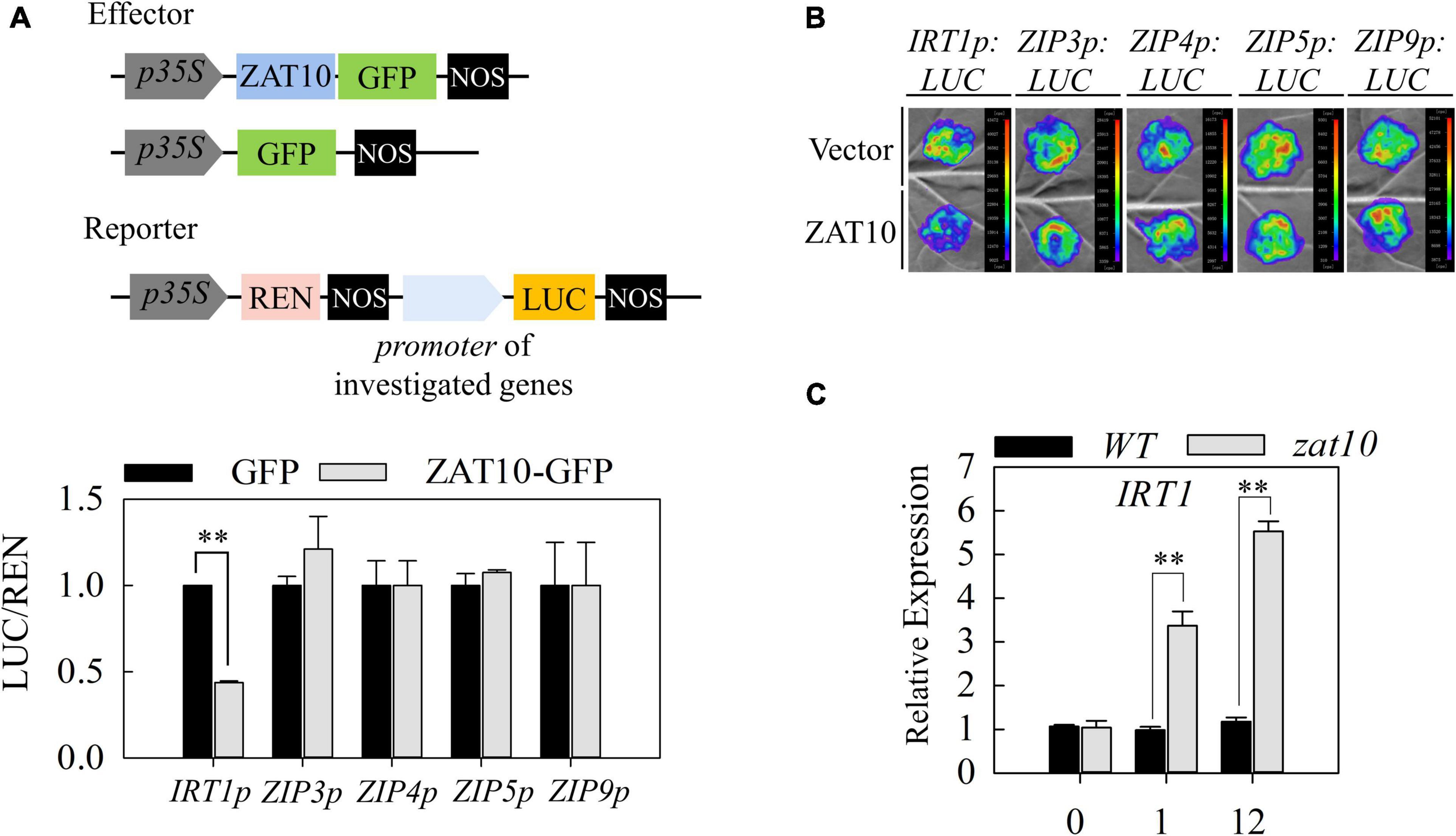
Figure 4. ZAT10 represses the expression of IRT1. (A) The schematic drawing of vectors used in dual-luciferase assays. Dual-luciferase assays in Arabidopsis protoplasts show that additional ZAT10 represses the activity of IRT1p:LUC. The REN was used as an internal control. LUC/REN ratio represents the relative activity of the IRT1, ZIP3, ZIP4, ZIP5, and ZIP9 promoters. Means ± SD, n = 3; **P < 0.01, Student’s t-test. (B) ZAT10 represses the transcriptional activity of IRT1 promoter in N. benthamiana leaves. Vectors containing IRT1p:LUC, ZIP3p:LUC, ZIP4p:LUC, ZIP5p:LUC, or ZIP9p:LUC were co-infiltrated with the empty vector or ZAT10 overexpression vector in N. benthamiana leaves as indicated. Images were taken at 48 h after infiltration. At least three replicates were measured with similar results. (C) Relative expression of IRT1 in the wild-type and zat10 mutant plants at 0, 1, and 12 h post treatment (hpt) with 30 μM CdSO4. Means ± SD, n = 3; **P < 0.01, Student’s t-test.
ZAT10 regulates the expression of heavy metal detoxification genes
In addition to reducing the uptake of Cd by roots, plants alleviate the toxicity of heavy metals to cells by chelating or sequestering them in vacuoles and vesicles (El Rasafi et al., 2020). A number of heavy metal detoxification genes have been reported previously. For example, NAS1 and NAS2 play a key role in the synthesis of NA, an important chelator in response to heavy metals (Kim et al., 2005; Klatte et al., 2009); IREG1, IREG2, IRT2, MTP3, and HMA3 are all involved in the transport of heavy metals into vesicles and vesicles (Arrivault et al., 2006; Schaaf et al., 2006; Morel et al., 2009; Vert et al., 2009). Thus, we evaluated whether these genes are regulated by ZAT10 through the dual-luciferase assays in Arabidopsis protoplasts. The results showed that ZAT10 up-regulated the transcriptional activity of NAS1p, NAS2p, IRT2p, and MTP3p, but had no effect on IREG1p, IREG2p, and HMA3p (Figure 5A). Consistent with these results, transient expression assays in N. benthamiana leaves showed that co-expression of ZAT10 protein significantly increased the transcriptional activation of the LUC reporter gene driven by NAS1p, NAS2p, IRT2p, and MTP3p (Figure 5B), indicating that ZAT10 positively regulates the expression of these genes.
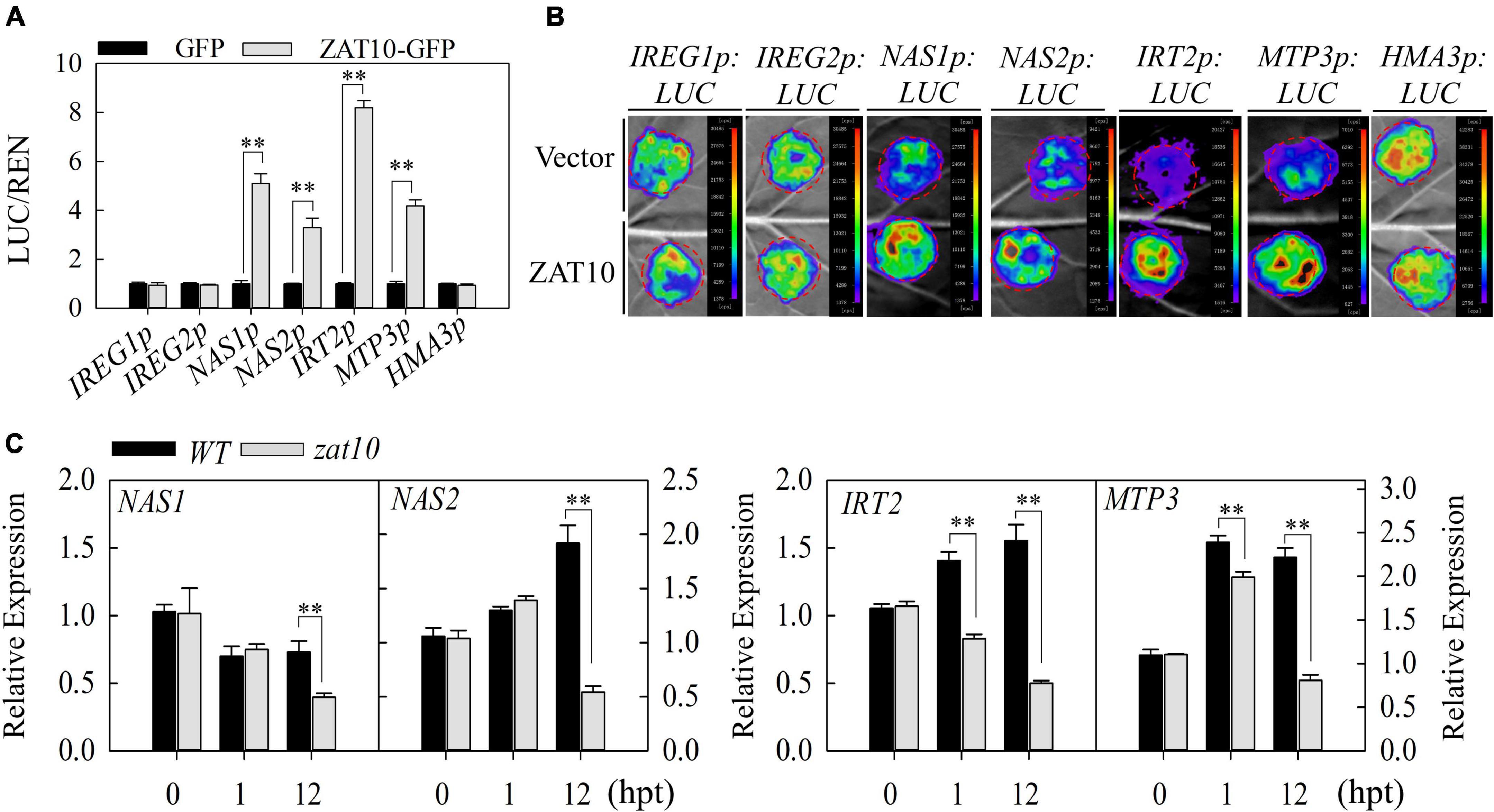
Figure 5. ZAT10 activates the expression of NAS1, NAS2, IRT2, and MTP3. (A) Dual-luciferase assays in Arabidopsis protoplasts show that additional ZAT10 enhances the activity of NAS1p:LUC, NAS2p:LUC, IRT2p:LUC, and MTP3p:LUC in protoplast transient expression assays. The REN was used as an internal control. LUC/REN ratio represents the relative activity of the IREG1, IREG2, NAS1, NAS2, IRT2, MTP3, and HMA3 promoters. Means ± SD, n = 3; **P < 0.01, Student’s t-test. (B) ZAT10 enhances the transcriptional activity of NAS1, NAS2, IRT2, and MTP3 promoters in N. benthamiana leaves. The IREG1p:LUC, IREG2p:LUC, NAS1p:LUC, NAS2p:LUC, MTP3p:LUC, and HMA3p:LUC were co-infiltrated with empty vector or ZAT10 overexpression vector in N. benthamiana leaves as indicated. Images were taken at 48 h after infiltration. At least three replicates were measured with similar results. (C) Relative expression levels of NAS1, NAS2, IRT2, and MTP3 in the wild-type and zat10 mutant plants at 0, 1, and 12 h post treatment (hpt) with 30 μM CdSO4. Means ± SD, n = 3; **P < 0.01, Student’s t-test.
To verify the above results, we further tested the expression levels of these seven genes in wild type and zat10 plants under Cd stress. qRT-PCR analysis revealed a similar degree of decrease in NAS1 expression in both wild-type and zat10 mutant plants after 1 h of Cd treatment, but the expression was further suppressed in zat10 plants after 12 h of treatment (Figure 5C). Moreover, the expression levels of NAS2, IRT2, and MTP3 in the wild type were significantly induced by 1 h of Cd treatment, and the expression levels continued to increase or remained high after 12 h of Cd treatment (Figure 5C). By contrast, the expression of NAS2 and MTP3 in zat10 mutant plants were also up-regulated after 1 h of Cd treatment, but they were considerably decreased after 12 h of treatment (Figure 5C). Meanwhile, IRT2 in zat10 mutant plants was inhibited after 1 h of Cd treatment, and this inhibition was enhanced with the duration of treatment (Figure 5C). In addition, in accordance with the transcriptional activation assays, there was no significant difference in the expression levels of IREG1, IREG2, and HMA3 between the wild-type and zat10 mutant plants, either under Cd-free or Cd-exposed conditions (Supplementary Figure 2B). Taken together, these results reveal that ZAT10 positively regulates the transcriptional activity of some detoxification genes (NAS1, NAS2, IRT2, and MTP3) under Cd stress, thereby enhancing Cd tolerance in plants.
ZAT10 interacts with FER-like iron deficiency-induced transcription and co-regulates the transcriptional expression of IRT1
We further performed Y1H assays to test whether these downstream genes are directly regulated by ZAT10. However, it is unfortunate that ZAT10 could not bind to the promoters of these genes in yeast (Supplementary Figure 3), suggesting that ZAT10 may not directly regulate the expression of these genes.
Previous research has shown that FIT is not only a key regulator of iron uptake and homeostasis, but also plays a role in Cd tolerance in plants, as an important factor in the interrelationship between Fe and Cd in plants (Wu et al., 2012). Specifically, FIT interacts with bHLH transcription factors to regulate Fe/Cd uptake genes IRT1 (Wang et al., 2007; Yuan et al., 2008; Wu et al., 2012), as well as HMA3, MTP3, IREG2, IRT2, NAS1, and NAS2, which are involved in Fe/Cd homeostasis in cells (Wu et al., 2012). Furthermore, a recent study showed that ZAT12, a homolog of ZAT10, negatively regulates FIT transcription and directly interacts with FIT proteins to enhance the stability of FIT proteins (Ben Daniel et al., 2016; Le et al., 2016). Therefore, we wondered whether ZAT10 regulates these downstream genes through modulating FIT.
First, we examined the function of ZAT10 in the transcriptional regulation of FIT through our transient expression system in Arabidopsis protoplasts. The results showed that ZAT10 did not affect the transcriptional activity of FIT (Supplementary Figure 4). Next, the yeast two-hybrid assay showed that ZAT10 physically interacted with FIT in yeast cells (Figure 6A). This interaction was further verified by the split luciferase complementation imaging assay (LCI) in N. benthamiana leaves and the bimolecular fluorescence complementation assay (BiFC) in Arabidopsis protoplasts (Figures 6B,C). ZAT12, which has been previously reported to interact with FIT, was applied as a positive control in these experiments (Figures 6A–C; Ben Daniel et al., 2016; Le et al., 2016). These results suggest that ZAT10 may coordinate with FIT to regulate downstream genes.
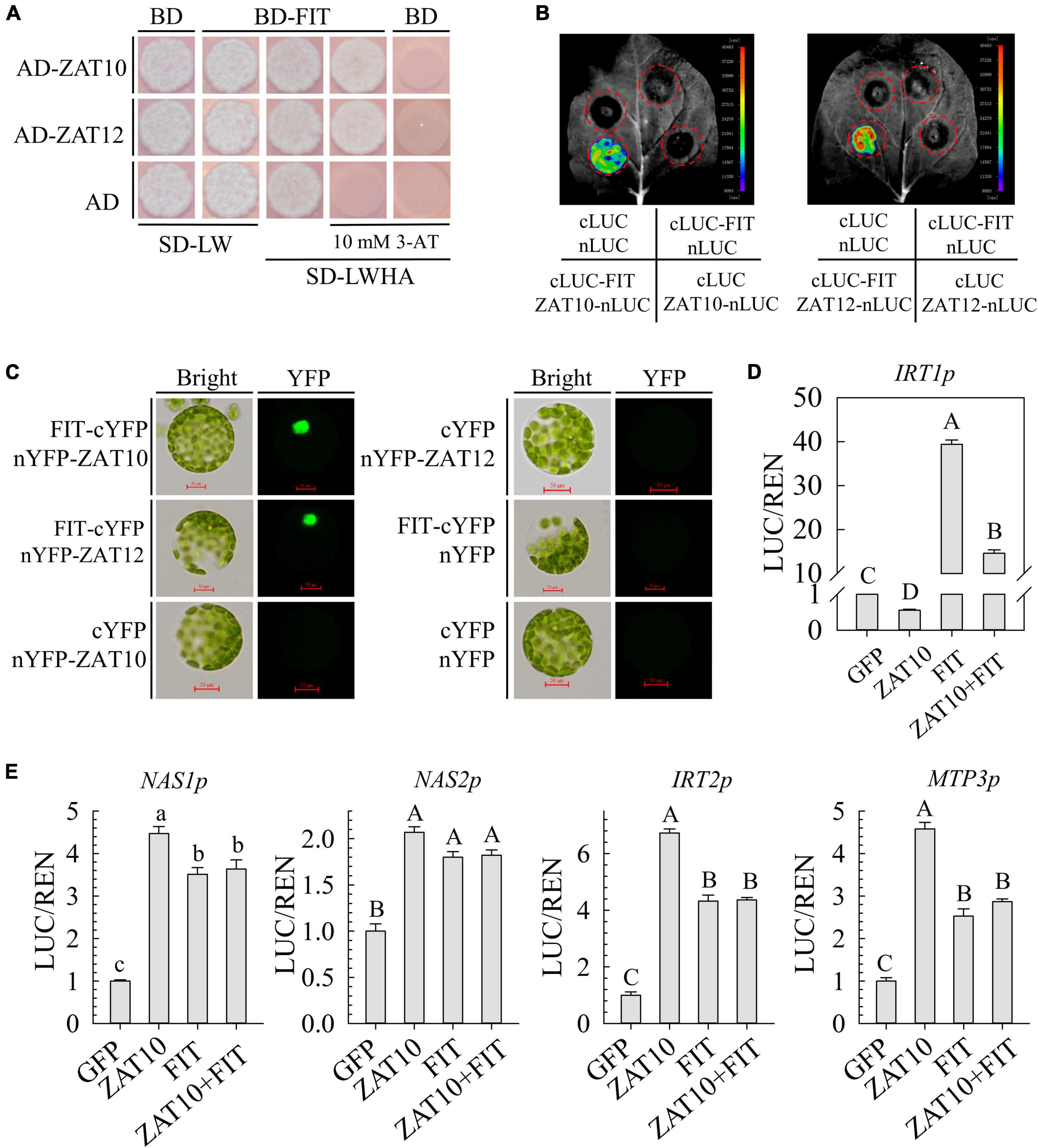
Figure 6. ZAT10 interacts with FER-like iron deficiency-induced transcription and co-regulates the transcriptional expression of IRT1. (A) ZAT10 interacts with FIT in yeast. 10 mM 3-AT was applied to inhibit the self-activation. ZAT12 was applied as a positive control. (B) luciferase complementation imaging assay confirms the interactions between FIT and ZAT10 or ZAT12 in N. benthamiana leaves. At least three replicates were observed with similar results. (C) BiFC assay confirms the interactions between FIT and ZAT10 or ZAT12 in Arabidopsis mesophyll cell protoplasts. At least three replicates were observed with similar results. Bar = 50 μm. (D) ZAT10 represses the transcriptional activation of FIT on IRT1p:LUC in Arabidopsis protoplasts. (E) ZAT10 has no effect on the transcription activity of FIT on NAS1p:LUC, NAS2p:LUC, IRT2p:LUC, and MTP3p:LUC in Arabidopsis protoplasts. Means ± SD, n = 3; Significant differences are indicated by letters (lowercase P < 0.05 or uppercase P < 0.01. Tukey’s multiple comparisons test).
Then, transient expression assays in Arabidopsis protoplasts were carried out to look into the relationship between ZAT10 and FIT in the transcriptional regulation of downstream genes. The quantification of LUC activity relative to REN showed that the expression of the IRT1p:LUC reporter gene was independently suppressed by ZAT10 and induced by FIT (Figure 6D). IRT1p:LUC reporter gene was also induced when ZAT10 and FIT were co-expressed, but to a significantly lower extent than that induced by FIT alone (Figure 6D). Furthermore, the expression of NAS1p:LUC, NAS2p:LUC, IRT2p:LUC, and MTP3p:LUC reporter genes was induced by either ZAT10 or FIT (Figure 6E). However, it should be noted that simultaneous expression with ZAT10 and FIT did not further raise the expression levels of these reporter genes; rather, they remained at levels similar to those observed when FIT was expressed alone (Figure 6E).
Feedback regulation of ZAT10 expression
Given that ZAT6, ZAT10, and ZAT12 are conserved in their sequences and their functions may be linked during abiotic stress (Mittler et al., 2006; Shi et al., 2014; Chen et al., 2016), we further tested the effect of ZAT10 on the activity of ZAT6, ZAT10, and ZAT12 promoters. Dual-luciferase assay in Arabidopsis protoplast showed that ZAT10 acted as a feedback regulator inhibiting the activity of ZAT10 promoter, while it had no effect on ZAT6 and ZAT12 promoter activities (Figure 7A). To verify this result, transient expression assays were carried out. ZAT6p:LUC, ZAT10p:LUC, or ZAT12p:LUC reporter constructs with or without 35S:ZAT10 were infiltrated into N. benthamiana leaves. As shown in Figure 7B, only the reporter genes driven by the ZAT10 promoter were significantly repressed by supplementary ZAT10, suggesting that ZAT10 indeed suppresses the expression of ZAT10. In addition, the Y1H (Figure 7C) and EMSA (Figure 7D) assays showed that ZAT10 directly bind to the ZAT10 promoter, further confirming the negative transcriptional regulation of ZAT10 on itself.
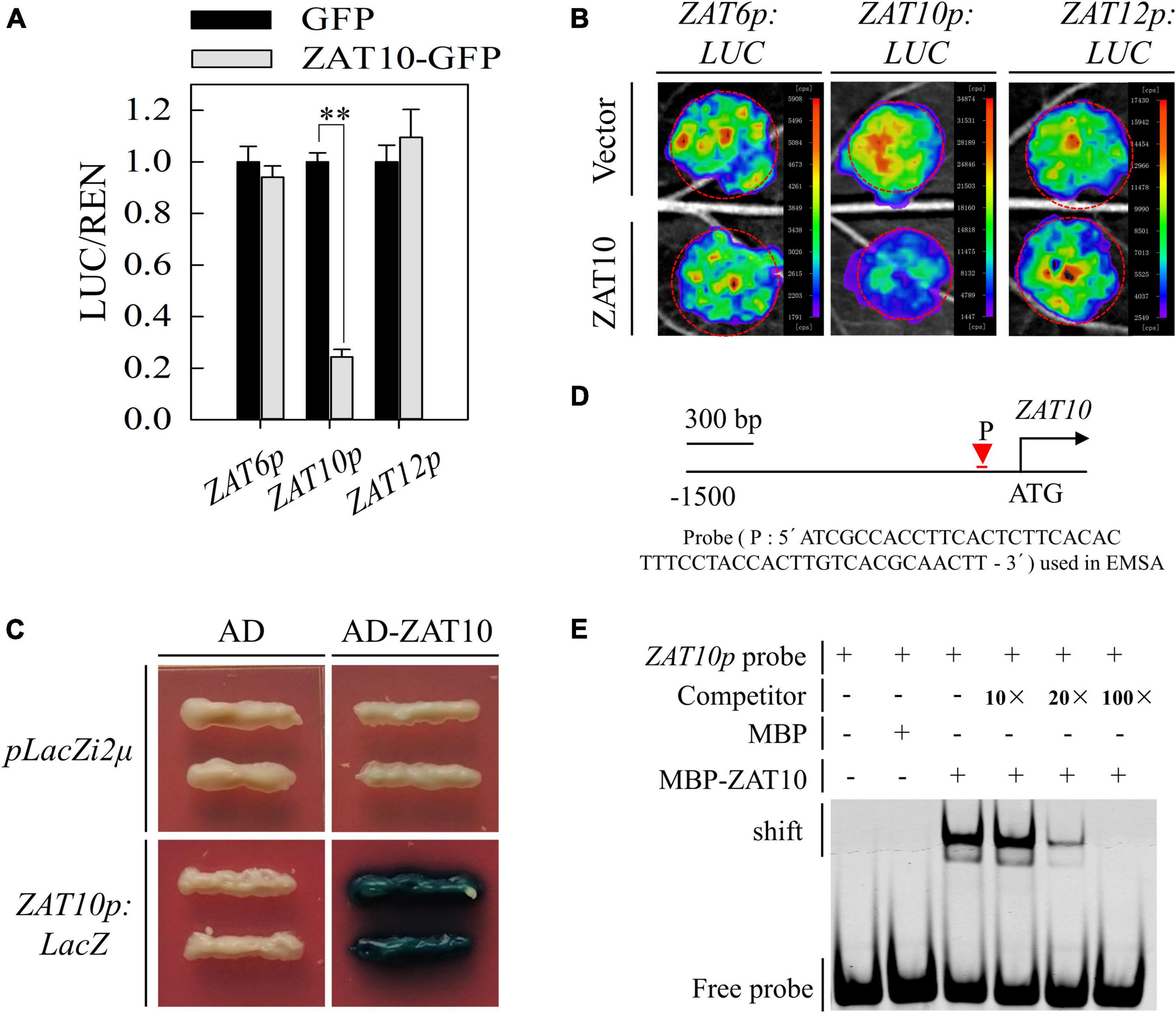
Figure 7. ZAT10 directly binds to its own promoter and represses its expression. (A) ZAT10 represses ZAT10p:LUC in protoplast transient expression assays. The renilla was used as an internal control. LUC/REN ratio represents the relative activity of the ZAT6, ZAT10, and ZAT12 promoters. Means ± SD, n = 3; **P < 0.01, Student’s t-test. (B) Transient expression assays of luminescence intensity show that ZAT10 represses the transcriptional activity of ZAT10 promoter in N. benthamiana leaves. The ZAT6p:LUC, ZAT10p:LUC, and ZAT12p:LUC were co-infiltrated with empty vector or ZAT10 overexpression vector in N. benthamiana leaves as indicated. Images were taken at 48 h after infiltration. At least three replicates were measured with similar results. (C) Yeast one-hybrid assay shows that ZAT10 directly bind to the ZAT10 promoter. (D) Illustration of the probe (P) used in electrophoretic mobility shift assay. (E) EMSA analysis shows the direct binding of ZAT10 to the ZAT10 promoter.
Discussion
Cadmium is a major heavy metal pollutant in soil that is toxic to plants. It severely impairs plant growth and development, and threatens animals and people via the food chain. Previous research has shown that there are two different strategies for plants to respond to Cd stress, either by reducing the uptake of Cd by roots, or by chelating and sequestering Cd within cells to reduce the toxicity of Cd. In the present study, we showed that ZAT10 is up-regulated by Cd exposure (Figure 1), while the zat10 mutant is more sensitive to Cd stress (Figures 2, 3). Further experiments revealed that ZAT10 represses the transcriptional activation of IRT1 to reduce Cd uptake in plants (Figure 4), and induces the expression of detoxification genes to reduce Cd toxicity by promoting Cd segregation, leading to enhanced tolerance to Cd (Figure 5). We also demonstrated that ZAT10 interacts with FIT. In addition, we identified a negative feedback regulation of the ZAT10 protein on its own transcriptional activation (Figure 7).
Previous studies have shown that the uptake and accumulation of Cd and a variety of other elements in plants are interrelated (Bao et al., 2010; Zhu et al., 2012; He et al., 2017b; Cheng et al., 2020; Meng et al., 2022). The Fe deficiency condition promotes Cd uptake in different plants (Bao et al., 2010). IRT1, regulated by FIT, is a key gene controlling Fe uptake (Yuan et al., 2008; Wang et al., 2013). IRT1 is induced by Fe deficiency circumstances to improve Fe uptake and also promote the absorption of other metal ions, suggesting that IRT1 is not only a transporter of Fe but also plays an important role in the uptake of other metals by plants (Vert et al., 2002; He et al., 2017a). Many studies have shown that additional Fe supply under Cd stress significantly inhibits IRT1 expression, thereby reducing Cd uptake and Cd accumulation and alleviating the suppression of plant root elongation by Cd (Clemens, 2006; Wu et al., 2012; Fan et al., 2014; He et al., 2017a). However, a recent study found that application of Fe increased the expression of IRT1 under Cd stress and still alleviated the toxicity of Cd to the root system (Meng et al., 2022). Regardless, these results suggest the presence of Fe-Cd uptake competition in plants. Based on these studies, we hypothesized that due to the competition between Cd and Fe in uptake, Cd exposure leads to the activation of Fe deficiency signaling pathways in plants and deficiency-responsive genes, such as IRT1, are induced to promote Fe uptake. However, these genes conversely lead to more Cd uptake and exacerbate the toxic effects of Cd on plants. Our findings revealed that ZAT10 down-regulated IRT1 expression and suppressed the induction level of IRT1 transcription by FIT (Figures 4, 6D). Thus, we proposed that Cd-induced ZAT10 can reduce Cd uptake by breaking the vicious cycle of “Cd stress-FIT-IRT1 induction-more Cd uptake” to alleviate the Cd hijacking of the Fe transporter. Consistent with this notion, the zat10 mutant plants displayed a significantly higher Cd accumulation in mutant plants under Cd stress than that in the wild-type plants (Figures 2E,F).
In addition to responding to Cd stress by regulating Cd uptake, plants can also enhance Cd tolerance through detoxification, which involves the translocation and sequestration of Cd in the plant. It has been reported that Cd treatment significantly reduced Fe transport from roots to shoots in plants, suggesting that Cd transport also involves competition with Fe for transport factors (Yao et al., 2018). Previous studies have demonstrated that FIT coordinates with bHLHs (bHLH38, bHLH39, bHLH100, and bHLH101) to activate the transcription of NAS1 and NAS2, which regulate the synthesis of important metal chelator nicotiananamine (NA). On the other hand, FIT promotes the sequestration of heavy metals in vacuoles and vesicles in Arabidopsis by regulating HMA3, MTP3, IREG2, and IRT2 (Yuan et al., 2008; Wu et al., 2012; Wang et al., 2013). In this study, we also found that ZAT10 activated the transcription of NAS1, NAS2, MTP3, and IRT2 under Cd stress (Figure 5), indicating ZAT10 could enhance the Cd chelation and sequestration. Taken together, ZAT10 can simultaneously suppress the expression of Cd uptake related genes and enhance the expression of Cd segregation related genes, indicating that its regulatory mechanisms may differ among genes with different functions.
ZAT10 does not bind to the promoter of these downstream genes in yeast (Supplementary Figure 3), suggesting that ZAT10 may indirect regulate downstream genes through other proteins. Our investigation revealed that ZAT10 interacts with FIT (Figures 6A–C), and all of the ZAT10-regulated downstream genes identified in this study are also regulated by FIT (Figures 6D,E). However, the regulatory relationship between them remains unclear. For the Cd uptake related gene IRT1, additional ZAT10 could suppress the expression induced by FIT (Figure 6D). In contrast, for Cd segregation-related genes, the simultaneous action of ZAT10 and FIT still showed similar levels of activation as FIT alone did, suggesting that although ZAT10 interacts with FIT, additional ZAT10 does not affect the regulation of these genes by FIT (Figure 6E). One possible explanation is that the interaction between FIT and ZAT10 affects the regulation of downstream genes by FIT. However, based on our current data, we cannot exclude the possibility that ZAT10 regulates downstream genes through other pathways independent of FIT or that a combination of these two mechanisms exists for different target genes.
Moreover, we identified two negative feedback regulatory pathways for ZAT10. First, we found that Cd stress induces H2O2 accumulation in plants, and the accumulated H2O2 negatively regulates ZAT10 expression (Figure 3). Similarly, previous studies reported that H2O2 decreases the Fe-deficiency-induced FIT expression and suppresses the induction of FIT in zat12 mutant plants, but induces more FIT and ZAT12 protein accumulation (Le et al., 2016). These results indicate that H2O2 is an important signal factor in the regulatory pathway of metal ion uptake and transport in plants. Secondly, the expression of ZAT10 was induced by Cd stress, and ZAT10 protein could directly bind to the promoter of ZAT10 to suppress its transcription as the feedback regulation loop (Figure 7). These two negative regulatory pathways protect plants from the over-response to Cd stress and may explain why the ZAT10 expression decreased after reaching a peak at 6 h of Cd treatment (Figure 1D). Furthermore, homology analysis and sequence alignment showed that ZAT10 in different crops, such as soybean, rice, maize, and pepper, are highly homologous to At ZAT10 (Supplementary Figure 1), suggesting that their functions may be highly conserved. Therefore, ZAT10 may have potential applications in the improvement of Cd tolerance in crops.
In summary, in this study, we demonstrated that ZAT10 has dual functions in plants in response to Cd stress. ZAT10 is involved in the regulation of both key genes for Cd uptake and those for Cd chelation and sequestration, thus enhancing the resistance of plants to Cd stress. The data presented here show that ZAT10 interacts with FIT, but the regulatory relationship between them on downstream genes remains unclear, hence further investigation is merited. In addition, we reported a negative feedback regulation loop of ZAT10. Based on our results, we proposed a brief model of the regulatory mechanism by which ZAT10 regulates plant responses to Cd stress (Figure 8). Taken together, our results provide new perspectives into the molecular function of ZAT10 in plants under Cd stress and ZAT10 is a potential candidate gene for improving cadmium tolerance in plants.
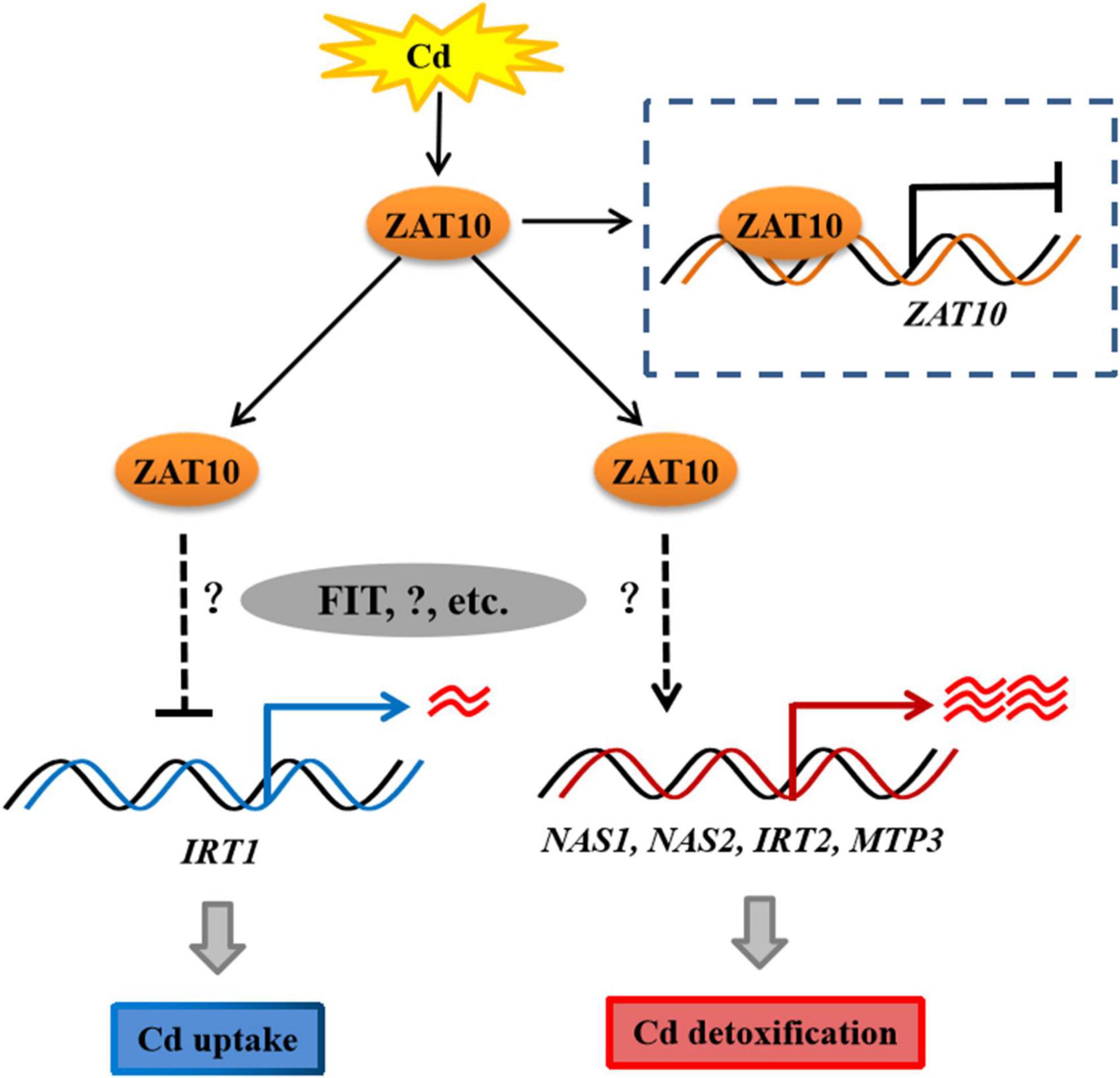
Figure 8. A schematic model depicting the role of ZAT10 in regulating cadmium tolerance in Arabidopsis. ZAT10 is induced by Cd stress, which in turn suppresses its own expression. ZAT10 negatively regulate the expression of the Cd uptake gene IRT1, leading to a reduction in Cd uptake in plants. ZAT10 also promotes the expression of the Cd detoxification genes NAS1, NAS2, IRT2, and MTP3, to enhance the detoxification of Cd in plants. Dashed lines with a question mark denote unclear regulatory mechanisms.
Data availability statement
The original contributions presented in this study are included in the article/Supplementary material, further inquiries can be directed to the corresponding author.
Author contributions
FD and XL designed the research. FD, YL, JL, and XL performed the experiments. FD, YW, SD, and XL wrote the manuscript. All authors analyzed the data, read and approved the manuscript.
Funding
This work was supported by the Basic and Applied Basic Research Foundation of Guangdong Province (2019A1515110239), the China Postdoctoral Science Foundation (2020M672648), and the National Natural Science Foundation of China (32001852).
Conflict of interest
The authors declare that the research was conducted in the absence of any commercial or financial relationships that could be construed as a potential conflict of interest.
Publisher’s note
All claims expressed in this article are solely those of the authors and do not necessarily represent those of their affiliated organizations, or those of the publisher, the editors and the reviewers. Any product that may be evaluated in this article, or claim that may be made by its manufacturer, is not guaranteed or endorsed by the publisher.
Supplementary material
The Supplementary Material for this article can be found online at: https://www.frontiersin.org/articles/10.3389/fpls.2022.994100/full#supplementary-material
Supplementary Figure 1 | Phylogenetic analysis and sequence alignment of ZAT10 in different plant species.
Supplementary Figure 2 | Expression of ZIP3, ZIP4, ZIP5, ZIP9, IREG1, IREG2, and HMA3 in the wild-type and zat10 mutant plants under cadmium stress.
Supplementary Figure 3 | Yeast one-hybrid assay showing that ZAT10 does not bind to the promoters of IRT1, NAS1, NAS2, IRT2, and MTP3.
Supplementary Figure 4 | ZAT10 has no effect on the activity of FITp:LUC in protoplast transient expression assays.
Supplementary Table 1 | List of the primers used in this study.
Footnotes
References
Alonso, J. M., Stepanova, A. N., Leisse, T. J., Kim, C. J., Chen, H. M., Shinn, P., et al. (2003). Genome-wide Insertional mutagenesis of Arabidopsis thaliana. Science 301, 653–657. doi: 10.1126/science.1086391
Antoniadis, V., Levizou, E., Shaheen, S. M., Ok, Y. S., Sebastian, A., Baum, C., et al. (2017). Trace elements in the soil-plant interface: Phytoavailability, translocation, and phytoremediation-A review. Earth-Sci. Rev. 171, 621–645. doi: 10.1016/j.earscirev.2017.06.005
Arrivault, S., Senger, T., and Kramer, U. (2006). The Arabidopsis metal tolerance protein AtMTP3 maintains metal homeostasis by mediating Zn exclusion from the shoot under Fe deficiency and Zn oversupply. Plant J. 46, 861–879. doi: 10.1111/j.1365-313X.2006.02746.x
Bae, J., Benoit, D. L., and Watson, A. K. (2016). Effect of heavy metals on seed germination and seedling growth of common ragweed and roadside ground cover legumes. Environ. Pollut. 213, 112–118. doi: 10.1016/j.envpol.2015.11.041
Bao, T., Sun, L. N., and Sun, T. H. (2010). Evaluation of Iron on Cadmium Uptake by Tomato, Morel and Leaf Red Beet in Hydroponic Culture. J. Plant Nutr. 33, 713–723. doi: 10.1080/01904160903575931
Ben Daniel, B. H., Cattan, E., Wachtel, C., Avrahami, D., Glick, Y., Malichy, A., et al. (2016). Identification of novel transcriptional regulators of Zat12 using comprehensive yeast one-hybrid screens. Physiol. Plant. 157, 422–441. doi: 10.1111/ppl.12439
Chen, J., Yang, L. B., Yan, X. X., Liu, Y. L., Wang, R., Fan, T. T., et al. (2016). Zinc-Finger Transcription Factor ZAT6 Positively Regulates Cadmium Tolerance through the Glutathione-Dependent Pathway in Arabidopsis. Plant Physiol. 171, 707–719. doi: 10.1104/pp.15.01882
Cheng, Y. R., Bao, Y. J., Chen, X., Yao, Q., Wang, C., Chai, S. Y., et al. (2020). Different nitrogen forms differentially affect Cd uptake and accumulation in dwarf Polish wheat (Triticum polonicum L.) seedlings. J. Hazardous Materials 400:123209. doi: 10.1016/j.jhazmat.2020.123209
Clemens, S. (2006). Toxic metal accumulation, responses to exposure and mechanisms of tolerance in plants. Biochimie 88, 1707–1719. doi: 10.1016/j.biochi.2006.07.003
Dang, F. F., Lin, J. H., Chen, Y. P., Li, G. X., Guan, D. Y., Zheng, S. J., et al. (2019). A feedback loop between CaWRKY41 and H2O2 coordinates the response to Ralstonia solanacearum and excess cadmium in pepper. J. Exper. Bot. 70, 1581–1595. doi: 10.1093/jxb/erz006
Dang, F. F., Wang, Y. N., Yu, L., Eulgem, T., Lai, Y., Liu, Z. Q., et al. (2013). CaWRKY40, a WRKY protein of pepper, plays an important role in the regulation of tolerance to heat stress and resistance to Ralstonia solanacearum infection. Plant Cell Environ. 36, 757–774. doi: 10.1111/pce.12011
Ding, F., Ren, L. M., Xie, F., Wang, M. L., and Zhang, S. X. (2022). Jasmonate and Melatonin Act Synergistically to Potentiate Cold Tolerance in Tomato Plants. Front. Plant Sci. 12:763284. doi: 10.3389/fpls.2021.763284
Ding, F., Wang, C., Xu, N., Wang, M. L., and Zhang, S. X. (2021). Jasmonic acid-regulated putrescine biosynthesis attenuates cold-induced oxidative stress in tomato plants. Sci. Horticulturae 288:110373. doi: 10.1016/j.scienta.2021.110373
El Rasafi, T., Oukarroum, A., Haddioui, A., Song, H., Kwon, E. E., Bolan, N., et al. (2020). Cadmium stress in plants: A critical review of the effects, mechanisms, and tolerance strategies. Crit. Rev. Environ. Sci. Technol. 52, 675–726. doi: 10.1080/10643389.2020.1835435
Fan, S. K., Fang, X. Z., Guan, M. Y., Ye, Y. Q., Lin, X. Y., Du, S. T., et al. (2014). Exogenous abscisic acid application decreases cadmium accumulation in Arabidopsis plants, which is associated with the inhibition of IRT1-mediated cadmium uptake. Front. Plant Sci. 5:110373. doi: 10.3389/fpls.2014.00721
Gallego, S. M., Pena, L. B., Barcia, R. A., Azpilicueta, C. E., Lannone, M. F., Rosales, E. P., et al. (2012). Unravelling cadmium toxicity and tolerance in plants: Insight into regulatory mechanisms. Environ. Exper. Bot. 83, 33–46. doi: 10.1016/j.envexpbot.2012.04.006
Geng, N., Wu, Y. C., Zhang, M., Tsang, D. C. W., Rinklebe, J., Xia, Y. F., et al. (2019). Bioaccumulation of potentially toxic elements by submerged plants and biofilms: A critical review. Environ. Int. 131:105015. doi: 10.1016/j.envint.2019.105015
Grotz, N., Fox, T. C., Connolly, E., Park, W., and Eide, D. (1998). Identification of a Family of Zinc Transporter Genes from Arabidopsis that Respond to Zinc Deficiency. Proc. Natl. Acad. Sci. 95, 7220–7224. doi: 10.1073/pnas.95.12.7220
He, S. Y., Yang, X. E., He, Z., and Baligar, V. C. (2017a). Morphological and Physiological Responses of Plants to Cadmium Toxicity: A Review. Pedosphere 27, 421–438. doi: 10.1016/S1002-0160(17)60339-4
He, X. L., Fan, S. K., Zhu, J., Guan, M. Y., Liu, X. X., Zhang, Y. S., et al. (2017b). Iron supply prevents Cd uptake in Arabidopsis by inhibiting IRT1 expression and favoring competition between Fe and Cd uptake. Plant Soil 416, 453–462. doi: 10.1007/s11104-017-3232-y
Kim, S., Takahashi, M., Higuchi, K., Tsunoda, K., Nakanishi, H., Yoshimura, E., et al. (2005). Increased nicotianamine biosynthesis confers enhanced tolerance of high levels of metals, in particular nickel, to plants. Plant Cell Physiol. 46:1809–1818. doi: 10.1093/pcp/pci196
Klatte, M., Schuler, M., Wirtz, M., Fink-Straube, C., Hell, R., and Bauer, P. (2009). The Analysis of Arabidopsis Nicotianamine Synthase Mutants Reveals Functions for Nicotianamine in Seed Iron Loading and Iron Deficiency Responses. Plant Physiol. 150, 257–271. doi: 10.1104/pp.109.136374
Le, C. T. T., Brumbarova, T., Ivanov, R., Stoof, C., Weber, E., Mohrbacher, J., et al. (2016). ZINC FINGER OF ARABIDOPSIS THALIANA12 (ZAT12) Interacts with FER-LIKE IRON DEFICIENCY- INDUCED TRANSCRIPTION FACTOR (FIT) Linking Iron Deficiency and Oxidative Stress Responses. Plant Physiol. 170, 540–557. doi: 10.1104/pp.15.01589
Lingam, S., Mohrbacher, J., Brumbarova, T., Potuschak, T., Fink-Straube, C., Blondet, E., et al. (2011). Interaction between the bHLH Transcription Factor FIT and ETHYLENE INSENSITIVE3/ETHYLENE INSENSITIVE3-LIKE1 Reveals Molecular Linkage between the Regulation of Iron Acquisition and Ethylene Signaling in Arabidopsis. Plant Cell 23, 1815–1829. doi: 10.1105/tpc.111.084715
Liu, Y., Wei, H. B., Ma, M. D., Li, Q. Q., Kong, D. X., Sun, J., et al. (2019a). Arabidopsis FHY3 and FAR1 Regulate the Balance between Growth and Defense Responses under Shade Conditions. Plant Cell 31, 2089–2106. doi: 10.1105/tpc.18.00991
Liu, Y. Y., Du, M. M., Deng, L., Shen, J. F., Fang, M. M., Chen, Q., et al. (2019b). MYC2 Regulates the Termination of Jasmonate Signaling via an Autoregulatory Negative Feedback Loop. Plant Cell 31, 106–127. doi: 10.1105/tpc.18.00405
Lombi, E., Tearall, K. L., Howarth, J. R., Zhao, F. J., Hawkesford, M. J., and McGrath, S. P. (2002). Influence of iron status on cadmium and zinc uptake by different ecotypes of the hyperaccumulator Thlaspi caerulescens. Plant Physiol. 128, 1359–1367. doi: 10.1104/pp.010731
Meng, X. X., Li, W. F., Shen, R. F., and Lan, P. (2022). Ectopic expression of IMA small peptide genes confers tolerance to cadmium stress in Arabidopsis through activating the iron deficiency response. J. Hazard. Mater. 422:126913. doi: 10.1016/j.jhazmat.2021.126913
Milner, M. J., Seamon, J., Craft, E., and Kochian, L. V. (2013). Transport properties of members of the ZIP family in plants and their role in Zn and Mn homeostasis. J. Exper. Bot. 64, 369–381. doi: 10.1093/jxb/ers315
Mittler, R., Kim, Y., Song, L. H., Coutu, J., Coutu, A., Ciftci-Yilmaz, S., et al. (2006). Gain- and loss-of-function mutations in Zat10 enhance the tolerance of plants to ablotic stress. Febs Lett. 580, 6537–6542. doi: 10.1016/j.febslet.2006.11.002
Morel, M., Crouzet, J., Gravot, A., Auroy, P., Leonhardt, N., Vavasseur, A., et al. (2009). AtHMA3, a P-1B-ATPase Allowing Cd/Zn/Co/Pb Vacuolar Storage in Arabidopsis. Plant Physiol. 149, 894–904. doi: 10.1104/pp.108.130294
Opdenakker, K., Remans, T., Keunen, E., Vangronsveld, J., and Cuypers, A. (2012). Exposure of Arabidopsis thaliana to Cd or Cu excess leads to oxidative stress mediated alterations in MAPKinase transcript levels. Environ. Exp. Bot. 83, 53–61. doi: 10.1016/j.envexpbot.2012.04.003
Palansooriya, K. N., Shaheen, S. M., Chen, S. S., Tsang, D. C. W., Hashimoto, Y., Hou, D. Y., et al. (2020). Soil amendments for immobilization of potentially toxic elements in contaminated soils: A critical review. Environ. Int. 134:105046. doi: 10.1016/j.envint.2019.105046
Pence, N. S., Larsen, P. B., Ebbs, S. D., Letham, D., Lasat, M. M., Garvin, D. F., et al. (2000). The molecular physiology of heavy metal transport in the Zn/Cd hyperaccumulator Thlaspi caerulescens. Proc. Natl. Acad. Sci. 97, 4956–4960. doi: 10.1073/pnas.97.9.4956
Perez-Chaca, M. V., Rodriguez-Serrano, M., Molina, A. S., Pedranzani, H. E., Zirulnik, F., Sandalio, L. M., et al. (2014). Cadmium induces two waves of reactive oxygen species in Glycine max (L.) roots. Plant Cell Environ. 37, 1672–1687. doi: 10.1111/pce.12280
Rogers, E. E., Eide, D. J., and Guerinot, M. L. (2000). Altered selectivity in an Arabidopsis metal transporter. Proc. Natl. Acad. Sci. U.S.A. 97, 12356–12360. doi: 10.1073/pnas.210214197
Schaaf, G., Honsbein, A., Meda, A. R., Kirchner, S., Wipf, D., and von Wiren, N. (2006). AtIREG2 encodes a tonoplast transport protein involved in iron-dependent nickel detoxification in Arabidopsis thaliana roots. J. Biol. Chem. 281, 25532–25540. doi: 10.1074/jbc.M601062200
Shi, H. T., Wang, X., Ye, T. T., Chen, F. F., Deng, J., Yang, P. F., et al. (2014). The Cysteine2/Histidine2-Type Transcription Factor ZINC FINGER OF ARABIDOPSIS THALIANA6 Modulates Biotic and Abiotic Stress Responses by Activating Salicylic Acid-Related Genes and C-REPEAT-BINDING FACTOR Genes in Arabidopsis. Plant Physiol. 165, 1367–1379. doi: 10.1104/pp.114.242404
Shi, H. T., Ye, T. T., Chen, F. F., Cheng, Z. M., Wang, Y. P., Yang, P. F., et al. (2013). Manipulation of arginase expression modulates abiotic stress tolerance in Arabidopsis: Effect on arginine metabolism and ROS accumulation. J. Exper. Bot. 64, 1367–1379. doi: 10.1093/jxb/ers400
Spielmann, J., Ahmadi, H., Scheepers, M., Weber, M., Nitsche, S., Carnol, M., et al. (2020). The two copies of the zinc and cadmium ZIP6 transporter of Arabidopsis halleri have distinct effects on cadmium tolerance. Plant Cell Environ. 43, 2143–2157. doi: 10.1111/pce.13806
Tran, T. A., and Popova, L. P. (2013). Functions and toxicity of cadmium in plants: Recent advances and future prospects. Turkish J. Bot. 37:154. doi: 10.3906/bot-1112-16
Verbruggen, N., Hermans, C., and Schat, H. (2009). Mechanisms to cope with arsenic or cadmium excess in plants. Curr. Opin. Plant Biol. 12, 364–372. doi: 10.1016/j.pbi.2009.05.001
Vert, G., Barberon, M., Zelazny, E., Seguela, M., Briat, J. F., and Curie, C. (2009). Arabidopsis IRT2 cooperates with the high-affinity iron uptake system to maintain iron homeostasis in root epidermal cells. Planta 229, 1171–1179. doi: 10.1007/s00425-009-0904-8
Vert, G., Grotz, N., Dedaldechamp, F., Gaymard, F., Guerinot, M. L., Briat, J. F., et al. (2002). IRT1, an Arabidopsis transporter essential for iron uptake from the soil and for plant growth. Plant Cell 14, 1223–1233. doi: 10.1105/tpc.001388
Wang, H. Y., Klatte, M., Jakoby, M., Baumlein, H., Weisshaar, B., and Bauer, P. (2007). Iron deficiency-mediated stress regulation of four subgroup Ib BHLH genes in Arabidopsis thaliana. Planta 226, 897–908. doi: 10.1007/s00425-007-0535-x
Wang, N., Cui, Y., Liu, Y., Fan, H. J., Du, J., Huang, Z. A., et al. (2013). Requirement and Functional Redundancy of Ib Subgroup bHLH Proteins for Iron Deficiency Responses and Uptake in Arabidopsis thaliana. Mol. Plant 6, 503–513. doi: 10.1093/mp/sss089
Wang, P., Chen, H., Kopittke, M. P., and Zhao, F. (2019). Cadmium contamination in agricultural soils of China and the impact on food safety. Environ Pollut. 249, 1038–1048. doi: 10.1016/j.envpol.2019.03.063
Wu, H. L., Chen, C. L., Du, J., Liu, H., Cui, Y., Zhang, Y., et al. (2012). Co-Overexpression FIT with AtbHLH38 or AtbHLH39 in Arabidopsis-Enhanced Cadmium Tolerance via Increased Cadmium Sequestration in Roots and Improved Iron Homeostasis of Shoots. Plant Physiol. 158, 790–800. doi: 10.1104/pp.111.190983
Wu, H. L., and Ling, H. Q. (2019). FIT-Binding Proteins and Their Functions in the Regulation of Fe Homeostasis. Front. Plant Sci. 10:844. doi: 10.3389/fpls.2019.00844
Yao, X. N., Cai, Y. R., Yu, D. Q., and Liang, G. (2018). bHLH104 confers tolerance to cadmium stress in Arabidopsis thaliana. J. Integr. Plant Biol. 60, 691–702. doi: 10.1111/jipb.12658
Yoo, S. D., Cho, Y. H., and Sheen, J. (2007). Arabidopsis mesophyll protoplasts: A versatile cell system for transient gene expression analysis. Nat. Protoc. 2, 1565–1572. doi: 10.1038/nprot.2007.199
Yuan, Y. X., Wu, H. L., Wang, N., Li, J., Zhao, W. N., Du, J., et al. (2008). FIT interacts with AtbHLH38 and AtbHLH39 in regulating iron uptake gene expression for iron homeostasis in Arabidopsis. Cell Res. 18, 385–397. doi: 10.1038/cr.2008.26
Zheng, X., Chen, L., and Li, X. (2018). Arabidopsis and rice showed a distinct pattern in ZIPs genes expression profile in response to Cd stress. Bot. Stud. 59:22. doi: 10.1186/s40529-018-0238-6
Zhu, X. F., Jiang, T., Wang, Z. W., Lei, G. J., Shi, Y. Z., Li, G. X., et al. (2012). Gibberellic acid alleviates cadmium toxicity by reducing nitric oxide accumulation and expression of IRT1 in Arabidopsis thaliana. J. Hazard. Mater. 239, 302–307. doi: 10.1016/j.jhazmat.2012.08.077
Zhu, X. F., Wang, Z. W., Dong, F., Lei, G. J., Shi, Y. Z., Li, G. X., et al. (2013). Exogenous auxin alleviates cadmium toxicity in Arabidopsis thaliana by stimulating synthesis of hemicellulose 1 and increasing the cadmium fixation capacity of root cell walls. J. Hazard. Mater. 263, 398–403. doi: 10.1016/j.jhazmat.2013.09.018
Keywords: ZAT10, Cd stress, uptake, detoxification, FIT, Arabidopsis
Citation: Dang F, Li Y, Wang Y, Lin J, Du S and Liao X (2022) ZAT10 plays dual roles in cadmium uptake and detoxification in Arabidopsis. Front. Plant Sci. 13:994100. doi: 10.3389/fpls.2022.994100
Received: 14 July 2022; Accepted: 11 August 2022;
Published: 30 August 2022.
Edited by:
Iftikhar Ali, State Key Laboratory of Molecular Developmental Biology, Institute of Genetics and Developmental Biology (CAS), ChinaReviewed by:
Wei Yunxie, Hainan University, ChinaArtem Bonchuk, Institute of Gene Biology (RAS), Russia
Copyright © 2022 Dang, Li, Wang, Lin, Du and Liao. This is an open-access article distributed under the terms of the Creative Commons Attribution License (CC BY). The use, distribution or reproduction in other forums is permitted, provided the original author(s) and the copyright owner(s) are credited and that the original publication in this journal is cited, in accordance with accepted academic practice. No use, distribution or reproduction is permitted which does not comply with these terms.
*Correspondence: Xinyang Liao, x.liao.1@outlook.com
†These authors have contributed equally to this work