- 1Department of Plant Agriculture, University of Guelph, Guelph, ON, Canada
- 2Department of Plant Agriculture, University of Guelph, Ridgetown, ON, Canada
In flowering plants, fertilization requires exposing maternal style channels to the external environment to capture pollen and transmit its resident sperm nuclei to eggs. This results in progeny seed. However, environmental fungal pathogens invade developing seeds through the style. We hypothesized that prior to environmental exposure, style tissue already possesses bacteria that can protect styles and seed from such pathogens. We further hypothesized that farmers have been inadvertently selecting immature styles over many generations to have such bacteria. We tested these hypotheses in maize, a wind-pollinated crop, which has unusually long styles (silks) that are invaded by the economically-important fungal pathogen Fusarium graminearum (Fg). Here, unpollinated silk-associated bacteria were cultured from a wild teosinte ancestor of maize and diverse maize landraces selected by indigenous farmers across the Americas, grown in a common Canadian field for one season. The bacteria were taxonomically classified using 16S rRNA sequencing. In total, 201 bacteria were cultured, spanning 29 genera, 63 species, and 62 unique OTUs, dominated by Pseudomonas, Pantoea and Microbacterium. These bacteria were tested for their ability to suppress Fg in vitro which identified 10 strains belonging to 6 species: Rouxiella badensis, Pantoea ananatis, Pantoea dispersa, Pseudomonas koreensis, Rahnella aquatilis, and Ewingella americana. Two anti-Fg strains were sprayed onto silks before/after Fg inoculation, resulting in ≤90% reductions in disease (Gibberella ear rot) and 70-100% reductions in associated mycotoxins (deoxynivalenol and zearalenone) in progeny seeds. These strains also protected progeny seeds post-harvest. Confocal fluorescent imaging showed that one silk bacterium (Rouxiella AS112) colonized susceptible entry points of Fg on living silks including stigmatic trichomes, wounds, and epidermal surfaces where they formed thick biofilms. Post-infection, AS112 was associated with masses of dead Fg hyphae. These results suggest that the maize style (silk) is endowed with potent bacteria from the mother plant to protect itself and progeny from Fusarium. The evidence suggests this trait may have been selected by specific indigenous peoples, though this interpretation requires further study.
Introduction
In flowering plants, the fertilization process is initiated by maternal-style channels exposed to the external environment on which the pollen (male gametophyte) lands (Dresselhaus et al., 2011; Dresselhaus et al., 2016). The pollen then germinates on the stigma and elongates as pollen tubes encased within the style through which the sperm nuclei migrate to the ovule, resulting in fertilization and seed formation. However, the exposed style passage also acts as the entry point for environmental fungal pathogens that target seeds (Thompson and Raizada, 2018).
The maternal parent plays diverse roles in the reproduction process from guiding pollen tubes to ovules, to controlling seed development after fertilization (Yadegari and Drews, 2004; Drews and Koltunow, 2011). Similar to placental mammals (Chettoor et al., 2016), in flowering plants, an embryo develops inside the surrounding tissue of the mother, and its well-being depends on the protection and nurture provided by the maternal tissue, including against pathogens (Robert et al., 2018; Nguyen et al., 2021). Quantitative trait loci (QTLs) have been identified that improve style resistance to disease (Reid et al., 1996a; Reid et al., 1996b; Akohoue and Miedaner, 2022), suggestive that the maternal parent may protect the stigma-style passage, but little appears to be known about the underlying mechanism(s).
The style and stigma tissues have microbiomes. These microbiomes have only been explored in a few plants, including pear stigma (Stockwell et al.,1999), styles of the wild tree Metrosideros polymorpha (Junker and Keller, 2015), stigma and styles of the yellow monkeyflower (Rebolleda Gómez and Ashman, 2019), apple stigma (Cui et al., 2021), and maize styles with stigmatic hairs (Khalaf et al., 2021; Diniz et al., 2022a). In pear and apple, epiphytic bacteria from stigmata surfaces were reported to reduce the severity of fire blight disease caused by the bacterial pathogen Erwinia amylovora (Wilson and Lindow, 1992; Wilson et al., 1992; Johnson et al., 1993; Kearns, 1993; Lindow et al., 1996; Nuclo et al., 1998; Stockwell et al., 1999; Cui et al., 2021), suggesting that the microbiome of the maternal parent may contribute to its ability to protect the male migration route from environmental pathogens. However, to the best of our knowledge, in these studies, the flowers were open and hence the stigma/style was exposed to the environment, making it unclear if the microbes originated from the air and/or insects, or pollen/pollen tubes since pollen have rich, diverse microbiomes in comparison to other phyllosphere plant tissues (Obersteiner et al., 2016; Zasloff, 2017; Manirajan et al., 2018; Oteros et al., 2018; McFrederick and Rehan, 2019), or alternatively whether unpollinated styles have their own defensive microbiome of maternal origin.
Maize (Zea mays L.) is a wind-pollinated plant, requiring environmental exposure of the style to capture airborne pollen. These style channels are known as silks, the threads that extend outside of husk leaves at the tips of corn cobs, with each silk terminating in an ovule (Kroh et al., 1979; Heslop-Harrison et al., 1985). The environmental fungal pathogen, Fusarium graminearum (Fg) Schwabe, invades and grows through the silks at the time of pollination, resulting in a seed disease called Gibberella ear rot (GER) (Reid et al., 1992). Maize silks are also targets of foraging insects (Hazzard et al., 2003), which create entry (wound) sites for fungal pathogens including Fg. Fg and associated species then synthesize several mycotoxins, including deoxynivalenol (DON) and zearalenone (ZEA), which affect human and livestock health after being consumed, causing major economic losses to maize farmers globally (Miller et al., 2007; Thompson and Raizada, 2018). DON also suppresses plant defense responses (McCormick et al., 2011), thereby promoting Fg infection (Mudge et al., 2006). Critically, Fusarium species are also known to be present in pollen, and DON and ZEA have been shown to suppress pollen tube growth (Tejaswini, 2002; Kačániová et al., 2011; Kostić et al., 2019) which would prevent fertilization. Therefore, the silk-invading pathogen Fg and its mycotoxins reduce the fitness of future progeny and ultimately the genetic contribution of the female gametes. The long length, abundance, and accessibility of maize silks, combined with the economic importance of Fg, make it a model system for style-pathogen interactions.
A previous study from our group showed that maize silks have a complex microbiome: silks from a North American maize diversity panel were shown to host >1300 bacterial genera when combined (Khalaf et al., 2021). The study also demonstrated that some silk-associated bacterial taxa increased in relative abundance after silk Fg infection but they were not cultured and hence could not be tested functionally. One challenge is that the study employed short-length 16S rRNA sequencing (V4 region) (Khalaf et al., 2021) which has limited taxonomic resolution, and hence silk-associated bacterial species that may have anti-Fg activity could not be identified at the species level. Furthermore, the study focused on open-pollinated maize silks, and hence as already noted, the maternal contribution of these microbes was ambiguous.
Another recent study involving modern Brazilian maize hybrids (unidentified) reported dominant silk-associated bacteria belonging to the genera Bacillus, Burkholderia, Achromobacter, Pseudomonas, and Serratia (Diniz et al., 2022a). The silk-derived bacteria were shown to be antagonistic to the maize stem rot pathogen Fusarium verticillioides both in vitro and in greenhouse trials, also reducing fungal growth when applied to stored grains, but their activity on silks was not tested (Diniz et al., 2022b). Furthermore, in the Brazilian study, as in the above studies, the microbes were isolated from open-pollinated silks, making their parental origin unknown. In maize, the microbiota of unpollinated silks has not been reported to the best of our knowledge. However, endophytic microbes inhabiting other tissues in maize and its relatives (e.g. seeds, roots) have been shown to combat Fg when sprayed onto silks (Mousa et al., 2015).
The ability of the maternally derived microbiome to defend the male gamete migration route against environmental pathogens remains unclear. Any study must screen microbes from stigma-styles/silks that are not exposed to pollen (the male microbiome), pollinators, and/or the ambient environment, and critically, must show direct evidence that these microbes can protect stigma-style tissues (e.g. using microscopy). If this maternal ability exists, then there should be evidence that farmers have been able to select for such microbes in their crops.
Here, we hypothesized that in plants, the maternal parent can pro-actively protect the male gamete migration route (stigma-style channel) from environmental pathogens. Specifically, in maize, we hypothesized that unpollinated silks possess bacteria that can protect silks from Fg at the time of pollination. Furthermore, we hypothesized that indigenous farmers across the Americas have inadvertently selected immature silks to have such bacteria, either by selecting seed for re-planting that are symptomless (healthy) and/or which belonged to seed batches that did not make humans or livestock sick because they had low concentrations of mycotoxins – though the latter would be less likely.
To test these hypotheses, we cultured bacteria from unpollinated maize silks, protected from environmental exposure by husk leaves and ear bags, originating from maize landraces selected by indigenous peoples from across the Americas. Unpollinated silks were collected from plants grown in a common field in Canada for one season. The bacteria were taxonomically classified using full-length 16S rRNA sequencing, tested for anti-Fg activity in vitro, and then sprayed onto silks prior to deliberate Fg exposure followed by progeny seed disease/mycotoxin analysis, to verify their biological relevance. A further trial tested whether the silk-derived bacteria could protect progeny seeds from Fg even after they had dispersed from the mother plant. Finally, we used confocal fluorescence imaging of living silks for direct evidence of whether silk-derived bacteria could defend silks against Fg.
Maize landraces as sources of silk-derived microbes were selected that spanned diverse geographies, cultivation timespans, and indigenous peoples (farmers) from across the Americas. Maize was domesticated about 9000 years ago in southwestern Mexico from wild teosintes including Zea mays ssp. mexicana, the minor teosinte ancestor of modern maize, which has contributed 12% of modern maize alleles (Matsuoka et al., 2002). After being domesticated, ancient maize was migrated across the Americas by distinct indigenous groups (Figure 1; Table 1) (Matsuoka et al., 2002; Warburton et al., 2011; Prasanna, 2012; Bedoya et al., 2017). In each new environment, farmers independently selected maize landraces to adapt to the local environment and needs (Ruiz Corral et al., 2008; Vigouroux et al., 2008; Langner et al., 2019).
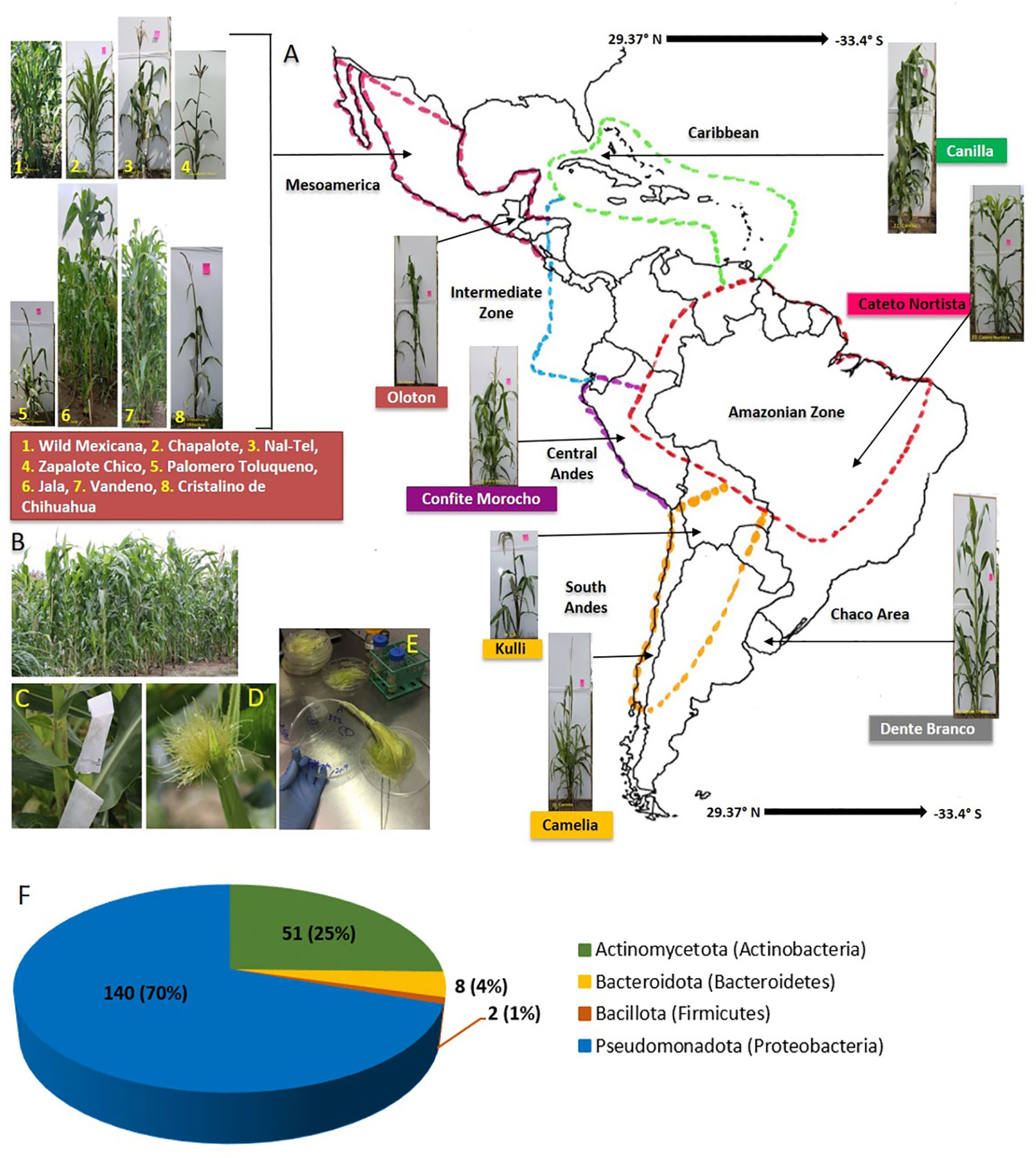
Figure 1 Origin of maize accessions used in this study to culture unpollinated silk-associated bacteria and their phylum level taxonomic distribution. Plants were grown in a common field in Canada. (A) A map of Latin America and the Caribbean showing the origin of the fifteen maize accessions used in this study. (B) Picture of the common maize field taken at the flowering stage, at Elora, Ontario, Canada. (C) Example of a maize ear covered within an ear bag to avoid silk exposure to the environment. (D) Example of silks ready for harvesting wherein silks had emerged inside the ear bags. (E) Processing of silk samples in the laboratory under sterile conditions prior to freezer storage in glycerol. (F) Phylum-level taxonomic distribution of the 201 bacterial isolates cultured in this study; the numbers on the left are the absolute number of isolates belonging to each phylum, while the numbers in brackets indicate their relative percentage in the silk-associated bacterial library.
Near the center of maize diversification in south-central Mexico, ancient indigenous peoples selected the highland Mexican popcorn landrace, Palomero Toluqueno (Rocío Aguilar-Rangel, 2018); it is reported to be resistant to drought and pests (Arnason et al., 1994), and is a progenitor of many productive modern highland landraces (Perez-Limó et al., 2022).
From its center of diversification, maize was then migrated north and south. Moving northwards, the ancient landrace Jala became known for its giant plant height (up to 5 m tall); it bears the longest cobs in the world and hence extremely long silks (Rice, 2007). Also in northern Mexico, the primitive lowland popcorn landrace Chapalote became highly valued by traditional farmers and has been continuously grown for 3000 years (Bonavia, 2013); Chapalote is thought to be the first maize to enter the United States more than 2000 years ago (Da Fonseca et al., 2015). Cristalino de Chihuahua, a more recent northern Mexican landrace, was adapted to very dry conditions (Ruiz Corral et al., 2008).
On the southern migration route of maize, the ancient Mayans of the Yucatan Peninsula in southern Mexico grew the landrace Nal-Tel, a distinctive primitive popcorn (Turrent and Serratos, 2004) that was critical to their diet (Santillán-Fernández et al., 2021). Similarly, Zapalote Chico was adapted to southern Mexican/Central American environments and later contributed to food security across Latin America (Taba et al., 1995). Also in this region, Vandeno became an agronomically important landrace that was the progenitor of many modern maize varieties (Bedoya et al., 2017) and has been extensively used in breeding programs (Taba, 1995); it is resistant to aflatoxin produced by the fungus, Aspergillus flavus (Ortega-Beltran et al., 2014). In Guatemala, indigenous peoples selected the highland landrace Oloton which was later introduced to Mexico (Wellhausen, 1952), becoming a staple food for the Mixe indigenous people in the Sierra Mixe region of Oaxaca in southern Mexico (Pskowski, 2019); it is highly resistant to pests and pathogens (CONABIO, 2017) and has aerial roots that produce a mucous-like gel that fixes nitrogen (Pskowski, 2019). In South America, Confite Morocho from Peru became the primitive ancestor of Andean popcorn (Goodman and Brown, 1988), known for its short stature and small ears, and hence short silks (Grobman et al., 1961). Kulli became a Bolivian highland maize landrace (Cuevas Montilla et al., 2011), popular for making fermented beer (Ricardo et al., 1960). Cateto Nortista, a tropical flint, was selected as late-flowering maize in coastal Brazil (Bedoya et al., 2017), while Camelia was selected in lowland Chile to be resistant to Fusarium ear rot (USDA-ARS, 2018). Dente Branco was reportedly migrated from the United States to lowland Uruguay and possesses resistance to Fusarium ear rot (USDA-ARS, 2018). Maize was also migrated to the Caribbean, where the landrace Canilla was adapted to the lowland region of Cuba (Hatheway, 1957).
Here we provide multiple lines of evidence in maize, including from direct microscopy, to demonstrate that while sexual reproduction necessitates that silks become exposed to the environmental pathogen Fg to enable capture and transmission of male gametes, some maternally derived silks possess bacteria that can protect silks and progeny seed from Fg. Furthermore, we provide preliminary evidence that suggests that distinct indigenous peoples in the Americas have selected for such defensive bacteria, but further confirmation is required. Since there are no prior studies on bacteria isolated from unpollinated maize silks, we also report preliminary qualitative observations on taxonomic patterns within the culturable maize silk microbiome across maize accessions, with the limitation that the bacteria were cultured from plants grown in a common field and a single season.
Materials and methods
Maize accession selection, growth conditions, and experimental design
A diverse panel of fifteen maize accessions including wild maize and ancient maize landraces from the Americas were selected for the study (Figure 1); the detailed information of which is presented in Tables 1 and S1. Seeds of 2 maize accessions (Chapalote and Zea mays ssp. mexicana) were received from the U.S. Department of Agriculture (Germplasm Resources Information Network, GRIN), and the remaining 13 from the Maize Germplasm Bank of the International Maize and Wheat Improvement Center (CIMMYT), Mexico. These maize accessions required a short day length between V5 to V8 growth stages to subsequently induce flowering in Ontario’s long-day field conditions since they were short-day adapted maize from the tropical regions, and were sensitive to photoperiod. To allow this, the seeds were first sown in 5X5 inch biodegradable pots filled with a mixture of Sunshine Mix (LA4, Sungrow®Horticulture, Brantford, Ontario, Canada) and field soil from the Elora Research Station, Elora, Ontario, Canada. Field soil was added to provide a source of microbes since some endophytes are known to be taken up through the roots. The pots were arranged in trays by maize accession and were grown in a growth room under a controlled environment in the University of Guelph Crop Science growth room facility with a 14-hour photoperiod until the seedlings reached the V5 growth stage. Then, the photoperiod was reduced to 10 hours until the V8 growth stage. Manual irrigation as well as fertilization with 20:20:20 plus micronutrients (Plant-Prod 20-20-20 Classic, Product Number: 10529, Brampton, Ontario, Canada) was done. The photoperiod for the plants was supplied with fluorescent light (LED 18 ET9/4/850 bulbs, GE), supplemented with LED 9.5 A19/DIM/0/827/G4 1100 Lumen 2700K bulbs (OSRAM, Canada). To supply equal light distribution to the plants, the trays were rotated twice per week, where the light intensity was 425-515 μmol m-2 s-1 at pot level. At the V8 growth stage, a total of 780 large corn seedlings were loaded in a trailer and transported to the field at Elora Research Station (latitude: 43°41’ 3.59” N; longitude: -80° 25’ 22.79” W). The seedlings were acclimatized to the field environment by keeping them under direct sunlight for 2-3 hours/day and left under the shade for the remaining time. This was important to avoid sunburn problems and seedling death since they originated from a controlled environment inside the growth room. After 3 days, the seedlings were transplanted to the field on the 10th of July, 2019, in a randomized block design with five replicate blocks, each having six plants. The field was pre-applied with 160 kg/ha of N, 60 kg/ha of P2O5, 80 kg/ha of K2O, 10 kg/ha of S fertilizers and herbicides (4.0 l/ha of Primextra, and 0.3 l/ha of Callisto). Irrigation of the seedlings was done manually for one week after transplantation.
Silk harvesting, culturing and taxonomic analyses
Silk harvesting was undertaken from the 3rd week of August to the 2nd week of October 2019. The ears were bagged before the silks emerged; they were allowed to develop and then harvested at the first emergence. The harvested ears were brought back to the lab with the ear bags on, then under sterilized conditions, ~ 3 cm at the cob tips were cut and removed, the remaining cob was de-husked, and their silks were dissected and kept for culturing. Each silk sample was pooled from all five field blocks, with 3-5 ears per block. Thereafter, 40% sterile glycerol was added to the pooled samples which were then stored at -80°C. The frozen silk samples were ground and the lysates were used to culture microbes on Reasoner’s 2A (R2A) (R2A powder 18.12 g/L – containing 0.5 g yeast extract, 0.5 g proteose peptone, 0.5 g casein hydrolysate, 0.5 g glucose, 0.5 g starch, 0.3 g di-potassium phosphate, 0.024 g magnesium sulphate, 0.3 g sodium pyruvate, and 15 g agar, per liter, adjusted to pH 7.2 with NaOH and autoclaved) and LB media (Luria-Bertani, composed of 10 g NaCl, 5 g yeast extract, and 10 g tryptone, per liter, adjusted to pH 7.2), followed by 16S rRNA based taxonomic profiling using 27F and 1492R primers, and phylogenetic tree construction using MEGA-X software. For details of culturing, glycerol stock preparation, taxonomic analysis, OTU assignment and phylogenetic tree construction, see Supplemental Methods.
In vitro assays for anti-Fusarium screening
Dual culture assays were undertaken to test silk-associated bacteria in vitro for anti-Fg activity, by modifying an earlier method (Mousa et al., 2015). See Supplemental Methods for details. For analyzing the results of these assays, the diameter of each Fg-inhibition zone was statistically modeled with a generalized linear mixed model (GLMM) using PROC GLIMMIX, then analyzed using One-Way ANOVA, and compared the means using Tukey’s pairwise comparison in SAS 9.4 (SAS Institute, Cary, NC) with a significance level of P ≤ 0.05. However, while reporting here, each silk-associated anti-Fg bacteria was compared only with the negative control (LB Control) and the positive control (Proline, the commercial fungicide) treatments.
Greenhouse trials to test the ability of silk-associated bacterial strains to suppress Gibberella ear rot disease
Microbial treatment selection: antibiotic susceptibility testing
Candidate anti-Fg bacteria were tested for susceptibility to 20 clinical antibiotics to assure human safety, and only the safest strains proceeded to greenhouse trials (see Supplemental Methods for details).
Plant growth conditions and experimental design
Seeds (surface-sterilized) belonging to a moderately susceptible commercial maize hybrid DKC55-05RIB (Bayer Crop Science, Canada) were grown in the University of Guelph Crop Science Greenhouse Facility, starting May 25, 2021 (Trial 1) and June 11, 2021 (Trial 2) as described (See Supplemental Methods for details). In total, there were 168 maize pots organized as a randomized block design. There were seven treatments (two silk-associated bacteria, a negative and positive control, along with three non-silk-associated bacteria to be reported in a separate manuscript). The treatments were arranged into 6 blocks, each having 4 treatment replicates. One replicate was defined as one plant in one pot. A second completely independent trial was initiated two weeks after the first trial in a separate greenhouse. Only the upper (primary) ear from each plant was pollinated, treated, and used for disease scoring.
Bacterial and control treatments
Each bacterial strain and the negative control treatment were sprayed onto silks twice to ensure a high titer. The first spray was done at 48 h after pollination followed by waiting for 72 h to ensure effective colonization of bacteria. The bacterial strains were grown in LB broth (pH 7.2) for 48 h at 30°C with shaking at 200 rpm, then centrifuged for 10 min, resuspended to OD600 of 0.4-0.6 and then 1 mL was sprayed onto each ear using spray bottles. To avoid any cross-contamination of the sprays, the air coolers in each greenhouse zone were turned off each day during and after spraying (from 4:30 pm to 6:00 am). The second bacterial inoculation (1 mL/ear) was done 48 h following the pathogen application and prepared as already noted. The negative control included spraying with 1 mL of LB liquid (pH 7.2) (as a buffer control) similar to the bacterial treatment mentioned above. The positive control was a commercial fungicide, Prothioconazole (PROLINE 480 SC, Bayer Crop Science, Canada), mixed with 1.125% V/V of the surfactant Agral 90 (registration #11809, Syngenta Canada, Guelph, ON), and sprayed only once (1 mL/ear) onto the silks at 48 h after pollination, similar to the other bacterial treatments.
Pathogen (Fg) treatment
The Fg spore broth was prepared as follows: 0.35 g KNO3, 0.35 g KH2PO4, 0.175 g MgSO4, 0.175 g KCL, and 0.175 g dextrose were added to 175 mL of distilled water in a 1 L Erlenmeyer flask. Then, 175 µL micronutrient solution (20 mg/100 mL of each minor element: FeCl2, MnSO4, ZnSO4) was added to this flask and sterilized at 121°C for 20 min. Two PDA plugs of Fg isolate (the same isolate used for the dual culture assays) were added to the flask once the spore broth cooled down, covered with aluminum foil, and incubated in a dark incubator at 25°C with shaking at 120 rpm for 2 weeks. This spore broth was first filtered with cheesecloth and stored in the fridge, which was then standardized to 20,000 spores/mL with a haemocytometer on the day of pathogen inoculation. One mL of this standardized solution was sprayed onto the silks of any ears that were marked as pollinated, 72 h after the first bacterial treatment. The ears were then covered and tied up with white plastic bags to preserve humidity. These plastic bags were removed after 10 days, and water misting was done every afternoon for the next two weeks to keep each treated ear moist. Automated misting was set in the greenhouse zone (2 min/h) to keep the zone humid.
Harvesting of ears
Once the ears had matured, watering was ceased, approximately 45 days after the last pollination/crosses, and ears were let to dry for an additional week before harvesting. Criteria such as hardening of the cobs, size of the kernels, and the color of the cobs (deep yellow/orange) were checked to verify the maturity of the cobs. All the treated primary ears were harvested with husks on, packed in labeled bags with their pot identification number, and stored in cardboard boxes inside the greenhouse zone before their disease assessments.
Visual disease scoring of cobs
The disease scoring was done by visually phenotyping each harvested cob for the percentage of apparent infection, which was scored as the length of the disease area from the infection-starting site on the cob (cob tip) relative to the total length of each respective cob. The husks of the ears were removed before scoring. The measurements were taken from four different sides of each cob, and then the average of the infected portion was calculated relative to the total cob length. The cobs were then shelled and grain weight was taken from all the harvested cobs. All the scoring was done by the researcher blindly by selecting the bags with ears randomly. Once the scoring was complete for both greenhouse zones, then the results were organized by treatment and block based on the greenhouse treatment map. The percent cob-infected data was statistically modeled using PROC GLIMMIX in GLMM, analyzed using a beta distribution, and compared using Tukey’s pairwise comparison. The average grain weight data were analyzed using One-Way ANOVA and compared using Tukey’s pairwise comparison, both in SAS version 9.4 (SAS Institute, Cary, NC) with a significance level of P ≤ 0.05.
Visual assessment of kernel disease severity
In addition to visual disease scoring of cobs, a visual disease severity assessment of kernels from each cob was performed. Kernel disease scoring was done blindly by a co-author (D.B.), by visualizing one side/angle of the cob, i.e., half the side of each cob using pictures of cobs. The scoring was based on a 1-4 scale, with 4 being the most infected kernel (infected from all sides); 3 described as a medium infected kernel [(limited damage to the kernel on the top portion + damage on the sides of the kernel (e.g., covered with mycelia)]; 2 as a less infected kernel (healthy kernel on the top portion + damage on the sides of the kernel, or limited damage on the top portion + no damage on the sides of the kernel); and 1 noted as no kernel damage + not covered with Fg-mycelium. The individual kernel disease severity percentage data was statistically modeled with a GLMM using PROC GLIMMIX, and analyzed using One-Way ANOVA where the percentage means were compared using Tukey’s pairwise comparisons in SAS version 9.4 (SAS Institute, Cary, NC) with a significance level of P ≤ 0.05.
Quantification of deoxynivalenol and other associated mycotoxins
After the grain weights were taken, the seeds were pooled from all four cobs of each treatment per block, mixed, and then one-third of the mixture was sent for multi-toxin analyses. In total, there were 84 samples (6 replicate samples per treatment, 7 treatments/zone, and 2 greenhouse zones). Analyses of DON and other associated mycotoxins were conducted using liquid chromatography-tandem mass spectrometry (LC-MS/MS) method following a previously reported protocol (Limay-Rios and Schaafsma, 2021). The data were statistically modeled with a GLMM using PROC GLIMMIX, then analyzed using One-Way ANOVA Dunnett’s comparisons in SAS version 9.4 (SAS Institute, Cary, NC) with a significance level of (P ≤ 0.05).
Light microscopy to visualize in vitro interactions between Fg pathogen and silk-associated bacterial strains
Light microscopy was used to study the in vitro interactions between Fg and the bacterial strains. For this, microscope slides were coated with a thin layer of 1 mL Potato Dextrose Agar (PDA) and allowed to solidify. 50 µL of Fg mycelia, grown for 72 h in Potato Dextrose Broth (PDB) at 25°C at 120 rpm, was applied in the center of the slide. On the adjacent side of this, 50 µL of each bacterial strain, cultured in LB liquid (pH 7.2) by incubating at 30°C at 200 rpm for 48 h, were applied. Likewise, LB broth was placed on the left side as a negative control. These slides were placed in closed Petri dishes and incubated at 25°C for 24 h. The positive control included Proline fungicide (1:10 v/v with ddH2O). The slides were then stained with the vitality stain, Evans blue (Catalog # E2129, Sigma Aldrich, Canada) by placing 1 mL of stain on the slide, which was then incubated for 5 min at room temperature, and washed 3-4 times with deionized water. Slides were prepared in triplicate for each treatment, visualized under a light microscope and pictures were captured.
Bacterial fluorescent tagging and confocal scanning fluorescence microscopy
Strain AS112 competent cells were transformed with plasmid pSW002-PpsbA-DsRed-Express2 then selected on LB agar (pH 7.2) with 5 mg/mL tetracycline, incubated at 30°C for 24 h and screened for fluorescence (see Supplemental Methods for details). Seeds belonging to modern maize inbreds (PHRE1, LH82) were surface disinfected, germinated in the lab for 7 days, followed by transplanting into pots filled with 100% Turface® clay at the University of Guelph Crop Science Greenhouse Facility. In the laboratory, a previous protocol from our lab was followed (Thompson and Raizada, 2023). Briefly, DsRed-tagged AS112 culture was inoculated onto silks at the tip of each cob; subsequently, 24 h later, GFP-tagged Fg [strain ZTE-2A (Miller et al., 2004)] was inoculated. To mimic damage from insects, silks were wounded. Cobs were placed in darkness for 48 h at 25°C, then the silks were stained with propidium iodide solution (1 mg/mL) (Catalog# P4864-10ML, Sigma-Aldrich, USA) and imaged using a confocal laser scanning microscope (model TCS SP5, Leica Microsystems, Mannheim, Germany) at the University of Guelph Molecular and Cellular Imaging Facility.
Testing anti-Fg bacterial strains for their ability to protect mature seed after harvest
This experiment was conducted as previously described (Mousa et al., 2016). The objective was to coat Fg-infected maize seeds with anti-Fg bacteria, dry and store the seeds at room temperature, and test for mycotoxin accumulation. For this experiment, deliberately Fg-infected maize seeds collected from the field with a moisture content of 17.5% were stored for 8 months, both in Falcon tubes and in envelopes to determine if the storage conditions affected the mycotoxin concentration. Ten grams of infected maize seeds were weighed per replicate per Falcon tube or sealed envelope. The anti-Fg bacteria were cultured in salt-reduced (5 g/L NaCl) LB broth (pH 7.2), then grown for 2 days at 30°C, with shaking at 200 rpm. The bacterial liquid cultures were then centrifuged for 10 min, followed by resuspension in LB broth to an optical density (OD600) of 0.4-0.6. One ml of this bacterial culture was applied to the infected maize seeds and mixed thoroughly. The negative control was seed coated with LB liquid (pH 7.2), whereas the positive control was a fungicide (PROLINE® 480 SC Foliar Fungicide, Bayer Crop Science) applied onto seeds, both at 1 mL per replicate. Proline was mixed with 0.125% v/v Agral 90 (registration #11809, Syngenta Canada, Guelph, ON).
There were 4 treatments in total: 2 anti-Fg bacterial treatments, a negative control (LB broth), and a positive control (Proline fungicide), with 3 replicates per treatment. After the inoculation step, the Falcon tubes and envelopes were kept in a secondary container and stored inside a cupboard in the lab at room temperature. ELISA analysis was performed to quantify DON levels in the maize seeds after 8 months of storage. For this, all seeds from each replicate were ground to a fine powder for one minute using a coffee grinder. Ten grams of these ground samples were diluted in 100 mL of deionized water followed by vigorously shaking for 3 min using a benchtop reciprocal Eberbach shaker equipped with a flask carrier (Eberbach, Corp. Ann Arbor, MI, USA). The sample extracts were allowed to sit for 3 min, allowing some particles to settle, and then 5 mL of each extract (aliquot) was filtered through a Neogen syringe filter, collecting a minimum of 3 mL into a sample collection tube. This filtrate was used for DON mycotoxin testing. Veratox for DON 5/5 (Lot #309312, NEOGEN Corporation, Lansing, USA), was used for ELISA analysis following the manufacturer’s protocol. The data were statistically modeled with a GLMM using PROC GLIMMIX and analyzed using One-Way ANOVA using Tukey’s multiple comparisons in SAS 9.4 with a significance threshold of P ≤ 0.05.
Results
Taxonomically diverse bacteria were cultured from unpollinated silks of diverse American maize accessions
From fifteen diverse American maize accessions including landraces and one wild teosinte (Mexicana, the minor ancestor of modern maize), all grown in a common field, a total of 201 unpollinated silk-associated bacterial isolates were cultured and taxonomically classified based on sequencing of the full-length 16S rRNA gene (Figures 1–3, S1; Table 1; Supplementary Table 1). The sequences were deposited in Genbank, and the accession numbers are reported (Supplementary Table 2). The isolates belonged to four phyla dominated by Pseudomonadota (Proteobacteria) with 140 isolates, followed by Actinomycetota (Actinobacteria) with 51 isolates, Bacteroidota (Bacteroidetes) with 8 isolates and Bacillota (Firmicutes) with 2 isolates (Figures 1–3). Within the phyla Actinobacteria and Firmicutes, all bacterial isolates belonged to only one class whereas, within Bacteroidetes and Proteobacteria, they belonged to more than one class. In total, the diverse American unpollinated silk-associated cultured library spanned 29 predicted bacterial genera, 63 predicted species, and 62 unique OTUs (Figures 2, 3; Supplementary Table 2). Of the bacterial genera, 19 belonged to Pseudomonadota with 42 OTUs, representing more than 65% of all unpollinated silk-associated OTUs (Figures 2A, B, 3). Another 6 belonged to phylum Actinomycetota with 12 unique OTUs, 2 belonged to Bacteroidota with 6 unique OTUs, and 2 belonged to Bacillota with 2 unique OTUs.
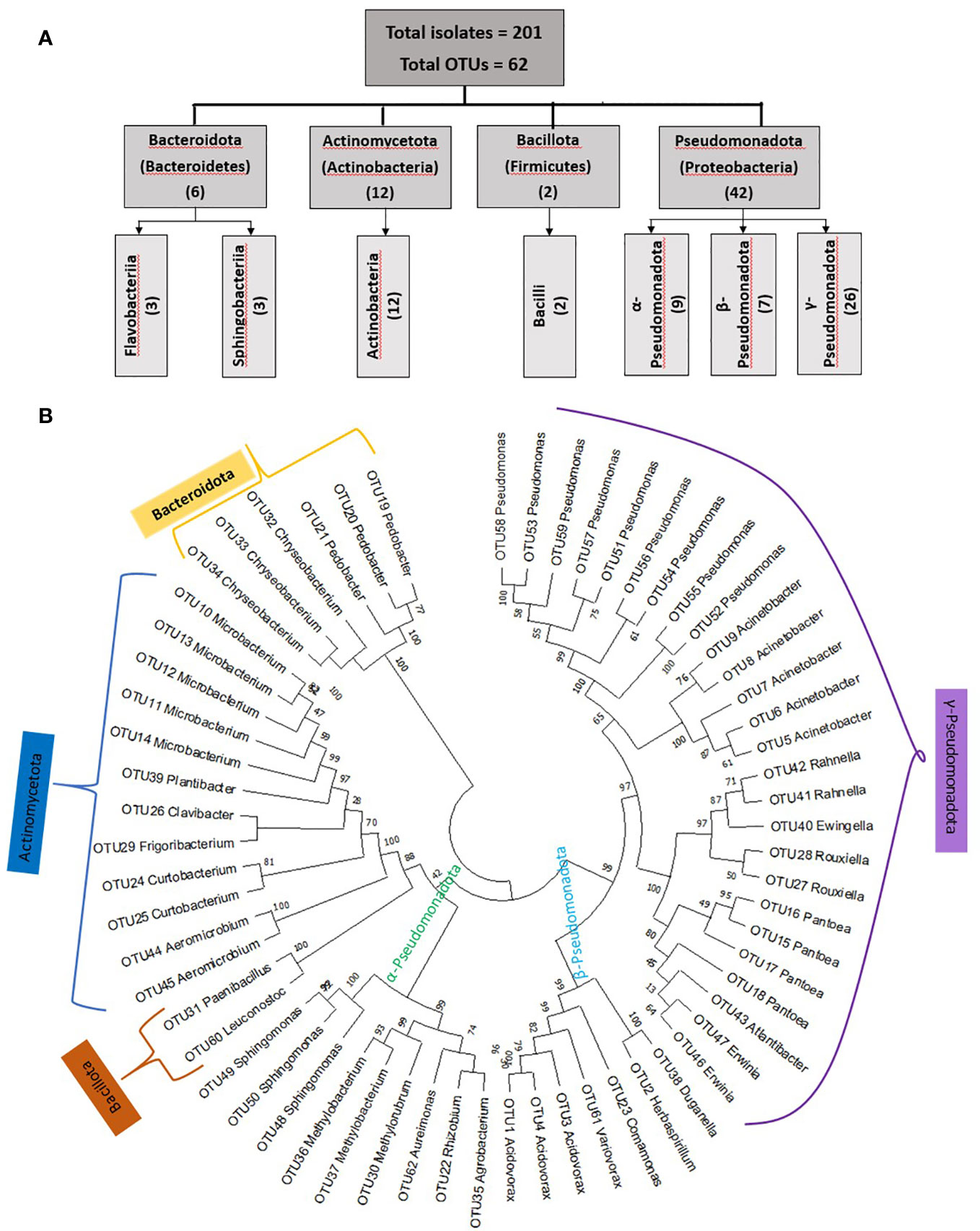
Figure 2 Summary of taxonomic classifications of unpollinated silk-associated bacteria cultured from diverse American maize accessions. (A) Diagrammatic sketch of the taxonomies at the phylum and class level based on full-length 16S RNA sequences. (B) Maximum likelihood (ML) phylogenetic tree of the entire bacterial population based on unique operational taxonomic units (OTUs). Bootstrap values are indicated above the branches.
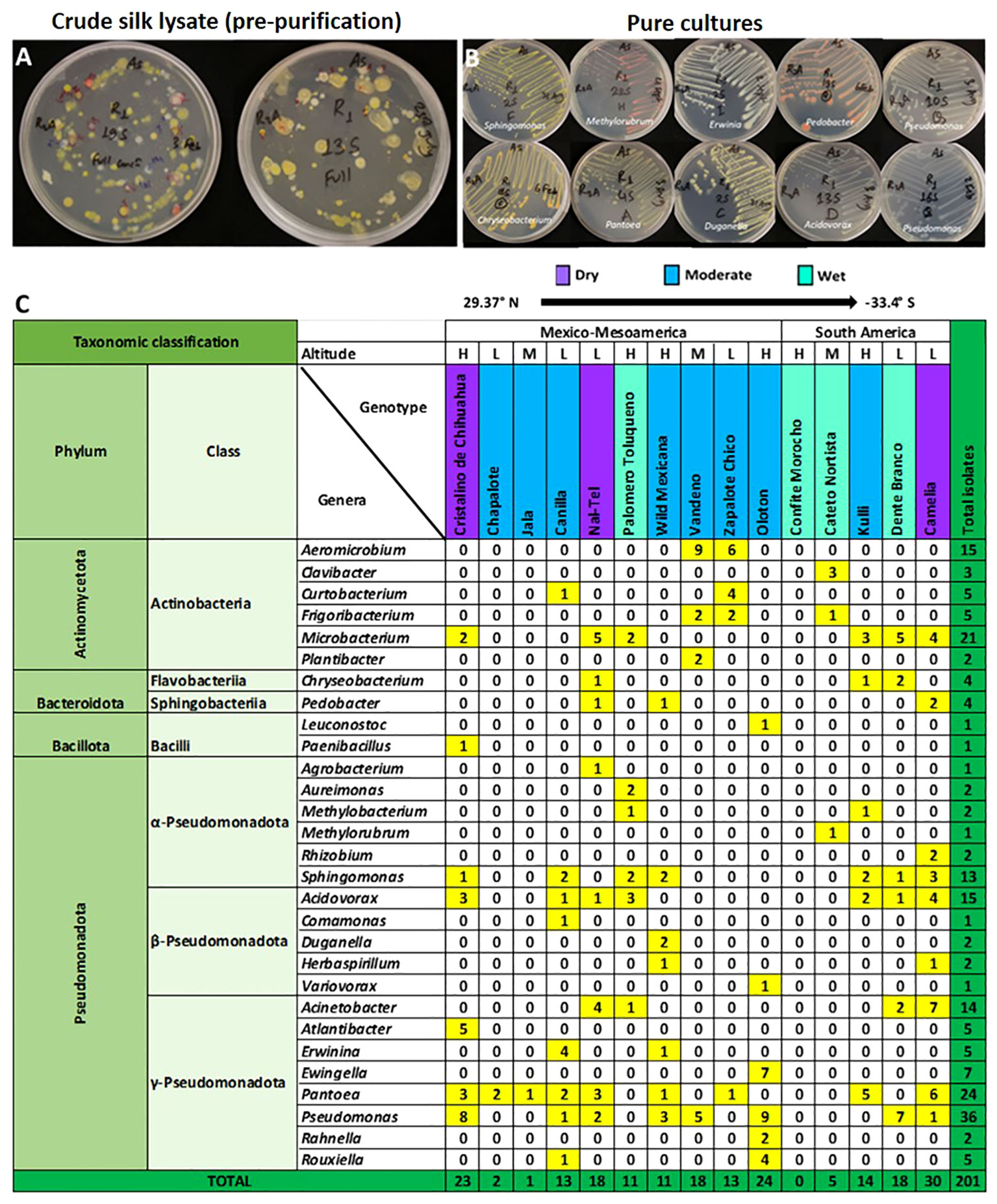
Figure 3 Summary of the taxonomies of unpollinated silk-associated bacteria at the phyla, class, and genus level. (A) Example photos of plates with crude silk lysate (pre-purification) after 5 days of incubation showing the presence of bacterial isolates with diverse colour, shape, and size. (B) Example photos of plates with pure cultures showing diverse bacterial genera after purification. (C) Phyla, class, and genera level summary of the cultured bacteria arranged by source host maize accession, arrayed by latitude from north to south. The predicted bacterial genera are based on full-length 16S Blastn searches at NCBI. The yellow and dark green colours highlight the total number of isolates (including redundant and unique sequences) belonging to the corresponding bacterial genus, arranged by host maize accession. The letter ‘H’ denotes an accession originating from a highland environment, ‘M’ denotes an accession originating from a mid-altitude environment, and ‘L’ denotes an accession originating from a lowland origin. The rainfall classification is shown in different colour codes (Dry, Moderate, and Wet). All plants were grown in a common field at Elora, Ontario, Canada.
Prevalence of culturable maize silk bacteria across maize accessions
We asked whether some unpollinated silk-associated bacteria were prevalent across maize accessions. Within phylum Pseudomonadota, the class Gammapseudomonadota was cultured from 13/15 host maize accessions studied, belonging to 8 different bacterial genera (Atlantibacter, Rahnella, Erwinia, Pantoea, Ewingella, Rouxiella, Acinetobacter, and Pseudomonas) (Figure 3). It is noteworthy that no bacterium was cultured from maize host Confite Morocho. Within phylum Actinomycetota, class Actinobacteria was isolated from 10/15 maize accessions, represented by six genera (Clavibacter, Curtobacterium, Frigoribacterium, Microbacterium, Plantibacter, and Aeromicrobium).
At the genus level, Pseudomonas was the most conserved in the unpollinated silk microbial library, accounting for 18% (36/201) of the total isolates, and was shared across 8/15 maize accessions (Figure 3). The second most conserved genus was Pantoea, accounting for 12% (24/201) of the total cultured isolates, and shared across 9/15 host accessions. Microbacterium was the third highest conserved genus, accounting for 10.4% (21/201) of the total cultured isolates and shared across 6/15 host accessions (Figure 3).
At the predicted species level, Pantoea agglomerans was the most prevalent, shared across 7/15 maize accessions, followed by Microbacterium testaceum (6/15 accessions), and Acidovorax wautersii (5/15 accessions) (Supplementary Table 2).
At the OTU level, 21 OTUs were cultured from more than one host maize accession (Figure 4). Of these shared OTUs, OTU1 (best match: Acidovorax wautersii), OTU10 (best match: Microbacterium testaceum), and OTU15 (best match: Pantoea agglomerans) were equally conserved and shared across 5/15 maize accessions (Figure 4).
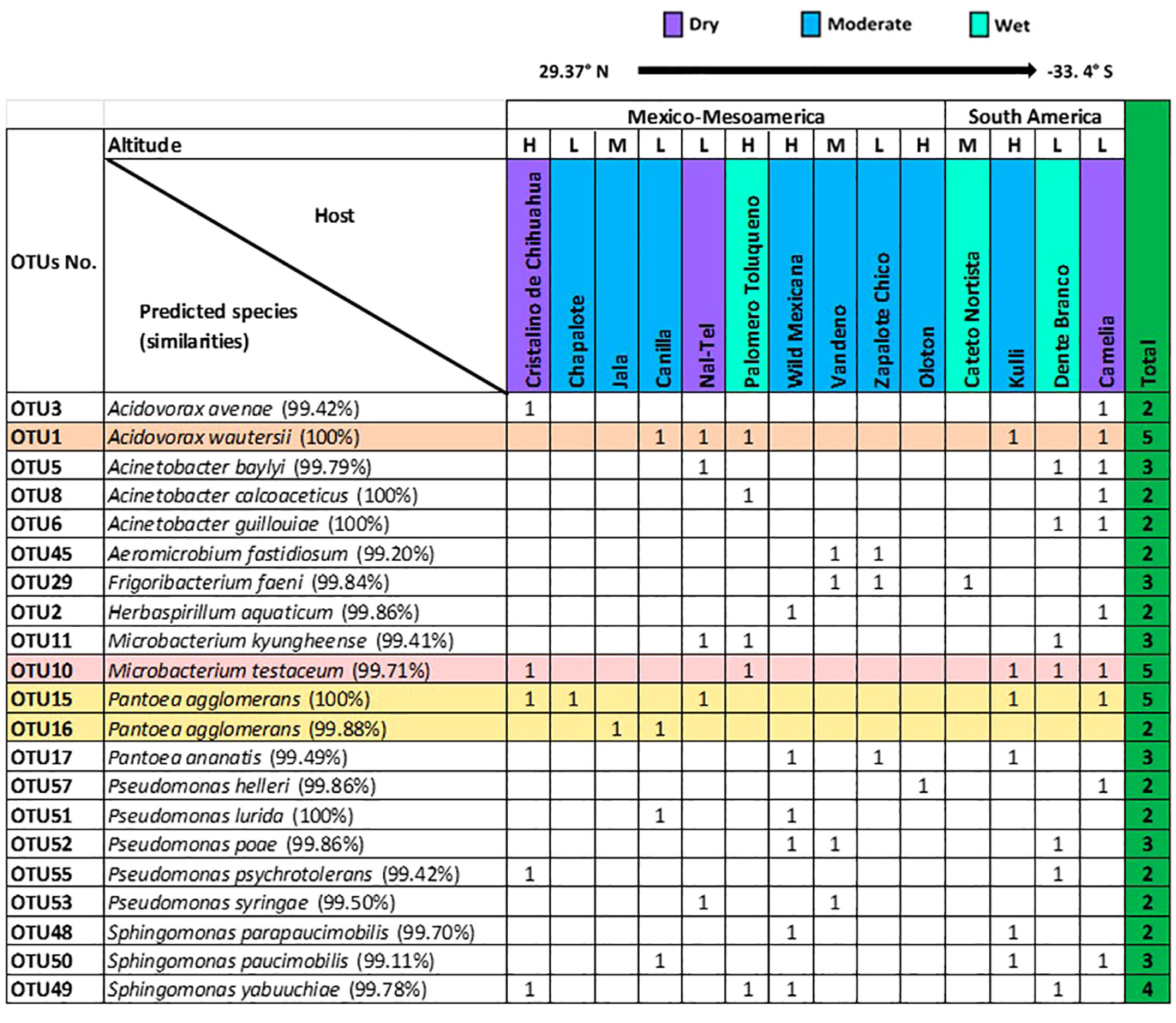
Figure 4 Summary of conserved OTUs of cultured unpollinated silk-associated bacteria across diverse American maize host accessions. Full-length 16S RNA sequences sharing the same genus were multi-aligned, and each unique sequence was assigned a distinct OTU number. Shown are the resulting species- and OTU-level taxonomic predictions of bacteria that were cultured from the unpollinated silks of more than one host maize accession. The most conserved OTUs are highlighted horizontally. The corresponding species prediction is noted along with the percentage identity to the top match in brackets. The data are organized by the latitude origin of source maize plants. The letter ‘H’ denotes a highland origin, ‘M’ denotes a mid-altitude origin, and ‘L’ denotes a lowland origin with respect to source maize plants. The rainfall classification is shown (Dry, Moderate, and Wet). All plants were grown in a common field in Elora, Ontario, Canada.
Unpollinated silk-associated bacteria have high intra-genus and intra-species diversity across diverse American maize accessions
Though some unpollinated silk-associated bacteria appeared to be highly conserved across diverse American maize accessions based on genus-level taxonomy (Figure 3), species/OTU level taxonomy revealed less conservation across host plants due to intra-genus level diversity. The 36 Pseudomonas isolates which appeared to be shared amongst 8 out of 15 maize accessions, actually encompassed 11 species (P. azotoformans, P. cannabina, P. cerasi, P. chlororaphis, P. helleri, P. koreensis, P. lurida, P. oryzihabitans, P. poae, P. psychrotolerans, and P. syringae) and 9 unique OTUs (OTU58, OTU53, OTU59, OTU57, OTU51, OTU56, OTU54, OTU55, and OTU52) (Supplementary Table S2; Figures 2, 3, S1). Of these, P. azotoformans, P. chlororaphis, P. helleri, and P. koreensis were only cultured from host Oloton, P. cannabina, and P. cerasi from host Vandeno, and P. oryzihabitans from host Camelia (Supplementary Table S2). At even greater taxonomic resolution within Pseudomonas, at the OTU level, OTU56 (best match: P. azotoformans/chlororaphis) and OTU54 (best match: P. koreensis) were only cultured from host Oloton, while OTU58 (best match: P. cannabina) and OTU59 (best match: P. cerasi) were only isolated from Vandeno (Supplementary Table S2). Similarly, the 24 isolates of Pantoea, apparently conserved amongst 9/15 maize accessions, spanned four diverse species and 4 unique OTUs. Of these, P. anthophila was only cultured from host Camelia, and P. dispersa from host Kulli. At the OTU level, OTU18 (best match: P. dispersa) was only cultured from Kulli. Similarly, the 21 isolates of Microbacterium, which also appeared conserved amongst 6/15 host accessions, included 3 species and 5 unique OTUs. Within this genus, M. neimengense was only cultured from host Nal-Tel. At higher resolution, OTU14 (best match: M. neimengense) was isolated from Nal-Tel, while OTU12 (best match: M. testaceum) was only cultured from host Dente Branco, and OTU13 (best match: M. testaceum) was only isolated from Nal-Tel (Supplementary Table S2; Figures 2, 3).
Maize host-specific silk microbiota
Amongst the maize host accessions, some of the silk-associated bacterial genera (14 in total) were uniquely cultured from a single maize accession (Figures 3, S2; Supplementary Table S2), though this could have occurred by random chance. For example, the genera Clavibacter and Methylorubrum were only cultured from landrace Cateto Nortista; Plantibacter from Vandeno; Paenibacillus and Atlantibacter from Cristalino de Chihuahua; Agrobacterium from Nal-Tel; Aureimonas from Palomero Toluqueno; Rhizobium from Camelia; Comamonas from Canilla; and Duganella from Mexicana. The landrace Oloton yielded unique genera (Leuconostoc, Variovorax, Ewingella, Rahnella). Furthermore, 8/62 OTUs (OTU28, OTU40, OTU41, OTU42, OTU54, OTU56, OTU60, and OTU61) in this study were unique to this landrace (Figures 2, 3, S1; Supplementary Table S2).
Descriptively, out of the total 201 silk-associated isolates cultured, 30/201 (~15%) were isolated from maize landrace Camelia, belonging to 9 bacterial genera and 14 unique OTUs (Figure 3; Supplementary Table S2), out of which 10 OTUs were shared with other host accessions (Figures 4, S3). The second highest number of isolates (24/201, ~12%) was cultured from the maize landrace Oloton, belonging to 6 different genera and 9 unique OTUs, out of which only one OTU was shared with other host accessions, suggesting these bacterial OTUs were potentially unique to this specific maize host. The next highest number of isolates (23/201, ~11.5%) was retrieved from landrace Cristalino de Chihuahua, belonging to 7 bacterial genera and 7 unique OTUs, out of which 5 OTUs were shared with other maize accessions (Figures 3, 4). No bacterium was cultured from maize host Confite Morocho. Only a single bacterial isolate was captured from Jala, two from Chapalote, and 5 from Cateto Nortista (Figures 3, S2).
Testing the ability of cultured unpollinated silk-associated bacteria to suppress Fusarium graminearum in vitro
As noted earlier, the fertilization process in maize requires its style tissues (silks) to become exposed to the environment to capture and transmit pollen with its resident sperm nuclei to eggs. This makes progeny seed susceptible to fungal pathogens, which use the same style/silk route to enter maize ears and reach kernels. Therefore, we hypothesized that there may have been selection pressure on silks by indigenous farmers to recruit protective bacteria to combat such fungal pathogens. To screen bacteria for their ability to suppress the growth of Fg in vitro using dual culture assays, a total of 112 unpollinated silk-associated bacterial isolates were selected which maximized both bacterial species and host maize diversity (Supplementary Table S2). In total, 10 bacterial isolates consistently and strongly inhibited Fg growth (Supplementary Table S2; Figure 5B). Among these, duplicate strains were removed that shared the identical 16S rRNA sequence based on multiple sequence alignments which originated from the same maize accession; from these, a single strain was randomly selected, resulting in 6 unique anti-Fg strains. These results were statistically analyzed (Figure 5A). The inhibitory zones of all these 6 strong anti-Fg strains were significantly different (P ≤ 0.05) from the inhibitory zones of LB (the negative control), as well as Proline fungicide (the positive control), except for the strain AS89, which showed no significant difference with Proline (at P ≤ 0.05).
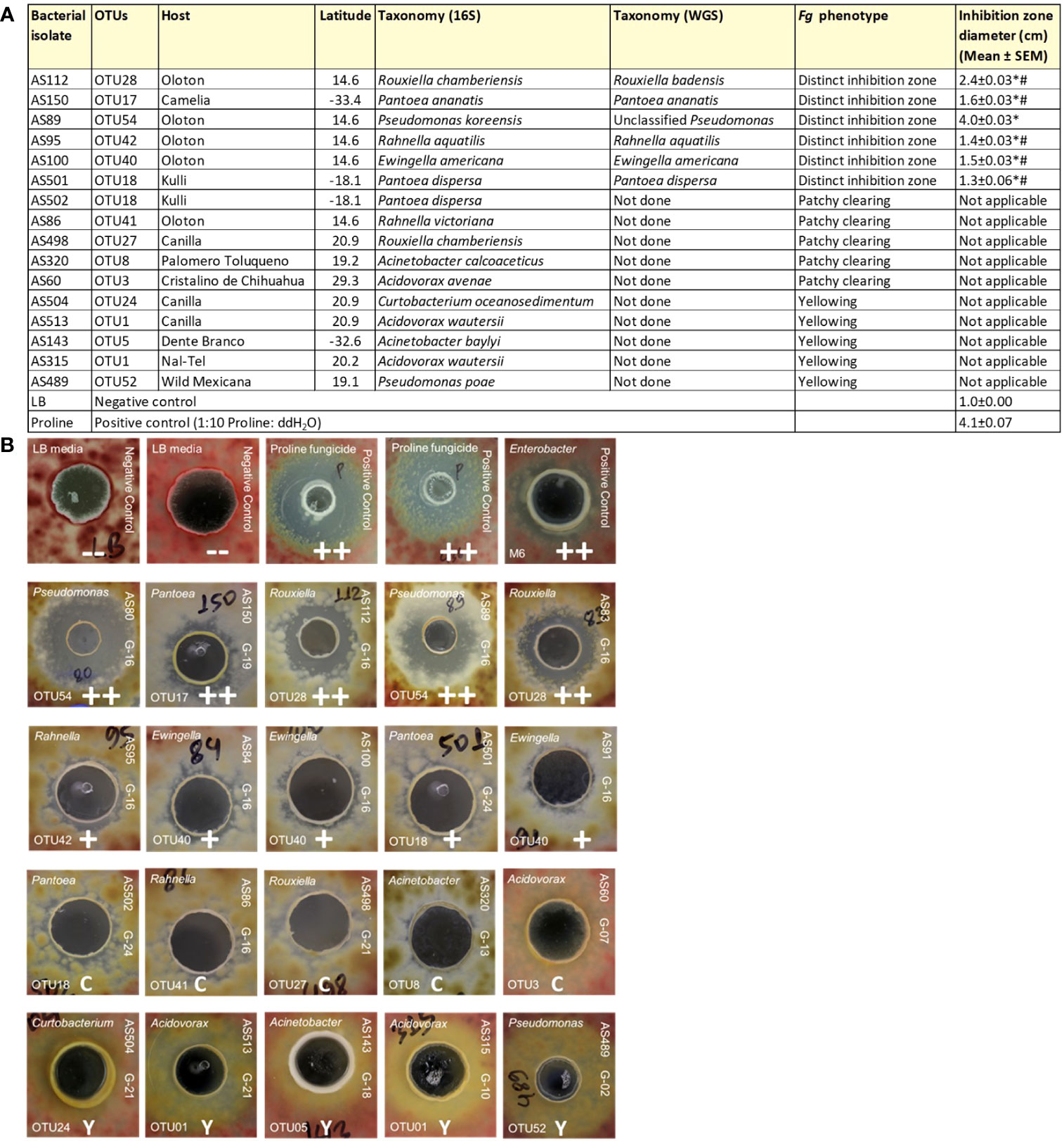
Figure 5 Dual culture assays to test the ability of unpollinated silk-associated bacteria to suppress Fusarium graminearum (Fg) in vitro. In these assays, each agar plate was embedded with Fg (pink background), then a well was created, into which was pipetted a single unpollinated silk-associated bacterial liquid culture or control solution. (A) Summary of the unpollinated silk-associated bacterial isolates that showed anti-Fg phenotypes along with their host source, taxonomies based on 16S and whole genome sequencing, and their zone of inhibition against Fg (in cm). An asterisk (*) indicates that the zone of inhibition is significantly different from the LB buffer negative control; and a number sign (#) indicates that the zone of inhibition is significantly different from the Proline fungicide positive control (P ≤ 0.05, see Methods). (B) Results of the dual culture assay with representative pictures of the effects of individual silk-associated bacteria on Fg. The rows on the top are the control treatments: LB buffer negative control with no zone of inhibition (–), Proline fungicide positive control, and M6 positive control which is Enterobacter sp. M6, previously shown to have anti-Fg activity (positive biological control). The isolates in the second row have a strong zone of Fg inhibition (++); third-row isolates cause minor inhibition (+); fourth-row isolates have a clearing (C) effect on Fg without any organized zone of inhibition; and fifth-row isolates have a yellowing (Y) effect on Fg. For each unpollinated silk isolate, the strain identifier information is noted: 16S BLAST genus prediction (top left corner), OTU number (bottom left corner), isolate sample identifier (ID) (top right side), and the source maize accession ID (bottom right side). The host maize accession IDs are as follows: G16, Oloton; G19, Camelia; G24, Kulli; G21, Canilla; G13, Palomero Toluqueno; G07, Cristalino de Chihuahua; G18, Dente Branco; G10, Nal-Tel; G02, Zea mays ssp. mexicana.
These anti-Fg strains belonged to diverse bacterial genera; however, most of them were cultured from the same host accession, Oloton (Figures 5A, B). Except for strain AS150 (OTU17), all the remaining anti-Fg strains were unique to a specific maize accession: AS112 (OTU28), AS89 (OTU54), AS95 (OTU42), and AS100 (OTU40) were unique to the landrace Oloton, while AS501 (OTU18) was unique to landrace Kulli based on the 16S sequences. Strain AS89, belonging to Pseudomonas (OTU54, predicted to be Pseudomonas koreensis, 99.41% identity) resulted in the largest zone of inhibition (Figure 5A). Of these potent anti-Fg strains, only one strain AS150 belonged to a maize accession that originated from lowland and/or dry environments (500-800 mm rainfall) whereas the remaining strains belonged to hosts originating from highlands with moderate rainfall (>800 to <1270 mm) (Figures 5A, S2).
In addition to the above potent anti-Fg bacteria, some isolates displayed patchy clearing effects with respect to Fg growth without distinct zones of inhibition, while others caused yellowing of Fg (Figure 5B; Supplementary Table S2).
Suppression of Gibberella ear rot by silk-associated anti-Fg strains in greenhouse trials
To test whether the in vitro anti-Fg activities of unpollinated-silk associated bacteria were biologically relevant in planta (e.g. in pollinated silks through which Fg invades grain at the time of pollination), replicated greenhouse trials were conducted where the bacteria were sprayed onto silks before inoculation with Fg pathogen, followed by a repeated bacterial spray. Two unpollinated silk-associated bacterial isolates were selected for greenhouse trials due to space limitations. They were selected based on the results of the dual culture assays and human safety concerns including initial human biosafety risk group assessments based on 16S-based phylogenetic tree reconstruction (Figure S4A, see detailed figure legend) and clinical antibiotic disc susceptibility testing (Figure S4B). Only biosafety Risk Group 1 strains (safest group) with low/average antibiotic resistance were selected. The bacterial treatments were AS112 (Rouxiella OTU28, from landrace Oloton), and AS150 (Pantoea, OTU17, from landrace Camelia) (Figure 5A).
Greenhouse trial results: visual assessment of the percentage of cob length showing disease
Initially, GER disease was scored visually at the whole cob level by measuring the fraction of the cob length that was diseased, a measure of disease spread, since Fg was inoculated at the cob tip. The results showed that the silk-associated bacteria resulted in significant reductions (P ≤ 0.05) in GER disease symptoms compared to the Fusarium + LB buffer treatment (Figure 6). Disease symptom reductions ranged from 87 to 92% in Trial 1, and 83 to 97% in Trial 2. Strain AS112 showed no significant difference (P ≤ 0.05) in disease suppression as compared to the positive Proline fungicide control, in both trials; while strain AS150 showed no significant difference compared to the fungicide treatment in Trial 1. Both of these bacterial strains showed a significant increase (P ≤ 0.05) in grain yield in both trials when compared to the negative control (Fusarium + LB buffer treatment) but no significant difference (P ≤ 0.05) when compared to the Proline fungicide treatment (Figure 6Q).
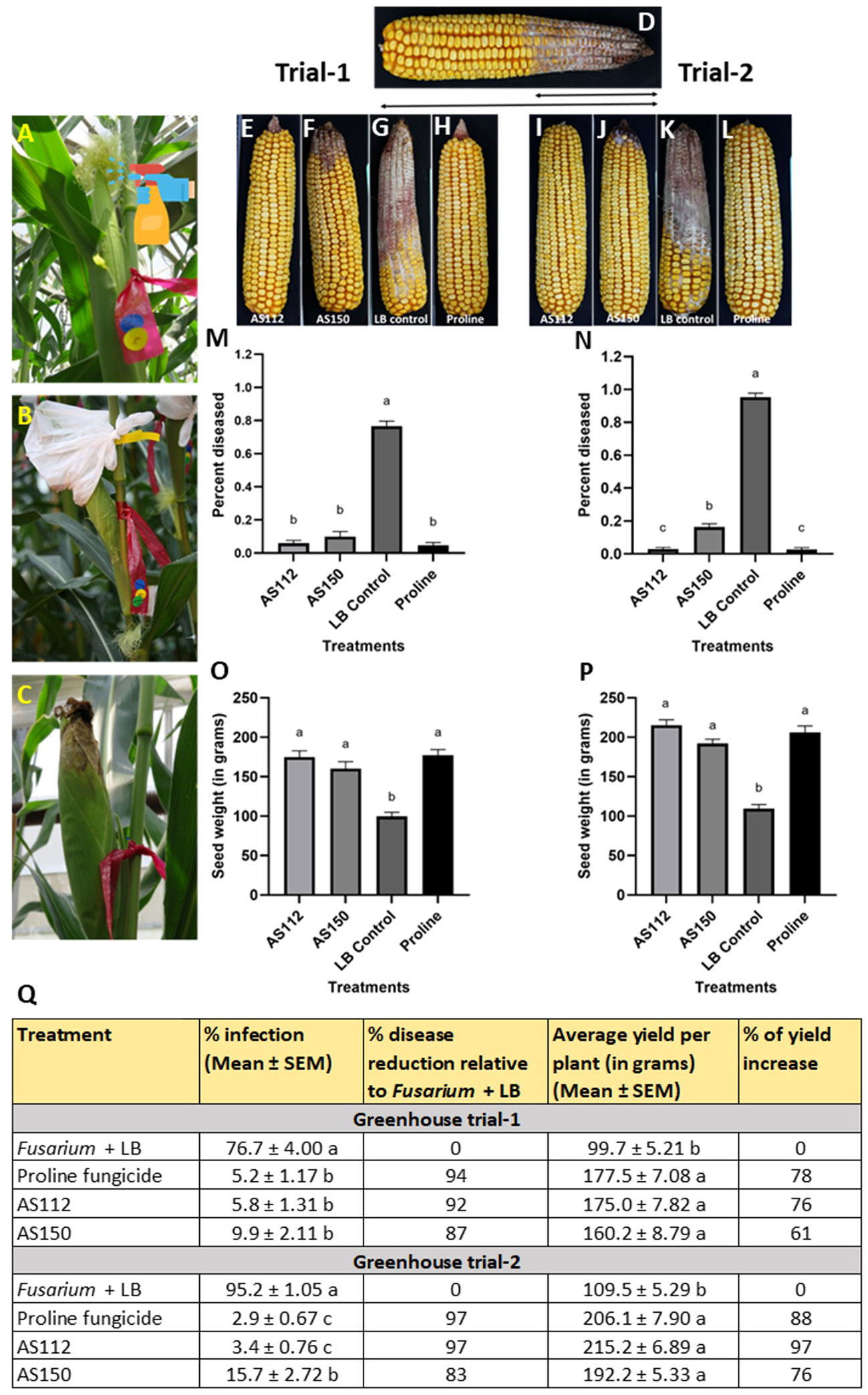
Figure 6 Greenhouse trials to test the ability of unpollinated silk-associated bacteria to suppress Gibberella ear rot (GER) in modern hybrid maize. (A-C) Application treatment procedure: all silks were sprayed with a bacterial strain or a control solution before inoculation with Fusarium graminearum (Fg), and then again after (except Proline). The cobs treated with Fg were covered with plastic bags which were removed 10 days after Fg application. (D) Picture of a mature cob illustrating the visual disease scoring method used which was quantified as the proportion of the diseased cob relative to the total length of the cob. The diseased portion was measured from tip to base (average of 4 measurements from 4 different sides of the cob), then multiplied by 100 to calculate the percentage of disease. (E-H) Representative treated cobs from each treatment in Trial 1, and (I-L) Trial 2. (M-P) Quantification of the effects of different treatments on GER suppression: (M, N) percentage diseased ear in (M) Trial 1 and (N) Trial 2, and (O, P) average seed weight (in grams) in Trial 1 (O) and Trial 2 (P). (Q) The effect of the bacterial strains on the percentage disease reduction and percentage yield increase relative to the Fusarium + LB buffer treatment (negative control). For both measurements, n= 6 blocks per treatment, completely randomized (with 4 plants per treatment per block). Error bars indicate the standard error of the mean (SEM). The different letters on the top of the histograms (M–P) and to the right of infection or yield measurements in (Q) indicate that the mean values are significantly different from each other (P ≤ 0.05, see Methods).
Greenhouse trial results: visual assessment of disease severity at the individual kernel level
Individual kernels of the treated cobs that were visually assessed at the whole cob level were again scored for ear rot symptoms. A visual rating scale from 1 to 4 was used with “4” indicating the most severely infected kernels, and “1” assigned to kernels showing no visual disease symptoms (Figure 7A). In both greenhouse trials, both silk-associated bacterial sprays resulted in a significant increase (P ≤ 0.05) in the percentage of seeds with no visual disease symptoms (scale of 1), compared to the Fg-only treated kernels (Figure 7B; Supplementary Tables 4A.7, 4A.8, 4B.7, 4B.8) and a simultaneous decrease in the percentage of seeds with severe disease symptoms (scale of 4) (Figure 7B; Supplementary Tables 4A.1, 4A.2, 4B.1, 4B.2). Among these anti-Fg strains, AS112 (Rouxiella OTU28, from Oloton) had a significantly lower percentage of diseased kernels (scale of 4) as compared to AS150 (Pantoea OTU17, from Camelia) (Supplementary Tables 4A.1, 4A.2, 4B.1, 4B.2), consistent with the whole cob level results.
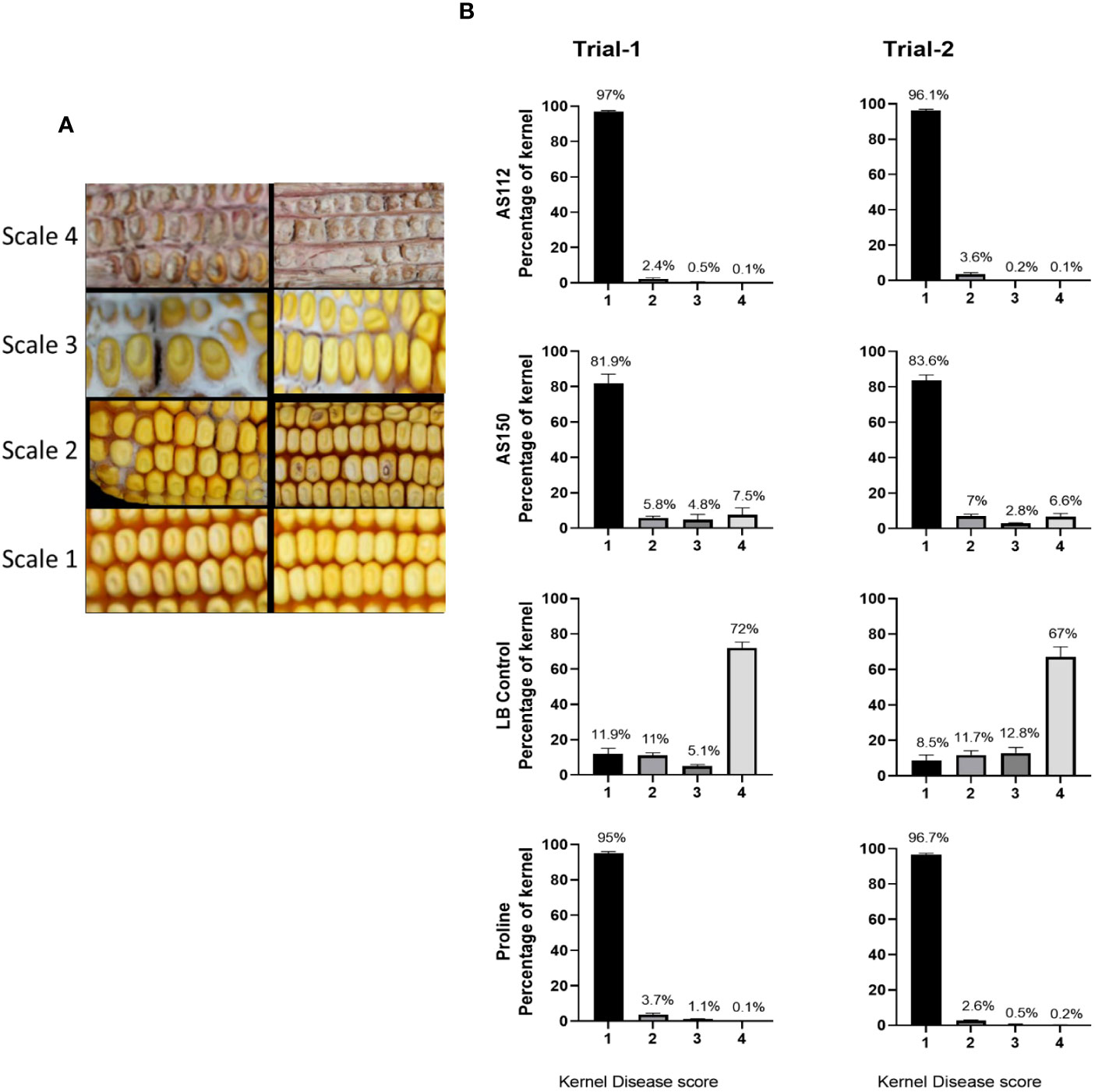
Figure 7 Testing the ability of unpollinated silk-associated bacteria to suppress Gibberella ear rot (GER) in modern hybrid maize based on visual disease severity assessment at the individual kernel level. All silks were sprayed with a bacterial strain or a control solution before inoculation with Fusarium graminearum, and then again after Fusarium (with the exception of Proline). (A) Representative photos of maize kernels showing the scale used for assessing the kernel disease severity visually. The scale ranged from 1 to 4, with a value of 1 indicating no infection; 2 indicating low infection; 3 indicating medium infection, and 4 indicating highly infected. The two images associated with each scale value indicate the range of phenotypes assigned to that disease score (see Methods). (B) The result of individual kernel disease severity assessment is presented in percentages, which is the fraction of the kernel population with each disease score. Each percentage is the mean of 24 cobs (plants). Proline is the positive fungicide control; LB is the negative buffer control; AS112 and AS150 are the bacterial treatments used in the trials. All error bars indicate the standard error of the mean (SEM).
Greenhouse trial results: grain mycotoxin accumulation
We hypothesized that indigenous farmers in the Americas may have inadvertently selected unpollinated silk-associated bacteria that could suppress Fg-associated mycotoxins in the grain, by associating diseased seed with human and/or livestock illness following their consumption. To test this hypothesis, seeds of the same treated cobs reported earlier for disease symptoms were pooled from each plant per treatment per block, resulting in a total of 6 replicates per treatment. Liquid chromatography-tandem mass spectrometry (LC-MS/MS) was used to quantify concentrations of the following Fg-producing mycotoxins in grain immediately after harvest: deoxynivalenol (DON), 3-acetyl-deoxynivalenol (3ADON), 15-acetyl-deoxynivalenol (15ADON), DON-3-glucoside, and zearalenone.
Overall, both silk-associated bacterial strains resulted in significant reductions (P ≤ 0.05) of mycotoxins ranging from 70-100% (except DON-3-glucoside in Trial 2) compared to the Fg + LB buffer-treated plants (Table 2). Furthermore, treatment with strain AS112 showed higher reductions in mycotoxin concentrations in Trial 2, while strain AS150 was better in Trial 1 (Table 2). This result also matched the earlier cob-level visual disease scoring.
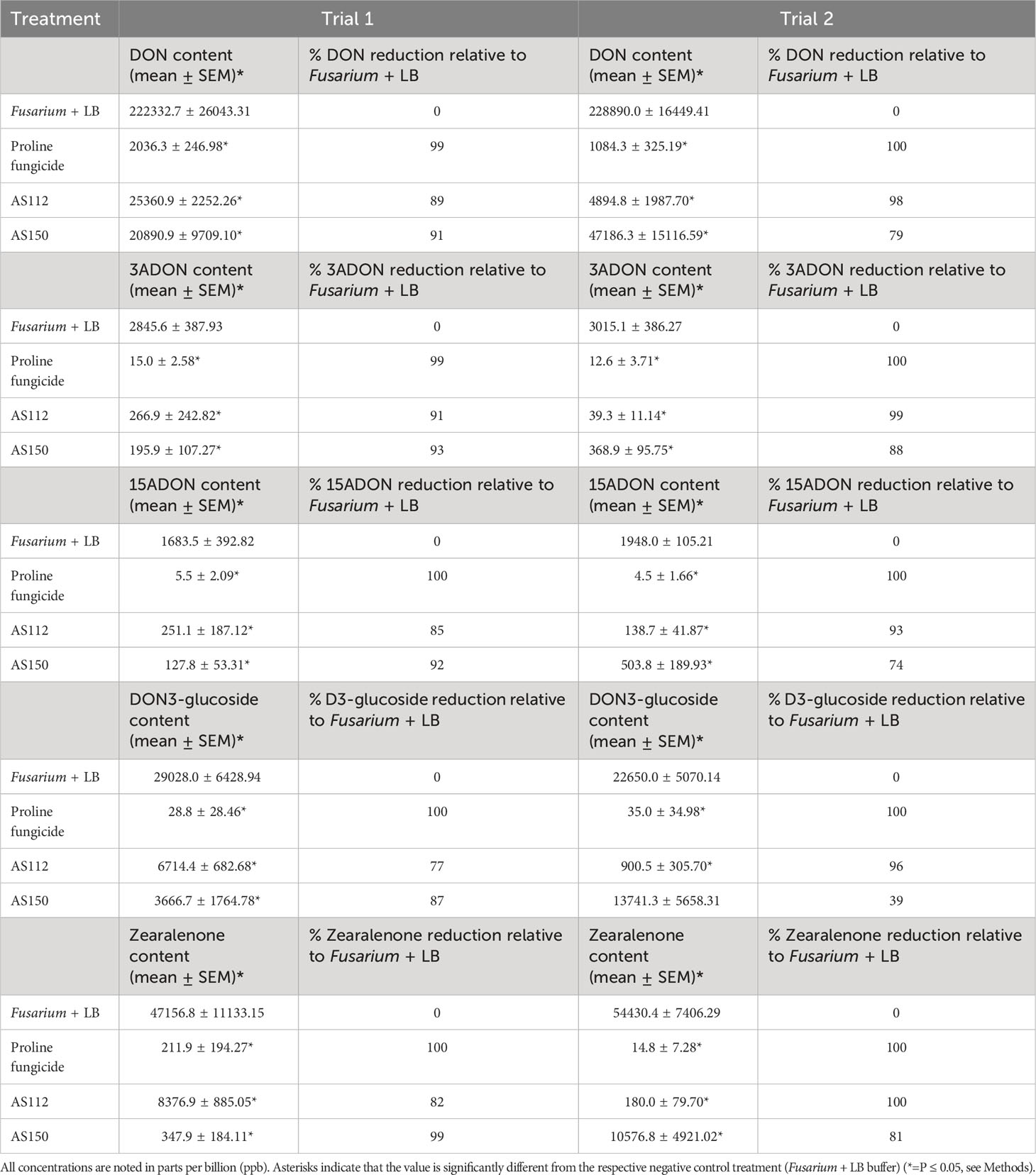
Table 2 Results of greenhouse Trials 1 and 2, showing the reduction in mycotoxin accumulation in Fg-infected grains following treatment with the unpollinated silk-associated anti-Fg bacterial strains.
In vitro interactions between silk-associated anti-Fg bacterial strains and Fg using light microscopy
The two silk-associated bacteria (AS112 and AS150) that inhibited Fg growth in vitro and in planta were visualized on microscopic slides to better understand their antifungal mode(s) of action (Figure 8). Each bacterium was grown alongside Fg, or a buffer control (LB) (Figure 8A), then stained with Evans blue, a vitality stain that stains fungal mycelia blue when dead (Gaff and Okong’O-Ogola, 1971). Microscopic analysis showed that Fg hyphae in contact with strain AS112 remained intact and appeared normal but stained darker blue compared to LB buffer exposure (Figures 8B, C). In the case of strain AS150, however, the Fg hyphae near the bacterial strain not only stained dark blue but showed morphological deformities (Figures 8D, E). Since AS112/AS150 exposed Fg hyphae took up more Evan’s blue, suggestive of death, we conclude that these strains have fungicidal activity against Fg.
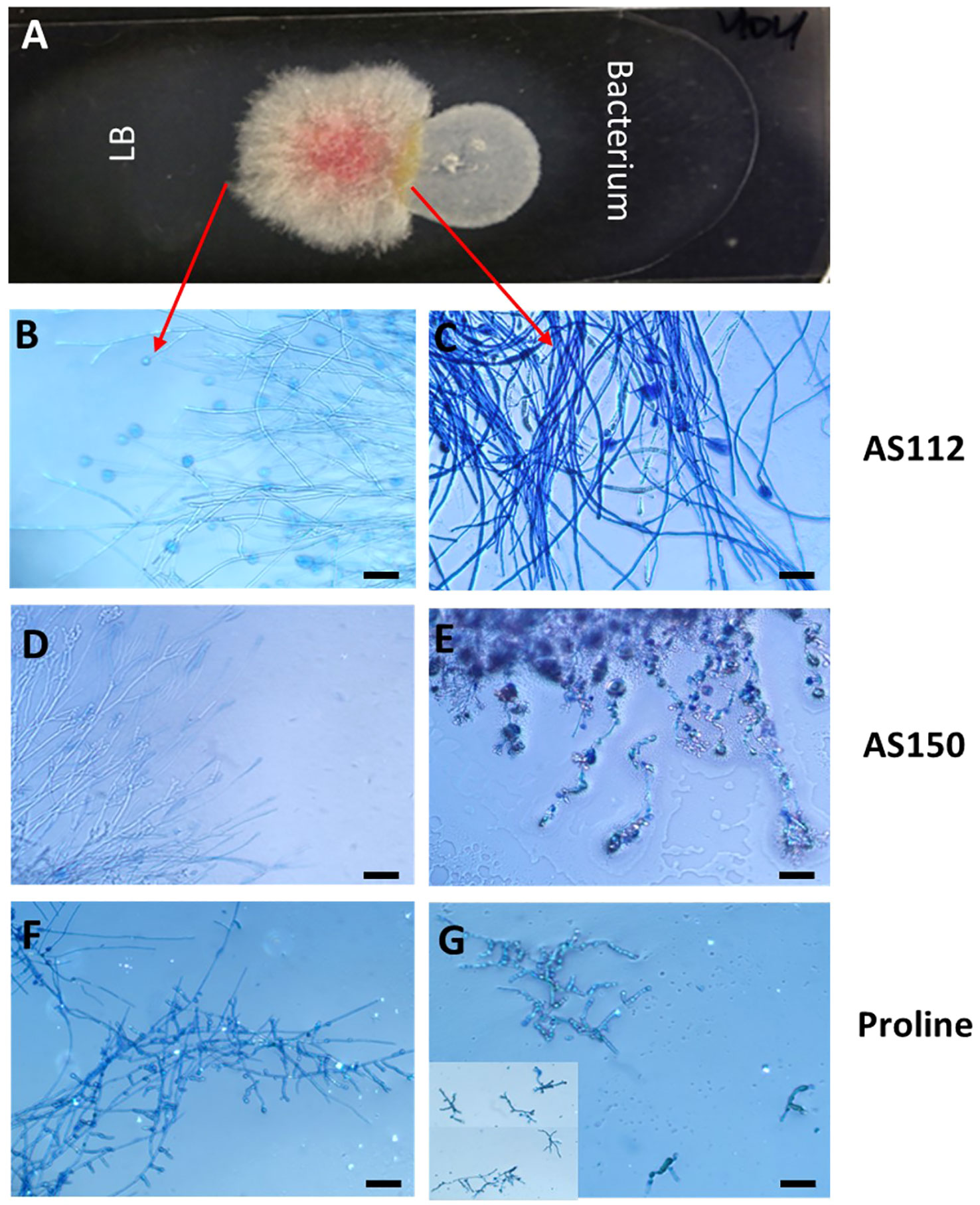
Figure 8 The interactions of unpollinated silk-associated anti-Fg bacterial strains with Fusarium graminearum (Fg) in vitro after staining with the vitality stain, Evans blue. (A) The methodology used: Potato dextrose agar-coated microscope slide with Fg in the center, flanked by an anti-Fg bacterial strain (right) or LB buffer control (left). After 24 h of co-incubation, Evan’s blue was added and then visualized using a light microscope: only dead Fg hyphae take up the stain. (B, D, F) Fg hyphae on the control side (no bacteria) and (C, E, G) the corresponding Fg hyphae on the side exposed to the different treatments as follows: (C) strain AS112, (E) strain AS150, and (G) Proline fungicide. The inset in panel (G) is to show multiple Fg hyphae. The scale bar in all images is 5 µM.
Confocal fluorescence imaging of interactions between AS112 and Fusarium graminearum on living silks
The previous greenhouse trials indicated AS112 could inhibit Fg when sprayed onto silks, which was then visualized on a light microscope, suggesting it has fungicidal activity in vitro. To further understand the mode of action of the anti-Fg bacterial strain AS112 on silk channels, intact silks from detached cobs were used, co-applied with fluorescently tagged AS112 and Fg, and visualized using confocal scanning fluorescence microscopy. AS112 was tagged with fluorescent protein DsRed (digitally transformed to a blue colour), Fg using GFP (green colour), and the silk cells were stained with propidium iodide (red colour) (Figure 9).
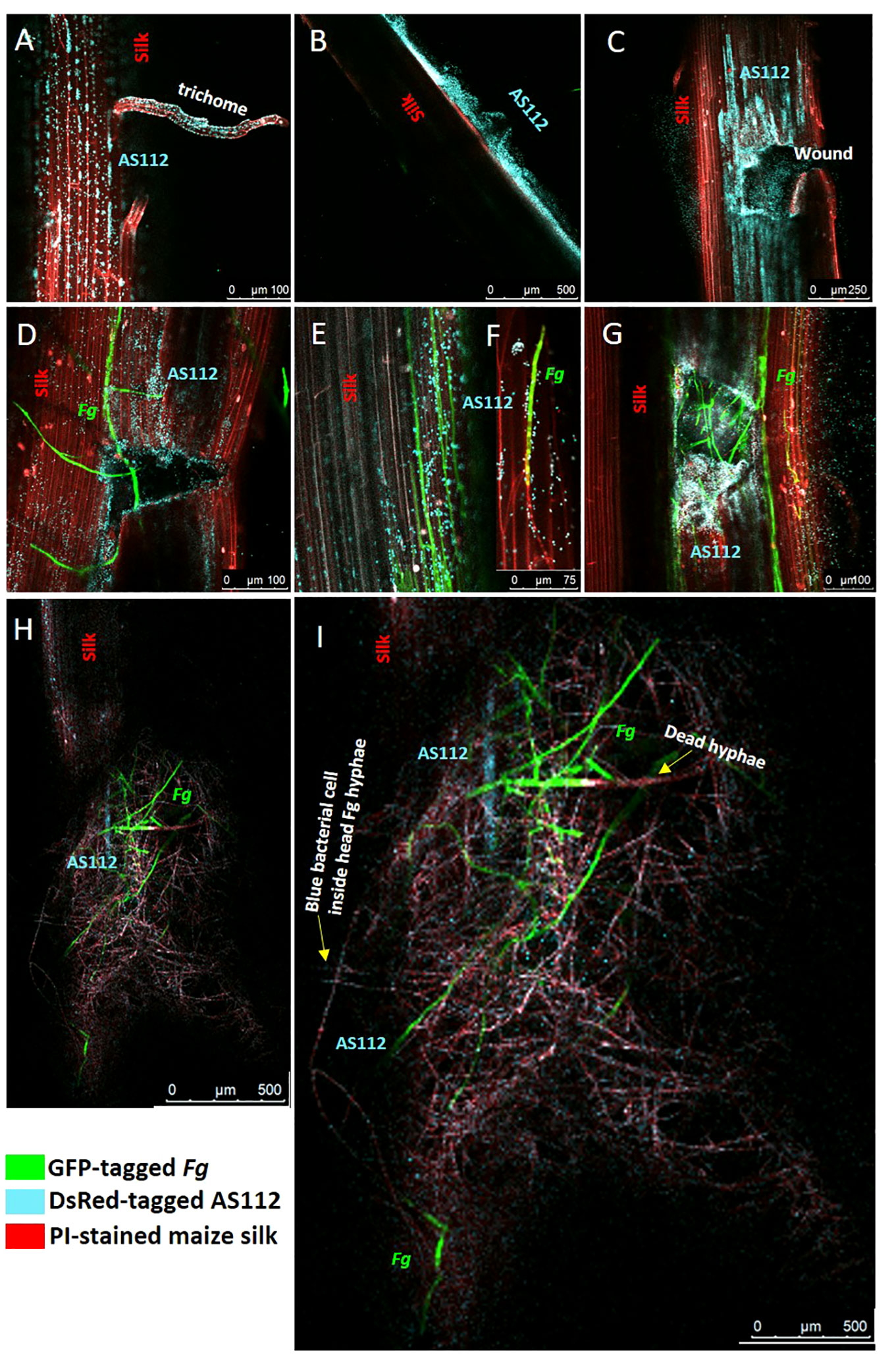
Figure 9 Confocal fluorescence microscopy imaging of maize silks showing interactions between AS112 and Fusarium graminearum (Fg). AS112 was tagged with DsRed, but false coloured blue, while Fg was tagged with GFP (green), and silks were stained with propidium iodide (red). Some silks were mechanically wounded to mimic insect damage, which promotes Fg infection. (A) AS112 colonizing a stigmatic trichome in the absence of Fg. (B) AS112 forming a biofilm on the silk epidermis in the absence of Fg. (C) AS112 heavily colonizing at and near a wound site in the absence of Fg. (D) AS112 colonizing a wound site in the presence of Fg. (E, F) AS112 colonizing Fg hyphae on the silk epidermis, leading to apparent Fg hyphal death (hyphae turning red and no longer expressing GFP). (G) AS112 heavily colonizing a wound site in the presence of Fg. (H, I) Residual AS112 colonization associated with a large mass of apparently dead Fg hyphae (red without GFP expression). Once Fg hyphae appear to die, the bacterial cells fluoresce less blue (this image was brightened to make bacterial cells more visible).
The confocal results showed that before Fg infection, the anti-Fg bacterial strain AS112 could: colonize trichomes (stigmatic hair), which are also known to be the susceptible entry points of Fg (Figure 9A); form biofilm structures on the silk epidermal surface (Figure 9B); and heavily colonize silk wound sites (Figure 9C). After Fg infection on silks, AS112 could colonize wound sites infected with Fg but seemed to be more attracted to the wounds rather than to Fg (Figures 9D, G); in other images, AS112 could colonize the Fg hyphae directly (Figures 9E, F), and was associated with large masses of dead Fg hyphae (Figures 9H, I). Overall, combined with the ability to suppress Fg disease in the greenhouse trials, these results suggested that AS112 has both preventative and protective activities against Fg on living silks.
Effect of the anti-Fg bacterial strains on protecting seed progeny after harvest
To further protect the genetic contribution of the female gametes, we hypothesized that the maternally derived silk-associated bacteria may protect progeny seed from the further progression of Fg after the seed had dispersed from the mother plant. To test this hypothesis, Fg field-infected grain was treated with the silk-associated anti-Fg bacterial strains or controls (LB buffer as the negative control; and Proline fungicide as the positive control), then stored for 8 months under low humidity conditions (sealed envelopes) or high humidity (sealed Falcon tubes), the latter known to promote Fg growth (Munkvold, 2003; Thapa et al., 2021), and then finally quantified for DON mycotoxin concentrations using ELISA. The low humidity condition resulted in a non-significant difference (P ≤ 0.05) in DON mycotoxin between the negative and positive controls. However, under high humidity conditions, maize seeds treated with strain AS150 showed a significant reduction (P ≤ 0.05, equaling 72%) in DON concentration, compared to the LB buffer negative control (Table 3). The impact of strain AS112 was only significant at P ≤ 0.10 (mean reduction of 53%) with respect to its efficacy compared to the buffer control (Table 3).
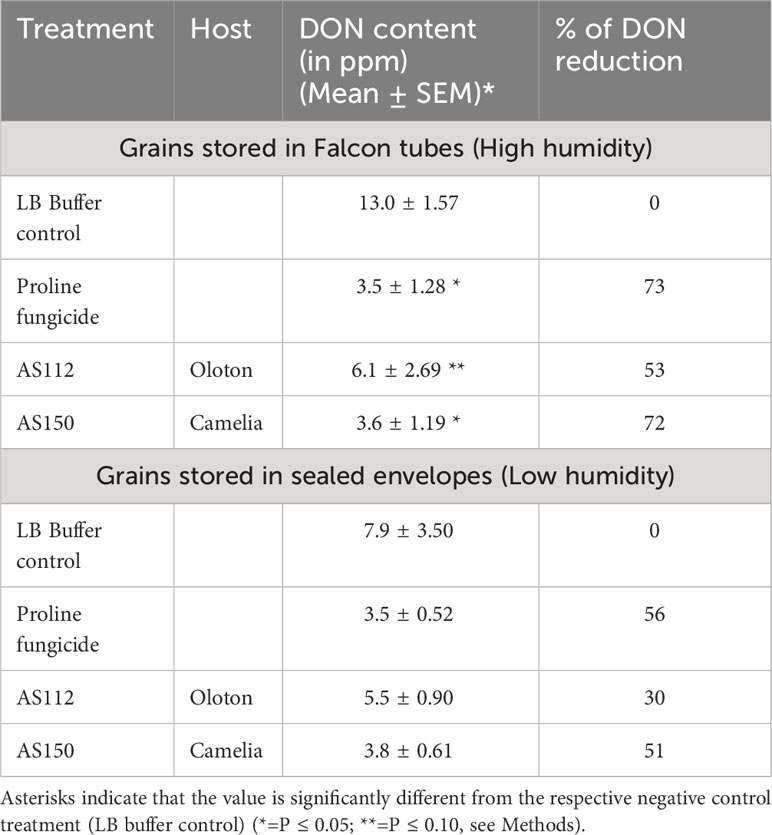
Table 3 Testing the ability of silk-associated anti-Fg bacterial strains to protect seed after harvest.
Discussion
During the fertilization process in flowering plants, there would be selection on the maternal parent to promote progeny fitness by protecting the exposed style/silk (male gamete fertilization channel) and their connected ovules and future seeds from environmental pathogens such as Fg, which enter from the air and also hitchhike on the pollen (Card et al., 2007; Thompson and Raizada, 2018; Khalaf et al., 2021). Here, anti-fungal activity was discovered in bacteria cultured from unpollinated maize silks of ancient maize accessions, and their beneficial roles were validated in living silks of a modern maize hybrid. These bacteria were sprayed onto silks of modern maize to mimic the route via which Fg enters developing seeds. The assays suggested that the silk-associated bacteria could reduce Fusarium infection along the male gamete fertilization channel by forming what appeared to be protective barriers on known Fg entry points on silks (trichomes, wound sites) including forming dense biofilms on the silk epidermis, but also subsequently having the ability to colonize Fg hyphae directly after infection. Additionally, these maternally derived bacteria could protect the seed after harvest, when no longer attached to the mother.
Comparison to prior maize silk microbiome studies
Here, the unpollinated maize silk library was found to be dominated by the bacterial genera Pseudomonas and Pantoea; furthermore, we showed that the most potent anti-Fg bacterial strains from the in vitro dual culture assays belonged to these two genera (Figures 3, 5). This is only moderately consistent with the prediction from a previous study by Khalaf et al. (2021) which reported that Pantoea but not Pseudomonas increased in abundance in pollinated silks of North American maize in response to Fg infection. Nevertheless, in that study, Pseudomonas constituted 9-13% of all read counts, the third most abundant genus, but it was abundant in both Fg-infected and healthy silks. It might be, similar to our findings here with Rouxiella strain AS112 (Figure 9), that some silk-associated bacteria can defend against Fg before and after infection and hence are always present. A recent study by Diniz et al. (2022a) similarly reported that silks exposed to pollen contained Pseudomonas.
Khalaf et al. (2021) further reported that the genera Herbaspirillium, Delftia, Stenotrophomonas, and Sphingomonas increased in abundance in silks after Fg infection; though Herbaspirillium and Sphingomonas were cultured here, they were not identified as anti-Fg strains. Furthermore, other silk-associated bacterial genera reported by Diniz et al. (2022a) (such as Burkholderia, Achromobacter, Serratia and Bacillus) were not cultured in this study. Various studies have shown that diverse bacteria can have anti-Fg activity including Bacillus strains (Zhao et al., 2014; Mahmoud, 2016; Dimkić et al., 2022; Jimenez-Quiros et al., 2022).
There were some additional differences between this study and the prior studies. First, in both prior studies, unlike this study, the silks may have contained pollen-transmitted bacteria. Second, Khalaf et al. (2021) used North American rather than Latin American maize accessions and relied on short-read 16S-V4 region sequencing, which has limited taxonomic resolution. Finally, this study differed from Diniz et al. (2022a) in terms of the culture media and/or did not rely on culturing in the case of Khalaf et al. (2021).
Preliminary evidence for indigenous farmer selection on silk microbiomes in the Americas
Indigenous peoples may have unknowingly selected the style microbiome to defend against an environmental pathogen that enters during pollination. Here, the in vitro dual culture assay identified diverse silk-associated bacterial genera from diverse maize accessions in the Americas as having anti-Fusarium activities (Figure 5). Since the anti-Fg bacteria belonged to only a subset of maize accessions (<50%), one possibility is that only specific indigenous peoples may have successfully selected for these traits (i.e. those that faced Fusarium grain disease). This interpretation requires further validation, as the plants in this study were grown in a common field for a single season; hence it is entirely possible that some of the silk-associated bacteria were derived from the Canadian field soil or soil associated with the seed banks that collected the accessions (e.g. CIMMYT in Mexico), rather than being vertically transmitted.
Interestingly, most accessions in this study that originated from the center of maize diversification (Oloton, Palomero Toluqueno, Cristalino de Chihuahua) (Matsuoka et al., 2002) were sources of anti-Fg bacteria, of which Oloton from Guatemala was the source of multiple potent anti-Fg strains (Figure 5A). Fungal pathogens associated with maize including Fusarium are prevalent in Guatemala (Rodrigo Mendoza et al., 2017). Oloton is a highland maize that was later introduced to Mexico, and is a staple food for the Mixe indigenous people in the Sierra Mixe region in southern Mexico (Pskowski, 2019); it is known to be highly resistant to pests and pathogens (CONABIO, 2017). It is noteworthy that this maize landrace predominates in a highly humid climate, which is known to favor fungal pathogens. Additionally, DON mycotoxin promotes Fg infection and suppresses pollen tube growth (Tejaswini, 2002; Mudge et al., 2006; Kačániová et al., 2011; Kostić et al., 2019), and also decreases host immunity (McCormick et al., 2011). These combined forces may have resulted in strong selection pressure for the Mixe people to have selected for silks to carry bacteria that could suppress Fusarium and DON directly (by degrading DON) or indirectly (by suppressing Fg) in order to protect pollen tube growth and preserve successful fertilization and hence seed yield. More evidence is needed to support this hypothesis, including whether silks directly collected from the Mixe region possess these anti-Fg bacteria. However, bacteria with anti-Fg traits were also cultured from the maize landrace Kulli which originated in Bolivia, and Camelia which originated in Chile, far away from the center of maize diversification.
It is compelling that all the OTUs shown to have anti-Fg activity in vitro were apparently host-specific (Figure 5). Also compelling is that anti-Fg bacterial strains belonged to diverse genera. Together, these results lead to the hypothesis that indigenous farmers selected for anti-Fusarium bacteria independently in the Americas. This selection would have occurred unknowingly by farmers, perhaps by selecting only visually healthy seed for replanting, or associating these disease symptoms with sick livestock or family members after ingestion of grain containing associated mycotoxins.
Another possibility is that indigenous farmers across the Americas were selecting silk-associated bacteria not only to combat F. graminearum but also other Fusarium species. Different Fusarium species and their associated mycotoxins have been reported to be prevalent in Mexico and Central America (Odjo et al., 2022). GER caused by Fg pathogen is favored by high levels of moisture during silking, followed by moderate temperatures and high precipitation (Sutton, 1982). However, in this study, the maize silk-associated anti-Fusarium bacterial isolates were cultured from maize hosts inhabiting environments ranging from wet to dry. F. verticilloides, which is prevalent across the Americas, favors dry environments (Miller, 2001; Sancho et al., 2017; Pfordt et al., 2020). Furthermore, a recent study (Diniz et al., 2022b) reported that some silk-associated bacterial isolates suppressed maize stalk rot severity caused by F. verticillioides in greenhouse trials, though they did not test F. graminearum. Intriguingly, the maize landrace Camelia from Chile, which possessed anti-Fg bacteria, is known to have resistance against ear rots caused by F. verticillioides (USDA-ARS, 2018). Chilean maize does suffer from mycotoxins associated with Fg including DON (Raj et al., 2021). Future studies are needed to test whether some of the anti-Fg isolates described in this study have broad-spectrum anti-Fusarium activity.
Silk-associated bacteria have the potential to defend the male gamete fertilization channel against a fungal pathogen
Here, the dual culture anti-Fg assays identified six silk-associated bacterial isolates that strongly suppressed Fg in vitro as follows:
Rouxiella badensis (AS112)
This strain was derived from maize accession Oloton which was sprayed onto silks, in replicated greenhouse trials, resulting in almost complete suppression of GER disease (up to 97%) and related mycotoxin accumulation (in general, 77-91% reductions in DON, 3ADON, 15ADON, DON3-glucoside, ZEA) and significantly increased grain yield (76-97%). Additionally, AS112 demonstrated fungicidal activity against Fg in vitro, while confocal imaging on living silks showed that AS112 could protect silks before infection by forming what appeared to be protective barriers on silks, and after infection by colonizing and apparently killing Fg. The former mechanism is consistent with a study from pear which showed that epiphytic style-associated bacteria could prevent the colonization of the bacterial pathogen Erwinia amylovora through pre-emptive exclusion (Stockwell et al., 1999). It is noteworthy that AS112, a highly effective silk colonizer, encodes two Type III secretion system operons (Supplementary Table 5). It has been reported that the Type III secretion systems facilitate the secretion of effectors that suppress host defense, to promote stable colonization (Zboralski et al., 2022). In addition, AS112 could also protect progeny seeds from further Fg progression after being harvested from the mother plant, although high concentrations of bacteria were used. The preliminary AS112 genome mining results showed the presence of chitinase; a biosynthesis gene encoding an anti-fungal metabolite (phzF for phenazine); and a biosynthetic gene encoding a signaling compound known to facilitate host resistance (acetoin) (Supplementary Table 3). However, these observations must be further validated experimentally.
R. badensis has not been previously reported to suppress GER disease in maize to the best of our knowledge. It has been reported as a novel biocontrol agent against the postharvest fungal pathogens Botrytis cinerea and Fusarium brachygibbosum in strawberry fruit, affecting mycelial development (Morales-Cedeño et al., 2021). It also showed different levels of in vitro antagonism against 20 fungal pathogens in berries (Morales-Cedeño et al., 2021). Here, we showed that R. badensis (AS112) seemed to colonize wound sites on silks more than Fg hyphae. However, AS112 could apparently kill Fg by colonizing the hyphae. Combined, one interpretation of these results is that AS112 has a saprophytic mode of action, prioritizing nutrient-rich host wound sites, followed by killing and feeding on dead Fg hyphae. R. badensis colonizes decaying strawberry fruits and could suppress Fusarium brachygibbosum and Botrytis cinerea in fungal growth inhibition bioassay on strawberry fruit (Morales-Cedeño et al., 2021), consistent with it being a saprophyte in plants that perhaps protects its territory from competitors. Furthermore, despite being one of the best anti-Fg strains in vitro and confirmed by greenhouse trials, AS112 did not perform well as strain AS150 in protecting mature seed progeny after harvest. This could be because AS112 might be adapted to moist and nutrient-rich environments such as silk tissues but not dry seeds. Indeed, in addition to inhabiting decaying strawberry fruits, previous studies have shown that R. badensis prefers peat bog soil (Fléche-Matéos et al., 2017) and the human intestine (Yahfoufi et al., 2021) – both moist environments. Additionally, strain AS112, when cultured on media plates in the lab, could not survive for more than 4-5 days, consistent with it favoring nutrient-rich conditions.
Pantoea ananatis (AS150)
This strain was derived from maize accession, Camelia. Similar to AS112, it was sprayed onto silks, in replicated greenhouse trials, resulting in suppression of GER disease (up to 87%) and related mycotoxins accumulation (in general, 87-99% reductions in DON, 3ADON, 15ADON, DON3-glucoside, ZEA) and significantly increased grain yield (61-76%). AS150 also demonstrated fungicidal activity against Fg in vitro similar like AS112. Additionally, AS150 could also protect progeny seeds from further Fg progression after being harvested from the mother plant. P. ananatis has not been previously reported to suppress GER disease in maize though it has been shown to reduce DON mycotoxin by 50% in wheat infected with Fg (Deroo et al., 2022). Preliminary genome mining results (Supplementary Table 3) show that the genome of AS150 encodes phzF, chitinase and a gene required for acetoin biosynthesis.
P. ananatis has been reported as a maize seed endophyte (Rijavec et al., 2007; Johnston-Monje and Raizada, 2011). In addition to suppressing Fg in wheat, P. ananatis has been shown to inhibit the growth of the maize-associated fungus Lecanicillium aphanocladii in vitro (Rijavec et al., 2007). In rice, it suppressed the causative agent of rice blast disease, Pyricularia oryzae (Someya et al., 2003), as well as enhanced crop growth and increased yield (Megías et al., 2016; Megías et al., 2017). Interestingly, P. ananatis was reported to rapidly colonize plant wounds before the establishment of the pathogen (Botrytis cinerea) thereby suppressing mycelial growth and disease symptoms (Roca-Couso et al., 2021).
The other four silk-derived bacteria with strong anti-Fg activity in vitro and anti-fungal genes (Supplementary Table 3), were also not reported to control GER disease in maize but were shown to suppress Fg, other Fusarium species, or other pathogens in other crops. Specifically:
Pseudomonas koreensis (AS89)
Pseudomonas koreensis (AS89) was derived from maize accession Oloton, similar to AS112. This strain showed the largest ring of Fg-inhibition in vitro, even better than Proline fungicide (the positive control). However, it was not chosen for greenhouse trials because of human biosafety concerns (tolerance to specific clinical antibiotics) (Figure S4B). P. koreensis was also previously not reported to control GER in maize. It was reported to suppress Cephalosporium maydis, the causative agent of late wilt disease in maize, in greenhouse trials and field experiments (Ghazy and El-Nahrawy, 2021). Additionally, P. koreensis significantly reduced disease caused by Pythium ultimum in hydroponic tomato cultivation (Hultberg et al., 2010a) and Phytophthora infestans which causes potato late blight (Hultberg et al., 2010b).
Rahnella aquatilis (AS95)
Rahnella aquatilis (AS95) was also derived from Oloton which inhibited Fg in this study. Previous studies have reported that R. aquatilis as biocontrol agents for different diseases but not for GER in maize. For example, R. aquatilis, a rice isolate showed antagonism against F. graminearum and Magnaporthe oryzae in vitro (Sun et al., 2020). It is also reported as a biocontrol agent against grapevine crown gall (Chen et al., 2007), apple fire blight (Abo-Elyousr et al., 2011), and fruit storage rots (Navarta et al., 2011).
Ewingella americana (AS100)
AS100 was again derived from Oloton, and was one of the anti-Fg strains in this study. E. americana has not been previously shown to suppress GER disease in maize. It has been reported as a maize seed endophyte (Shiomi et al., 2008), able to enhance maize growth (Rana et al., 2021) and suppress northern corn leaf blight disease in maize (Shiomi et al., 2015).
Pantoea dispersa (AS501)
It was cultured from maize accession, Kulli, and showed Fg inhibition in vitro. P. dispersa is known to be an endophyte of maize and wheat, reported to promote growth in maize, wheat, and rice (Suman et al., 2020; Fernando Amezquita-Aviles et al., 2022; Shovitri et al., 2022). Most relevant to this study, it has been shown to be antagonistic to Fusarium moniliforme as well as Rhizoctonia solani pathogens of maize (Swamy et al., 2022). It strongly inhibited mycelium growth and spore germination in vitro of the pathogenic fungus Ceratocytis fimbriata, the causative agent of black rot in sweet potatoes, and inhibited its growth in planta, suggesting P. dispersa has fungicidal rather than fungistatic activity (Jiang et al., 2019).
Exploiting plant maternal reproductive tissue as a source of disease-suppressive bacteria
In humans, the resident microbiota of the female reproductive tract plays a role in the maintenance of the mother’s health as well as in protecting babies from environmental infections before and after birth (Feng and Liu, 2022). In flowering plants such as maize, the transmission of different fungal pathogens occurs during the flowering phase when the male parent (pollen grains) and the maternal parent contact each other indirectly during pollination (Antonovics, 2005). Such fungal pathogens including Fg affect the health of future progeny seed in part by depositing hazardous mycotoxins, ultimately jeopardizing the contribution of the male gamete. DON mycotoxin also reduces the health of humans, cattle, poultry, and swine, especially lactating animals, with strict limits placed by different jurisdictions (Canadian Food Inspection Agency, 2015) which ultimately limit the profits of farmers globally (Konkin et al., 2022). In maize, pollination is correlated with changes to the innate plant defense system; the flavonoid antioxidant capacity decreases as maize silks mature, which might contribute to increased ear rot susceptibility after pollination (Rahman and Wan Rosli, 2014). To control such fungal pathogens, this study adds to emerging studies that the natural microbiome of disease-target tissue may have evolved or been selected to suppress disease (Stockwell et al., 1999; Cui et al., 2021; Khalaf et al., 2021). In particular, the style/silk provides an excellent niche for microbial colonization since it is rich in lipids, proteins, carbohydrates, minerals, and vitamins, and also has a high moisture content (Guo et al., 2009). Moving forward, our prior study reported seasonal variation of the pollinated silk-associated microbiome in response to Fg (Khalaf et al., 2021); therefore, the next focus should be on metagenome profiling and breeding for host genes that stabilize the defensive silk microbiome across seasons, environments and varieties. Since several studies, including now this one, have reported that wild and ancient relatives of crops possess an untapped reservoir of beneficial microbes (Johnston-Monje and Raizada, 2011; Yokoya et al., 2017; Preece and Peñuelas, 2020), the style tissues of diverse modern crops and ancient landraces selected by indigenous farmers, should be explored for their microbiome.
Conclusion
In plants and animals, the mother has a major role in assuring the fitness of her future progeny (Galloway, 2005; Chettoor et al., 2016; Nguyen et al., 2021). In plants, the maternal-style tissue becomes susceptible to environmental pathogens due to its exposure to the ambient environment during the fertilization process. Here, we presented evidence from maize to suggest that in plants, the female parent has evolved a strategy to use its style microbiome to proactively defend the male gamete fertilization channel against future fungal attacks, thereby protecting the progeny and hence the genetic contribution of the mother. Specifically, in vitro and in planta experiments demonstrated that maize silks host bacteria that possess anti-Fusarium traits, perhaps selected independently by specific indigenous farmers in the Americas, at and distant from the site of maize diversification, though we cannot rule out that these bacteria originated later through horizontal transmission into silks. Confocal microscopy-based imaging suggests that one of these silk-associated bacteria protects the male gamete fertilization channel from Fg by a novel mechanism, specifically by colonizing wounds and trichomes (stigmatic hair), and forming biofilms on the silk surface before Fg infection; and later by colonizing and apparently killing Fg hyphae after infection. Overall, the results show that the microbiome of the female reproductive tissue of plants has the potential to defend the maternal parent and its progeny. Furthermore, this ability may have been inadvertently exploited by indigenous farmers, though this interpretation requires further evidence. Specifically, the origin of these bacteria needs investigation, in particular the extent to which they originate from seed via the maternal vascular tissue.
Data availability statement
The original contributions presented in the study are included in the article/Supplementary Material. Further inquiries can be directed to the corresponding author.
Author contributions
AS: Conceptualization, Formal Analysis, Investigation, Methodology, Validation, Visualization, Writing – original draft. VL-R: Investigation, Writing – review & editing. DB: Investigation, Writing – review & editing. MR: Conceptualization, Funding acquisition, Supervision, Writing – review & editing.
Funding
The author(s) declare financial support was received for the research, authorship, and/or publication of this article. Funding was provided by grants to MR from Grain Farmers of Ontario (054810), the Ontario Ministry of Agriculture, Food and Rural Affairs (OMAFRA 030356, 030564) and the Natural Sciences and Engineering Research Council of Canada (NSERC 401424, 401663, 400924).
Acknowledgments
We thank Prof. Jason Wallace (University of Georgia) for helpful comments on the manuscript, and Prof. Jeffrey Ross-Ibarra (University of California, Davis) for valuable discussions about the origin and nature of maize landraces. We thank Godfrey Chu, Byron Good, Sue Couling, Jacob Kaszas, Jake Gregory, Bianka Ketheeswaranathan and Eman Khalaf (University of Guelph) for assistance with plant growth and/or silk dissections. We thank Dr. Joseph Colasanti (University of Guelph) for technical advice. We thank Dr. Michaela Strueder-Kypke and Michelle Thompson (University of Guelph) for technical assistance and lab protocols, respectively, in confocal microscopy imaging. We thank CIMMYT (Mexico) and the National Plant Germplasm System (NPGS) of the U.S. Department of Agriculture for providing maize seeds.
Conflict of interest
The authors declare that the research was conducted in the absence of any commercial or financial relationships that could be construed as a potential conflict of interest.
Publisher’s note
All claims expressed in this article are solely those of the authors and do not necessarily represent those of their affiliated organizations, or those of the publisher, the editors and the reviewers. Any product that may be evaluated in this article, or claim that may be made by its manufacturer, is not guaranteed or endorsed by the publisher.
Supplementary material
The Supplementary Material for this article can be found online at: https://www.frontiersin.org/articles/10.3389/fpls.2023.1292109/full#supplementary-material
Supplementary Figure 1 | A Maximum likelihood (ML) phylogenetic tree of the entire bacterial population based on unique operational taxonomic units (OTUs) (in black letters) and all the 201 bacterial strains cultured (strains ID in green letters). Bootstrap values are indicated above the branches.
Supplementary Figure 2 | Predicted genus-level taxonomy of unpollinated silk-associated bacteria cultured from different host maize accessions spanning the Americas after growth in a common field. The predicted genera are based on full-length 16S Blastn searches at NCBI. The colours (yellow and dark green) highlight the total number of isolates (including redundant and unique sequences) belonging to the corresponding bacterial genus, arranged by host maize accession. The data is organized based on the driest to the wettest environments of source maize plants differentiated with different color codes (Dry, Moderate, and Wet) with the actual rainfall values (in mm) of the location from which the seeds were collected. The letter ‘H’ denotes a highland origin, ‘M’ denotes a mid-altitude origin, and ‘L’ denotes a lowland origin with respect to source maize plants. The latitudes and countries from where the seeds of host accessions were collected are presented. All plants were grown in Elora, Ontario, Canada.
Supplementary Figure 3 | The most prevalent cultured unpollinated silk-associated bacteria at the OTU level from diverse American maize after growth in a common field. Full-length 16S RNA sequences sharing the same genus were multi-aligned, and each unique sequence was assigned a distinct OTU number (see Methods for stringency criteria). Shown are the resulting species- and OTU-level taxonomic predictions of silk-associated bacteria that were cultured from more than one host maize accession. The corresponding species prediction is noted along with the percentage identity to the top match at NCBI in brackets. The data is organized by the rainfall environment of source maize plants with the actual rainfall value (in mm) classified as Dry, Moderate, and Wet. The letter ‘H’ denotes a highland origin, ‘M’ denotes a mid-altitude origin, and ‘L’ denotes a lowland origin with respect to source maize plants. The latitude values and the country of origin of the source maize plants are noted. The most conserved OTUs are highlighted horizontally. All plants were grown in a common field in Elora, Ontario, Canada.
Supplementary Figure 4 | Selection of safe anti-Fg bacterial strains from unpollinated silks for the replicated greenhouse trials. (A) Selection based on the initial human biosafety risk group assessments based on 16S-based phylogenetic tree reconstruction. 16S rRNA as well as whole genome sequencing were done for taxonomic identification of the unpollinated silk-associated anti-Fg bacterial strains. A BLAST search using the 16S rRNA sequences was done against the NCBI GenBank database that showed that AS112 resembled Rouxiella chamberiensis (99.34% identity match), and AS150 as Pantoea ananatis (99.41% identity match). The Gene bank accession identification for these strains are OL670733 and MW385266 respectively. The 16S rRNA-based neighbor-joining phylogenetic tree of these anti-Fg bacterial strains was constructed with these predicted taxonomies which confirmed that AS112 was closely related to Rouxiella badensis and AS150 to Pantoea ananatis. The Red arrows point to the anti-Fusarium bacterial strains used in the replicated greenhouse trials. The sequences other than the anti-Fusarium strains AS112 and AS150 were pooled from the gene bank which were closely related to these anti-Fg strains. These sequences were then aligned and evolutionary analyses were conducted in MEGA X to generate a neighbor-joining tree for all strains, with bootstrap values based on 900 replicates. (B) Selection based on clinical antibiotic disc susceptibility testing using a disc diffusion method. Anti-Fg bacterial strains were grown for two days in LB broth (pH 7.2) at 30°C, at 200 rpm. Then, a 500 µL liquid culture of each bacterial strain was spread evenly on a 150 mm X 15 mm plate containing Muller-Hilton agar. Ten antibiotic discs per plate were placed on the agar surface and pressed gently followed by incubation at 30°C for 24 h. Each antibiotic disc was tested in three different independent plates for each bacterial strain. The zones of inhibition were measured in mm and categorized as Susceptible (S): a larger ring of inhibition at >15mm diameter (green); Moderate (M): a smaller ring of inhibition (less than 15mm diameter) (yellow); Resistant (R): No ring of inhibition to the antibiotic disc used (red).
References
Abo-Elyousr, A. M. K., Sallam, M. A. A., Zeller, W. (2011). Effect of Acibenzolar-S-methyl and Rahnella aquatilis (Ra39) on fire blight of apple plants. Acta Hortic. 896, 511–518. doi: 10.17660/ACTAHORTIC.2011.896.75
Akohoue, F., Miedaner, T. (2022). Meta-analysis and co-expression analysis revealed stable QTL and candidate genes conferring resistances to Fusarium and Gibberella ear rots while reducing mycotoxin contamination in maize. Front. Plant Sci. 13. doi: 10.3389/fpls.2022.1050891
Antonovics, J. (2005). Plant venereal diseases: insights from a messy metaphor. New Phytol. 165, 71–80. doi: 10.1111/J.1469-8137.2004.01215.X
Arnason, J. T., Baum, B., Gale, J., Lambert, J. D. H., Bergvinson, D., Philogene, B. J. R., et al. (1994). Variation in resistance of Mexican landraces of maize to maize weevil Sitophilus zeamais, in relation to taxonomic and biochemical parameters. Euphytica 74, 227–236. doi: 10.1007/BF00040405
Bedoya, C. A., Dreisigacker, S., Hearne, S., Franco, J., Mir, C., Prasanna, B. M., et al. (2017). Genetic diversity and population structure of native maize populations in Latin America and the Caribbean. PloS One 12, e0173488. doi: 10.1371/journal.pone.0173488
Bonavia, D. (2013). Maize: Origin, domestication, and its role in the development of culture (Cambridge: Cambridge University Press). Available at: https://books.google.ca/books?hl=en&lr=&id=qGQgAwAAQBAJ&oi=fnd&pg=PR11&ots=HCGp0ifVm8&sig=8XSGkFZNMq_b2mWDA-uO9VufKQw&redir_esc=y#v=onepage&q&f=false (Accessed March 15, 2022).
Canadian Food Inspection Agency. (2015). RG-8 Regulatory guidance: Contaminants in feed section 1: Mycotoxins in livestock feed. Can. Food Insp. Agency, 1–29. Available at: https://inspection.canada.ca/animal-health/livestock-feeds/regulatory-guidance/rg-8/eng/1347383943203/1347384015909?chap=1#s1c1
Card, S. D., Pearson, M. N., Clover, G. R. G. (2007). Plant pathogens transmitted by pollen. Australas. Plant Pathol. 36, 455. doi: 10.1071/AP07050
Chen, F., Guo, Y. B., Wang, J. H., Li, J. Y., Wang, H. M. (2007). Biological control of grape crown gall by Rahnella aquatilis HX2. Plant Dis. 91, 957–963. doi: 10.1094/PDIS-91-8-0957
Chettoor, A. M., Phillips, A. R., Coker, C. T., Dilkes, B., Evans, M. M. S. (2016). Maternal gametophyte effects on seed development in maize. Genetics 204, 233–248. doi: 10.1534/GENETICS.116.191833/-/DC1
CONABIO. (2017) Ecosystems and agro-biodiversity across small and large-scale maize production systems, feeder study to the “TEEB for Agriculture and Food.” Available at: http://www.teebweb.org/wp-content/uploads/2018/01/Final-Maize-TEEB-report_290817.pdf%0Ahttps://www.biodiversidad.gob.mx/diversidad/proyectos/valoracion-de-maices.
Cuevas Montilla, E., Hillebrand, S., Antezana, A., Winterhalter, P. (2011). Soluble and bound phenolic compounds in different Bolivian purple corn (Zea mays L.) cultivars. J. Agric. Food Chem. 59, 7068–7074. doi: 10.1021/jf201061x
Cui, Z., Huntley, R. B., Zeng, Q., Steven, B. (2021). Temporal and spatial dynamics in the apple flower microbiome in the presence of the phytopathogen Erwinia amylovora. ISME J. 15, 318–329. doi: 10.1038/s41396-020-00784-y
Da Fonseca, R. R., Smith, B. D., Wales, N., Cappellini, E., Skoglund, P., Fumagalli, M., et al. (2015). The origin and evolution of maize in the southwestern United States. Nat. Plants 1, 14003. doi: 10.1038/nplants.2014.3
Deroo, W., De Troyer, L., Dumoulin, F., De Saeger, S., De Boevre, M., Vandenabeele, S., et al. (2022). A novel in planta enrichment method employing Fusarium graminearum-infected wheat spikes to select for competitive biocontrol bacteria. Toxins (Basel). 14, 222. doi: 10.3390/TOXINS14030222/S1
Dimkić, I., Janakiev, T., Petrović, M., Degrassi, G., Fira, D. (2022). Plant-associated Bacillus and Pseudomonas antimicrobial activities in plant disease suppression via biological control mechanisms - A review. Physiol. Mol. Plant Pathol. 117, 101754. doi: 10.1016/J.PMPP.2021.101754
Diniz, G. F. D., Cota, L. V., Figueiredo, J. E. F., Aguiar, F. M., da Silva, D. D., de Paula Lana, U. G., et al. (2022a). Antifungal activity of bacterial strains from maize silks against Fusarium verticillioides. Arch. Microbiol. 204, 89. doi: 10.1007/S00203-021-02726-4
Diniz, G. F. D., Figueiredo, J. E. F., Lana, U. G. P., Marins, M. S., Silva, D. D., Cota, L. V., et al. (2022b). Microorganisms from corn stigma with biocontrol potential of Fusarium verticillioides. Braz. J. Biol. 82, e262567. doi: 10.1590/1519-6984.262567
Dresselhaus, T., Lausser, A., Márton, M. L. (2011). Using maize as a model to study pollen tube growth and guidance, cross-incompatibility and sperm delivery in grasses. Ann. Bot. 108, 727–737. doi: 10.1093/aob/mcr017
Dresselhaus, T., Sprunck, S., Wessel, G. M. (2016). Fertilization mechanisms in flowering plants. Curr. Biol. 26, R125–R139. doi: 10.1016/j.cub.2015.12.032
Drews, G. N., Koltunow, A. M. (2011). The female gametophyte. Arabidopsis Book 9, e0155. doi: 10.1199/TAB.0155
Feng, T., Liu, Y. (2022). Microorganisms in the reproductive system and probiotic’s regulatory effects on reproductive health. Comput. Struct. Biotechnol. J. 20, 1541–1553. doi: 10.1016/J.CSBJ.2022.03.017
Fernando Amezquita-Aviles, C., Brizeida Coronel-Acosta, C., de los Santos-Villalobos, S., Santoyo, G., Isela Parra-Cota, F. (2022). Characterization of native plant growth-promoting bacteria (PGPB) and their effect on the development of maize (Zea mays L.). Biotechnia 1, 15–22. doi: 10.18633/biotecnia.v24i1.1353
Fléche-Matéos, A., Kügler, J. H., Hansen, S. H., Syldatk, C., Hausmann, R., Lomprez, F., et al. (2017). Rouxiella badensis sp. nov. and Rouxiella silvae sp. nov. isolated from peat bog soil and emendation description of the genus Rouxiella. Int. J. Syst. Evol. Microbiol. 67, 1255–1259. doi: 10.1099/IJSEM.0.001794/CITE/REFWORKS
Gaff, D. F., Okong’O-Ogola, O. (1971). The use of non-permeating pigments for testing the survival of cells. J. Exp. Bot. 22, 756–758. doi: 10.1093/jxb/22.3.756
Galloway, L. F. (2005). Maternal effects provide phenotypic adaptation to local environmental conditions. New Phytol. 166, 93–100. doi: 10.1111/j.1469-8137.2004.01314.x
Ghazy, N., El-Nahrawy, S. (2021). Siderophore production by Bacillus subtilis MF497446 and Pseudomonas koreensis MG209738 and their efficacy in controlling Cephalosporium maydis in maize plant. Arch. Microbiol. 203, 1195–1209. doi: 10.1007/s00203-020-02113-5
Goodman, M. M., Brown, W. L. (1988). “Races of corn,” In Corn and Corn Improvement, 3rd ed. John Wiley & Sons, Ltd: New York, NY, USA 18, 33–79. doi: 10.2134/agronmonogr18.3ed.c2
Grobman, A., Salhuana, W., Sevilla, R. (1961). Races of maize in Peru: Their origins, evolution and classification. (Washington DC: National Academy of Sciences-National Research Council).
Guo, J., Liu, T., Han, L., Liu, Y. (2009). The effects of corn silk on glycaemic metabolism. Nutr. Metab. 6, 47. doi: 10.1186/1743-7075-6-47
Hatheway, W. H. (1957). Race of maize in Cuba (Washington D.C: National Academy of Sciences-National Research Council).
Hazzard, R. V., Schultz, B. B., Groden, E., Ngollo, E. D., Seidlecki, E. (2003). Evaluation of oils and microbial pathogens for control of Lepidopteran pests of sweet corn in New England. J. Econ. Entomol. 96, 1653–1661. doi: 10.1093/JEE/96.6.1653
Heslop-Harrison, Y., Heslop-Harrison, J., Reger, B. J. (1985). The pollen-stigma interaction in the grasses. 7. Pollen-tube guidance and the regulation of tube number in Zea mays L. Acta Bot. Neerl. 34, 193–211. doi: 10.1111/J.1438-8677.1985.TB01879.X
Hultberg, M., Alsberg, T., Khalil, S., Alsanius, B. (2010a). Suppression of disease in tomato infected by Pythium ultimum with a biosurfactant produced by Pseudomonas koreensis. BioControl 55, 435–444. doi: 10.1007/S10526-009-9261-6
Hultberg, M., Bengtsson, T., Liljeroth, E. (2010b). Late blight on potato is suppressed by the biosurfactant-producing strain Pseudomonas koreensis 2.74 and its biosurfactant. BioControl 55, 543–550. doi: 10.1007/S10526-010-9289-7
Jiang, L., Jeong, J. C., Lee, J. S., Park, J. M., Yang, J. W., Lee, M. H., et al. (2019). Potential of Pantoea dispersa as an effective biocontrol agent for black rot in sweet potato. Sci. Rep. 9, 16354. doi: 10.1038/s41598-019-52804-3
Jimenez-Quiros, C., Okechukwu, E. C., Hong, Y., Baysal, Ö., Tör, M. (2022). Comparison of antifungal activity of Bacillus strains against Fusarium graminearum In vitro and In planta. Plants 11, 1999. doi: 10.3390/plants11151999
Johnson, K. B., Stockwell, V. O., McKaughlin, R. J., Sugar, D., Loper, J. E., Roberts, R. G. (1993). Effect of antagonistic bacteria on establishment of honey bee-dispersed Erwinia amylovora in pear blossoms and on fire blight control. Phytopathology 83, 995–1002. doi: 10.1094/Phyto-83-995
Johnston-Monje, D., Raizada, M. N. (2011). Conservation and diversity of seed associated endophytes in Zea across boundaries of evolution, ethnography and ecology. PloS One 6, e20396. doi: 10.1371/journal.pone.0020396
Junker, R. R., Keller, A. (2015). Microhabitat heterogeneity across leaves and flower organs promotes bacterial diversity. FEMS Microbiol. Ecol. 91, 97. doi: 10.1093/FEMSEC/FIV097
Kačániová, M., Miroslav, J., Chlebo, R., Kňazovická, V., Kadasi-Horáková, M., Kunová, S., et al. (2011). Mycobiota and mycotoxins in bee pollen collected from different areas of Slovakia. J. Environ. Sci. Heal. - Part B Pestic. Food Contam. Agric. Wastes 46, 623–629. doi: 10.1080/03601234.2011.589322
Kearns, L. (1993). Biological control of Erwinia amylovora by Erwinia herbicola. (New Zeal: Univ. Canterbury).
Khalaf, E. M., Shrestha, A., Rinne, J., Lynch, M. D. J., Shearer, C. R., Limay-Rios, V., et al. (2021). Transmitting silks of maize have a complex and dynamic microbiome. Sci. Rep. 11, 13215. doi: 10.1038/s41598-021-92648-4
Konkin, D., Hsueh, Y.-C., Kirzinger, M., Kubaláková, M., Haldar, A., Balcerzak, M., et al. (2022). Genomic sequencing of Thinopyrum elongatum chromosome arm 7EL, carrying Fusarium head blight resistance, and characterization of its impact on the transcriptome of the introgressed line CS-7EL. BMC Genomics 23, 228. doi: 10.1186/S12864-022-08433-8
Kostić, A., Milinčić, D. D., Petrović, T. S., Krnjaja, V. S., Stanojević, S. P., Barać, M. B., et al. (2019). Mycotoxins and mycotoxin producing fungi in pollen: Review. Toxins (Basel). 11, 64. doi: 10.3390/toxins11020064
Kroh, M., Gorissen, M. H., Pfahler, P. L. (1979). Ultrastructural studies on styles and pollen tubes of Zea mays L. General survey on pollen tube growth in vivo. Acta Bot. Neerl. 28, 513–518. doi: 10.1111/J.1438-8677.1979.TB01176.X
Langner, J. A., Zanon, A. J., Streck, N. A., Reiniger, L. R. S., Kaufmann, M. P., Alves, A. F. (2019). Maize: Key agricultural crop in food security and sovereignty in a future with water scarcity. Rev. Bras. Eng. Agrícola e Ambient. 23, 648–654. doi: 10.1590/1807-1929/AGRIAMBI.V23N9P648-654
Limay-Rios, V., Schaafsma, A. W. (2021). Relationship between mycotoxin content in winter wheat grain and aspirated dust collected during harvest and after storage. ACS Omega 6, 1857–1871. doi: 10.1021/acsomega.0c04256
Lindow, S. E., McGourty, G., Elkins, R. (1996). Interactions of antibiotics with Pseudomonas fluorescens strain A506 in the control of fire blight and frost injury to pear. Phytopathology 86, 841–848. doi: 10.1094/Phyto-86-841
Mahmoud, A. F. (2016). Genetic variation and biological control of Fusarium graminearum isolated from wheat in Assiut-Egypt. Plant Pathol. J. 32, 145. doi: 10.5423/PPJ.OA.09.2015.0201
Manirajan, B. A., Maisinger, C., Ratering, S., Rusch, V., Schwiertz, A., Cardinale, M., et al. (2018). Diversity, specificity, co-occurrence and hub taxa of the bacterial-fungal pollen microbiome. FEMS Microbiol. Ecol. 94, 8. doi: 10.1093/femsec/fiy112
Matsuoka, Y., Vigouroux, Y., Goodman, M. M., Sanchez, J. G., Buckler, E., Doebley, J. (2002). A single domestication for maize shown by multilocus microsatellite genotyping. Proc. Natl. Acad. Sci. U.S.A. 99, 6080–6084. doi: 10.1073/pnas.052125199
McCormick, S., Stanley, A. M., Stover, N. A., Alexander, N. J. (2011). Trichothecenes: From simple to complex mycotoxins. Toxins (Basel) 3, 802–814.
McFrederick, Q. S., Rehan, S. M. (2019). Wild bee pollen usage and microbial communities co-vary across landscapes. Microb. Ecol. 77, 513–522. doi: 10.1007/s00248-018-1232-y
Megías, E., Megías, M., Ollero, F. J., Hungria, M. (2016). Draft genome sequence of Pantoea ananatis strain AMG521, a rice plant growth-promoting bacterial endophyte isolated from the Guadalquivir marshes in southern Spain. Genome Announc. 4, 1681–1696. doi: 10.1128/GENOMEA.01681-15
Megías, E., Reis Junior, F. B., Ribeiro, R. A., Megías, M., Ollero, F. J., Hungria, M. (2017). Genome sequence of Pantoea sp. strain 1.19, isolated from rice rhizosphere, with the capacity to promote growth of legumes and nonlegumes. Genome Announc. 5, e00707–e00717. doi: 10.1128/GENOMEA.00707-17
Miller, J. D. (2001). Factors that affect the occurrence of fumonisin. Environ. Health Perspect. 109, 321–324. doi: 10.1289/EHP.01109S2321
Miller, S. S., Chabot, D. M. P., Ouellet, T., Harris, L. J., Fedak, G. (2004). Use of a Fusarium graminearum strain transformed with green fluorescent protein to study infection in wheat (Triticum aestivum). Can. J. Plant Pathol. 26, 453–463. doi: 10.1080/07060660409507165
Miller, S. S., Reid, L. M., Harris, L. J. (2007). Colonization of maize silks by Fusarium graminearum, the causative organism of Gibberella ear rot. Can. J. Bot. 85, 369–376. doi: 10.1139/B07-027
Morales-Cedeño, L. R., de los Santos-Villalobos, S., Santoyo, G. (2021). Functional and genomic analysis of Rouxiella badensis SER3 as a novel biocontrol agent of fungal pathogens. Front. Microbiol. 12. doi: 10.3389/FMICB.2021.709855/BIBTEX
Mousa, W. K., Shearer, C., Limay-rios, V., Ettinger, C. L., Eisen, J. A., Raizada, M. N. (2016). Root-hair endophyte stacking in finger millet creates a physicochemical barrier to trap the fungal pathogen Fusarium graminearum. Nat. Microbiol. 1, 16167. doi: 10.1038/nmicrobiol.2016.167
Mousa, W. K., Shearer, C. R., Limay-Rios, V., Zhou, T., Raizada, M. N. (2015). Bacterial endophytes from wild maize suppress Fusarium graminearum in modern maize and inhibit mycotoxin accumulation. Front. Plant Sci. 6. doi: 10.3389/fpls.2015.00805
Mudge, A. M., Dill-Macky, R., Dong, Y., Gardiner, D. M., White, R. G., Manners, J. M. (2006). A role for the mycotoxin deoxynivalenol in stem colonisation during crown rot disease of wheat caused by Fusarium graminearum and Fusarium pseudograminearum. Physiol. Mol. Plant Pathol. 69, 73–85. doi: 10.1016/j.pmpp.2007.01.003
Munkvold, G. P. (2003). Epidemiology of Fusarium diseases and their mycotoxins in maize ears. Eur. J. Plant Pathol. 109, 705–713. doi: 10.1023/A:1026078324268
Navarta, L. G., Calvo, J., Calvente, V., Benuzzi, D., Sanz, M. I. (2011). Freezing and freeze-drying of the bacterium Rahnella aquatilis BNM 0523: study of protecting agents, rehydration media and freezing temperatures. Lett. Appl. Microbiol. 53, 565–571. doi: 10.1111/J.1472-765X.2011.03150.X
Nguyen, C. D., Chen, J., Clark, D., Perez, H., Huo, H. A. (2021). Effects of maternal environment on seed germination and seedling vigor of petunia × hybrida under different abiotic stresses. Plants 10, 581. doi: 10.3390/plants10030581
Nuclo, R. L., Johnson, K. B., Stockwell, V. O., Sugar, D. (1998). Secondary colonization of pear blossoms by two bacterial antagonists of the fire blight pathogen. Plant Dis. 82, 661–668. doi: 10.1094/PDIS.1998.82.6.661
Obersteiner, A., Gilles, S., Frank, U., Beck, I., Häring, F., Ernst, D., et al. (2016). Pollen-associated microbiome correlates with pollution parameters and the allergenicity of pollen. PloS One 11, e0149545. doi: 10.1371/journal.pone.0149545
Odjo, S., Alakonya, A. E., Rosales-Nolasco, A., Molina, A. L., Muñoz, C., Palacios-Rojas, N. (2022). Occurrence and postharvest strategies to help mitigate aflatoxins and fumonisins in maize and their co-exposure to consumers in Mexico and Central America. Food Control 138, 108968. doi: 10.1016/J.foodcont.2022.108968
Ortega-Beltran, A., Guerrero-Herrera, M. D. J., Ortega-Corona, A., Vidal-Martinez, V. A., Cotty, P. J. (2014). Susceptibility to aflatoxin contamination among maize landraces from Mexico. J. Food Prot. 77, 1554–1562. doi: 10.4315/0362-028X.JFP-13-474
Oteros, J., Bartusel, E., Alessandrini, F., Núñez, A., Moreno, D. A., Behrendt, H., et al. (2018). Artemisia pollen is the main vector for airborne endotoxin. J. Allergy Clin. Immunol. 143, P369–377.E5. doi: 10.1016/j.jaci.2018.05.040
Perez-Limó, S., Li, M., Cintora-Martinez, G. C., Rocio Aguilar-Rangel, M., Nancy Salazar-Vidal, M., Gonzá Lez-Segovia, E., et al. (2022). A B73 X Palomero Toluqueno mapping population reveals local adaptation in Mexican highland maize. Genes|Genomes|Genetics 12, jkab447. doi: 10.1093/g3journal/jkab447
Pfordt, A., Romero, L. R., Schiwek, S., Karlovsky, P., von Tiedemann, A. (2020). Impact of environmental conditions and agronomic practices on the prevalence of Fusarium species associated with ear- and stalk rot in maize. Pathogens 9, 236. doi: 10.3390/PATHOGENS9030236
Prasanna, B. M. (2012). Diversity in global maize germplasm: Characterization and utilization. J. Biosci. 37, 843–855. doi: 10.1007/s12038-012-9227-1
Preece, C., Peñuelas, J. (2020). A return to the wild: Root exudates and food security. Trends Plant Sci. 25, 14–21. doi: 10.1016/j.tplants.2019.09.010
Pskowski, M. (2019) Indigenous maize: Who owns the rights to Mexico’s “wonder” plant? Available at: https://e360.yale.edu/features/indigenous-maize-who-owns-the-rights-to-Mexicos-wonder-plant.
Rahman, N. A., Wan Rosli, W. I. (2014). Nutritional compositions and antioxidative capacity of the silk obtained from immature and mature corn. J. King Saud Univ. Sci. 26, 119–127. doi: 10.1016/J.JKSUS.2013.11.002
Raj, J., Nicholls, C., Vasiljevic, M. (2021) Multiple mycotoxins detected in corn, soya and sorghum samples from Argentina, Chile, Bolivia and Colombia in 2021. Available at: https://mycotoxinsite.com/multiple-mycotoxins-detected-corn-soya-sorghum-samples-Bolivia-columbia-Argentina-Chile/?lang=en.
Rana, K. L., Kour, D., Kaur, T., Devi, R., Yadav, A., Yadav, A. N. (2021). Bioprospecting of endophytic bacteria from the Indian himalayas and their role in plant growth promotion of maize (Zea mays L.). J. Appl. Biol. Biotechnol. 9, 41–50. doi: 10.7324/JABB.2021.9306
Rebolleda Gómez, M., Ashman, T.-L. (2019). Floral organs act as environmental filters and interact with pollinators to structure the yellow monkeyflower (Mimulus guttatus) floral microbiome. Mol. Ecol. 28, 5155–5171. doi: 10.1101/721647
Reid, L. M., Bolton, A. T., Hamilton, R. I., Woldemariam, T., Mather, D. E. (1992). Effect of silk age on resistance of maize to Fusarium graminearum. Can. J. Plant Pathol. 14, 293–298. doi: 10.1080/07060669209500867
Reid, L. M., Hamilton, R. I., Mather, D. E. (1996a). Screening maize for resistance to Gibberella ear rot : Technical Bulletin (Ottawa, Ontario, Canada:Research Branch, Agriculture and Agri-Food Canada). Available at: https://archive.org/details/screeningmaizefo19965reid
Reid, L. M., Stewart, D., Hamilton, R. I. (1996b). A 4-year study of the association between Gibberella ear rot severity and deoxynivalenol concentration. J. Phytopathol. 144, 431–436. doi: 10.1111/j.1439-0434.1996.tb00319.x
Ricardo, R. E., Timothy, D. H., Grant, U. J. (1960). Races of maize in Bolivia. (Washington D.C: National Academy of Sciences-National Research Council). Available at: http://www.ars.usda.gov/sp2UserFiles/Place/50301000/Races_of_Maize/RoM_Peru_0_Book.pdf.
Rice, E. (2007). Conservation in a changing world: In situ conservation of the giant maize of Jala. Genet. Resour. Crop Evol. 54, 701–713. doi: 10.1007/s10722-006-0023-3
Rijavec, T., Lapanje, A., Dermastia, M., Rupnik, M. (2007). Isolation of bacterial endophytes from germinated maize kernels. Can. J. Microbiol. 53, 802–808. doi: 10.1139/W07-048/ASSET/IMAGES/LARGE/W07-048F1.JPEG
Robert, H. S., Park, C., Gutièrrez, C. L., Wójcikowska, B., Pěnčík, A., Novák, O., et al. (2018). Maternal auxin supply contributes to early embryo patterning in Arabidopsis. Nat. Plants 4, 548–553. doi: 10.1038/s41477-018-0204-z
Roca-Couso, R., David Flores-Félix, J., Rivas, R. (2021). Mechanisms of action of microbial biocontrol agents against Botrytis cinerea. J. Fungi 7, 1045. doi: 10.3390/jof7121045
Rocío Aguilar-Rangel, M. (2018). The role of introgression from teosinte (Zea mays ssp. mexicana) in the adaptation of maize to the highlands of Mexico, PhD Thesis, Department of Plant Biotechnology, Centro de Investigaciones y de Estudios Avanzados del Instituto Politécnico Nacional, Irapuato Unit.
Rodrigo Mendoza, J., Reen Kok, C., Stratton, J., eia Bianchini, A., Hallen-Adams, H. E. (2017). Understanding the mycobiota of maize from the highlands of Guatemala, and implications for maize quality and safety. Crop Prot. 101, 5–11. doi: 10.1016/j.cropro.2017.07.009
Ruiz Corral, J. A., Durán Puga, N., Sánchez González, J. D. J., Ron Parra, J., González Eguiarte, D. R., Holland, J. B., et al. (2008). Climatic adaptation and ecological descriptors of 42 Mexican maize races. Crop Sci. 48, 1502–1512. doi: 10.2135/CROPSCI2007.09.0518
Sancho, A. M., Moschini, R. C., Filippini, S., Rojas, D., Ricca, A. (2017). Weather-based logistic models to estimate total fumonisin levels in maize kernels at export terminals in Argentina. Trop. Plant Pathol. 43, 99–108. doi: 10.1007/s40858-017-0199-4
Santillán-Fernández, A., Salinas-Moreno, Y., Valdez-Lazalde, J. R., Bautista-Ortega, J., Pereira-Lorenzo, S. (2021). Spatial delimitation of genetic diversity of native maize and its relationship with ethnic groups in Mexico. Agronomy 11, 672. doi: 10.3390/AGRONOMY11040672
Shiomi, H. F., de Melo, I. S., de Almeida Minhoni, M. T. (2015). Assessing of endophytic bacteria for biological control of northern corn leaf blight (Exserohilum turcicum). Arq. Inst. Biol. (Sao. Paulo). 82, 00210. doi: 10.1590/1808-1657000642013
Shiomi, H. F., Soares De Melo, I., Teixeira, M., Minhoni, A. (2008). Selection of endophytic bacteria with antagonistic action against plant pathogens. Sci. Agrar. 9, 535–538.
Shovitri, M., Sugianto, S. K., Kuswytasari, N. D., Alami, N., Zulaika, E. (2022). Application of rhizobacteria and NPK for growth and productivity of sweet corn (Zea mays L.). Adv. Biol. Sci. Res. 22, 111–117. doi: 10.2991/absr.k.220406.017
Someya, N., Numata, S., Nakajima Akira Hasebe, M., Hibi, T., Akutsu, K., Someya, N., et al. (2003). Biological control of rice blast by the epiphytic bacterium Erwinia ananas transformed with a chitinolytic enzyme gene from an antagonistic bacterium, Serratia marcescens strain B2. J. Gen. Plant Pathol. 69, 276–282. doi: 10.1007/S10327-003-0043-1
Stockwell, V. O., McLaughlin, R. J., Henkels, M. D., Loper, J. E., Sugar, D., Roberts, R. G. (1999). Epiphytic colonization of Pear stigmas and hypanthia by bacteria during primary bloom. Phytopathology. 89, 1162–1168. doi: 10.1094/PHYTO.1999.89.12.1162
Suman, A., Shukla, L., Marag, P. S., Verma, P., Gond, S., Prasad, J. S. (2020). Potential use of plant colonizing Pantoea as generic plant growth promoting bacteria for cereal crops. Artic. J. Environ. Biol. 41, 987–994. doi: 10.22438/jeb/41/5/MRN-1250
Sun, X., Ma, W., Xu, Y., Jin, X., Ni, H. (2020). Complete genome sequence of Rahnella aquatilis MEM40, a plant growth-promoting rhizobacterium isolated from rice rhizosphere soil, with antagonism against Magnaporthe oryzae and Fusarium graminearum. Microbiol. Resour. Announc. 9, e00651–e00620. doi: 10.1128/MRA.00651-20
Sutton, J. C. (1982). Epidemiology of wheat head blight and maize ear rot caused by Fusarium graminearum. Can. J. Plant Pathol. 4, 195–209. doi: 10.1080/07060668209501326
Swamy, M., Naik, N. M., Yallappa, M., Ramesh, YM., Yenherappa, ST. (2022). Biocontrol potentiality of bacterial endophytes against maize diseases. Pharma Innov. J. 11, 111–113.
Taba, S. (1995). Maize genetic resources. Maize Program Special Report (Mexico). Available at: https://books.google.ca/books?hl=en&lr&id=yn0RKKnSiMgC&oi=fnd&pg=PA7&dq=Nal-Tel,+an+ancient+maize+landraces&ots=OWmuSCSUOi&sig=aMaNn1MKN3J9CJSordtdfZShfZo&pli=1#v=onepage&q&f=false (Accessed March 10, 2022).
Tejaswini (2002). Variability of pollen grain features: A plant strategy to maximize reproductive fitness in two species of Dianthus? Sex Plant Reprod. 14, 347–353. doi: 10.1007/s00497-002-0130-z
Thapa, A., Horgan, K. A., White, B., Walls, D. (2021). Deoxynivalenol and zearalenone—Synergistic or antagonistic agri-food chain co-contaminants? Toxins (Basel). 13, 561. doi: 10.3390/TOXINS13080561
Thompson, M. E. H., Raizada, M. N. (2018). Fungal pathogens of maize gaining free passage along the silk road. Pathogens 7, 81. doi: 10.3390/pathogens7040081
Thompson, M. E. H., Raizada, M. N. (2023). Protocols to enable fluorescence microscopy of microbial interactions on living silks (style tissue) that balance the requirements for containment, biological relevance, viability, and experimental accessibility. bioRxiv 564993. doi: 10.1101/2023.10.31.564993
Turrent, A., Serratos, J. A. (2004). “Context and background on maize and its wild relatives in Mexico,” in Maize and Biodiversity: The Effects of Transgenic Maize in Mexico. Eds. Sarukhan, P., Raven, J. (Oaxaca:Secretariat of the Commission for Environmental Cooperation of North America), 1–56. Available at: http://www.cec.org/sites/default/files/related_documents/maize_biodiversity/4534_Maize-Biodiversity-Chapter1_en.pdf.
Vigouroux, Y., Glaubitz, J. C., Matsuoka, Y., Goodman, M. M., Sanchez G., J., Doebley, J. (2008). Population structure and genetic diversity of New World maize races assessed by DNA microsatellites. Am. J. Bot. 95, 1240–1253. doi: 10.3732/ajb.0800097
Warburton, M. L., Wilkes, G., Taba, S., Charcosset, A., Mir, C., Dumas, F., et al. (2011). Gene flow among different teosinte taxa and into the domesticated maize gene pool. Genet. Resour Crop Evol. 58, 1243–1261. doi: 10.1007/s10722-010-9658-1
Wellhausen, E. (1952). Races of maize in Mexico their origin, characteristics and distribution. (Jamaica Plain: Bussey Institution of Harvard University).
Wilson, M., Epton, H. A. S., Sigee, D. C. (1992). Interactions between Erwinia herbicola and E. amylovora on the stigma of Hawthorn blossoms. Phytopathology 82, 914–918. doi: 10.1094/Phyto-82-914
Wilson, M., Lindow, S. E. (1992). Interactions between the biological control agent Pseudomonas fluorescens A506 and Erwinia amylovora in pear blossoms. Phytopathology 83, 117–123. doi: 10.1094/Phyto-83-117
Yadegari, R., Drews, G. N. (2004). Female gametophyte development. Plant Cell 16, S133–S141. doi: 10.1105/tpc.018192
Yahfoufi, N., Alsadi, N., Mallet, J. F., Kulshreshtha, G., Hincke, M., Ismail, N., et al. (2021). Immunomodulation and intestinal morpho-functional aspects of a novel gram-negative bacterium Rouxiella badensis subsp. acadiensis. Front. Microbiol. 12. doi: 10.3389/fmicb.2021.569119
Yokoya, K., Postel, S., Fang, R., Sarasan, V. (2017). Endophytic fungal diversity of Fragaria vesca, a crop wild relative of strawberry,along environmental gradients within a small geographical area. PeerJ 5, e2860. doi: 10.7717/peerj.2860
Zasloff, M. (2017). Pollen has a microbiome: implications for plant reproduction, insect pollination and human allergies. Environ. Microbiol. 19, 1–2. doi: 10.1111/1462-2920.13661
Zboralski, A., Biessy, A., Filion, M. (2022). Bridging the gap: Type III secretion systems in plant-beneficial bacteria. Microorganisms 10, 187. doi: 10.3390/microorganisms10010187
Keywords: maize, silk, style, microbiome, Fusarium, mycotoxin, Gibberella ear rot, biocontrol
Citation: Shrestha A, Limay-Rios V, Brettingham DJL and Raizada MN (2023) Bacteria existing in pre-pollinated styles (silks) can defend the exposed male gamete fertilization channel of maize against an environmental Fusarium pathogen. Front. Plant Sci. 14:1292109. doi: 10.3389/fpls.2023.1292109
Received: 11 September 2023; Accepted: 09 November 2023;
Published: 04 December 2023.
Edited by:
Raffaella Balestrini, National Research Council (CNR), ItalyCopyright © 2023 Shrestha, Limay-Rios, Brettingham and Raizada. This is an open-access article distributed under the terms of the Creative Commons Attribution License (CC BY). The use, distribution or reproduction in other forums is permitted, provided the original author(s) and the copyright owner(s) are credited and that the original publication in this journal is cited, in accordance with accepted academic practice. No use, distribution or reproduction is permitted which does not comply with these terms.
*Correspondence: Manish N. Raizada, raizada@uoguelph.ca