- 1 Department of Cognition, Perception and Brain Sciences, University College London, London, UK
- 2 CoMPLEX, University College London, London, UK
Traditionally time perception has been considered the product of a central, generic, cognitive mechanism. However, evidence is emerging for a distributive system with modality-specific sensory components (Morrone et al., 2005; Johnston et al., 2006). Here we show that fast contrast adaptation, which can be observed in the retina, induces a change in apparent duration. The perceived duration of a subsecond interval containing a 50% luminance contrast drifting pattern is compressed when it follows a high (90%) as compared to a low (10%) contrast interval. The duration effect cannot be attributed to changes in latency at onset relative to offset, can be dissociated from the effect of contrast context on apparent speed or apparent contrast per se and it occurs in a retinocentric frame of reference. The temporal compression is limited to high drift temporal frequencies (≥10 Hz) and is not observed for equiluminant chromatic stimuli. This pattern of results indicates a major role for the magnocellular pathway in the neural encoding and representation of visual time.
Introduction
Recently, an increasing number of studies, targeting the subsecond range (Morrone et al., 2005; Johnston et al., 2006; Kanai et al., 2006; Burr et al., 2007; Terao et al., 2008) have shown that purely visual manipulations can alter the apparent duration of a stimulus. Temporal (Morrone et al., 2005) as well as spatial (Ross et al., 1997) distortions, appearing around the time of onset of a saccadic eye movement, have been associated with predictive spatial shifts in lateral intraparietal (LIP) receptive fields. In contradistinction, spatially localized duration compression induced by adaptation for several seconds to high frequency drift or flicker has been attributed to changes in subcortical regions of the magnocellular pathway (Johnston et al., 2006, 2008; Ayhan et al., 2009), and specifically to a sharpening of temporal tuning. Some neurons in the Wallaby nucleus of the optic tract (NOT) show a sharpening of the temporal impulse response after high temporal frequency adaptation (Ibbotson, 2005) and those that do also show contrast gain control (Clifford et al., 1997; Ibbotson, 2005).
In primates and man, adaptation to high temporal frequencies is likely to be mediated by the magnocellular pathway. M cells have higher contrast sensitivity and are tuned to higher temporal frequencies than P cells (Derrington and Lennie, 1984). They are also more liable to adapt to stimulus contrast. A slow adaptation phase in which adaptation to high contrast patterns at high temporal frequencies selectively reduces the contrast sensitivity of lateral geniculate nucleus (LGN) M cells has been reported (Solomon et al., 2004; Duong and Freeman, 2007). This slow adaptation in the LGN is not spatial frequency specific (Duong and Freeman, 2007). Benardete and Kaplan (1999) report that there is also a very fast adaptation phase referred to as “contrast gain control” (Shapley and Victor, 1978; Mante et al., 2008) in primate ganglion cells, which consists of a decrease of contrast gain at low temporal frequencies delivering a more bandpass temporal frequency response (Figure 2 of Benardete and Kaplan, 1999). This occurs in M cells but not in P cells. In the time domain, this means that with increased contrast, the temporal impulse response function of an M cell shortens and advances (i.e., the peak of the function occurs progressively earlier when contrast is increased, Figure 5 of Benardete and Kaplan, 1999).
If changes in perceived duration are mediated by the magnocellular system then we should expect to see effects of fast adaptation to contrast as well as slow adaptation at high temporal frequencies. Our group has previously shown that slow adaptation to 20 Hz flicker or drifting motion induces duration compression (Johnston et al., 2006, 2008; Ayhan et al., 2009; Bruno et al., 2010). To test whether fast adaptation has also an effect on perceived duration, in the present study, we investigated the influence of luminance contrast context on the apparent duration of a visual stimulus. We showed a reduction of apparent duration when an intermediate contrast test interval was briefly preceded by a high contrast context as compared to when preceded by a low contrast context. This time compression does not seem to depend on the perceived temporal frequency of the stimulus or on it’s perceived contrast and it is observed only for dynamic stimuli moving at high temporal frequencies (≥10 Hz). Since the effects of contrast on the temporal tuning of M cells occur at an early stage in the visual system, we also included a procedure in which the stimuli changed position relative to the head but not to the eye, thus providing a purely retinotopic stimulation. Under these conditions we observed the same pattern of results found during fixation. These results, together with the observation that chromatic contrast context was found to have no influence on apparent duration, suggest that changes in perceived duration might be related to modifications in the shape of the temporal impulse response function induced by gain-control mechanisms.
Materials and Methods
Apparatus
Stimuli were displayed, in a darkened room, on a 19-inch Sony Trinitron Multiscan 400PS, with a refresh rate of 100 Hz, driven by a VSG2/5 visual stimulus generator (Cambridge Research Systems). Stimuli were viewed from a distance of 57 cm.
Stimuli and Procedure
Perceived duration and temporal order
In the first experiment (Figure 1A) we measured apparent duration of a 50% contrast interval following either a 90% or 10% contrast inducer. Subjects were asked to foveate a central fixation spot and attend to a dynamic sequence in the near periphery. They were asked to compare the relative duration of two luminance-modulated sinusoidal gratings (spatial frequency 1 c/degree; Michelson contrast in percentage 50%) drifting in opposite directions that were presented within a five interval sequence. The two test intervals were interleaved with three 1500-ms intervals of oscillating motion whose direction changed every 250 ms. In one sequence the contrast of the first and last interval was set to 90% and the middle interval to 10%, in a second sequence this relationship was reversed to control for order effects. The choice of sequence was fixed within a session. This arrangement ensured the test intervals were always bounded by a transition in contrast. The interval transition was also defined by orientation. The gratings (−45° with respect to vertical) in the test intervals were orthogonal to those in the background intervals (45°). To ensure there was no build up of slow adaptation, stimuli (diameter: 5° of visual angle) were randomly displayed across trials in one of eight possible spatial positions in a notional circular aperture (midpoint 5° from the center). The duration of one of the two test intervals (the standard) was fixed (500 ms), while the other interval (the comparison) was varied over trials between 250 and 1000 ms to generate a psychometric function. We randomly interleaved trials with the standard in the first or second interval within a run so that subjects could not ignore the standard. Subjects had to report which of the two test intervals contained the longer stimulus. The 50% point on the psychometric function (the psychometric data from each condition were fitted with a cumulative Gaussian) provided a measure of the perceived duration of the comparison required to match the duration of the standard. The plotted points are the difference between perceived duration of the interval following high contrast and the interval following low contrast collapsed over type of sequence and position of the standard in the sequence. Specifically for each contrast context, we calculated the shift in perceived duration of the first 50% contrast test interval in the sequence relative to the second 50% contrast test interval. We then determined the difference between the shift obtained in the 90%–10%–90% contrast context and the shift obtained in the 10%–90%–10% contrast context. This difference (divided by two sequences) describes the average effect of the perceived duration of an intermediate contrast interval when it is preceded by a high contrast background interval as compared to when it is preceded by a low contrast background interval, canceling any difference in duration due to order in the sequence. A negative value indicates a compression of apparent duration after high contrast relative to low contrast. Perceived duration was measured for a range of speeds (2, 5, 10, 20 Hz; °/s) and inducer and test gratings had the same speed in each condition.
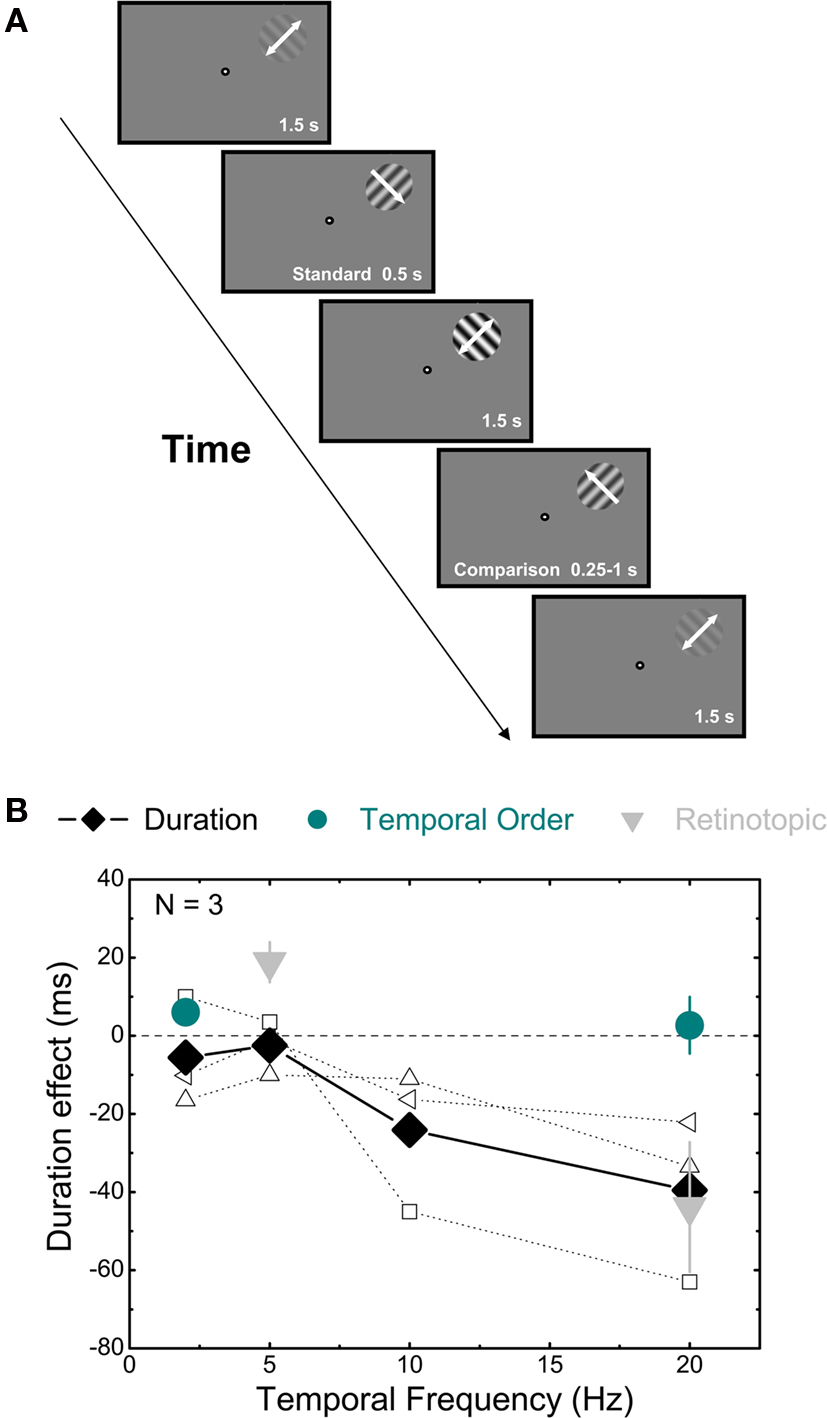
Figure 1. Effect of luminance contrast on duration judgments. (A) Schematic illustration of the time course of the binary choice experiment, in which subjects were required to judge the relative duration of two test intervals containing a 50% contrast grating embedded, in separate sessions, in two different contrast contexts: in the first one (shown in the figure), the contrast of the first and last background intervals was 10%, while the contrast of the intervening interval was 90%; in the second condition (not shown), the contrast of the first and last interval was 90% and in the intervening one it was 10%. (B) Results of the luminance contrast, the onset/offset and the pursuit experiments averaged across three subjects. The differences between the perceived duration (PSE) of the first test interval when it was preceded by a high (90%) contrast interval and the perceived duration of the same interval when it was preceded by a low (10%) contrast interval (divided by two sequences, see section Materials and methods for details) are plotted as a function of the drift frequency of the stimuli. Filled black diamonds indicate the means of the duration experiment (empty symbols indicate the individual data for the duration experiment), dark cyan circles indicate the results obtained when subjects were asked to compare the temporal order of the onset (or offset) of an auditory stimulus and the onset (or offset) of a 50% contrast interval and light gray triangles describe the results obtained when both the fixation spot and the stimuli slowly translated across the screen. Error bars show ± 1 SEM.
In the temporal order experiment, in order to test whether changes in duration are due to changes in latency at onset relative to offset, subjects were asked to compare the time of onset (or offset, in separate sessions) of the comparison stimulus with the time of onset of a 30-ms square wave white noise burst produced by a TDT Basic Psychoacoustic Workstation (Tucker-Davis Technologies) and delivered binaurally by Sennheiser HD 265 linear headphones at 80 dB. Subjects reported which occurred first. The comparison stimulus (50% contrast) was in different sessions either preceded by a 90% contrast oscillating grating and followed by a 10% contrast oscillating grating or vice versa. Subjects were tested both at 2 and at 20 Hz. The delay of the auditory stimulus relative to the visual stimulus was varied across trials (range: −200 to +200) to generate a psychometric function and the 50% point provided a measure of the apparent time of onset (or offset) of the visual stimuli.
The pursuit experiment, in which we aimed to investigate the frame of reference of the duration effect, differed from the first experiment in that both the fixation spot and the stimuli (which were randomly displayed across trials in one of four possible positions around the fixation spot) translated slowly (∼6°/s) across the screen. Subjects were required to track the fixation spot, keeping the stimuli on the same position relative to the eye but not to the head. The horizontal starting point of the fixation spot was randomly selected at the beginning of each trial within a range spanning 10° left to 10° right of the center of the screen. Its vertical position was randomly chosen on a trial-by-trial basis within a range spanning 6° below to 6° above the center. At the end of the stimulus sequence, the fixation spot stopped and subjects were required to judge the relative duration of the two tests. Subjects were tested at both 5 and at 20 Hz.
The direct comparison experiment, in which we asked subject to directly compare the relative duration of a 10 with a 90% contrast interval, differed from the pursuit experiment only in the pattern of the contrast sequence. In this case, the standard stimulus had Michelson contrast 90%, the comparison 10% and all the background intervals had the same contrast (50%). Subjects were tested both at 5 and at 20 Hz.
In the static context experiment, we measured the effect of a static contrast context on perceived duration in a way that was procedurally identical to the first experiment. The context intervals were static luminance-modulated sinusoidal gratings (their phase was randomly varied both within and across trials). The test stimuli drifted, in separate session, at either 5 or 20 Hz.
Perceived speed and contrast
To measure perceived speed we employed the same sequence as in the first experiment but in this experiment subjects compared the relative speed of the test intervals, the duration of which was fixed at 500 ms. The spatial frequency was always 1 c/degree, therefore the numerical value of the speed and the temporal frequency was the same. The speed of the standard interval was set to 5 or 20°/s (in separate sessions), while the speed of the comparison varied from trial to trial (range 2–8°/s when the standard drifted at 5, 12–28°/s when the standard drifted at 20°/s) to determine a psychometric function. The speed of the oscillating background intervals matched the standard. Subjects were asked to report which of the tests appeared to drift faster. The 50% point of the psychometric function provided an estimate of the perceived speed of the standard.
In the perceived contrast experiment the stimulus configuration was identical to the first experiment but the contrast of the comparison interval was varied rather than the duration to generate a psychometric function. The point of subjective equality (PSE) provided a measure of perceived contrast. Note that if the test interval is preceded by high contrast the comparison will be preceded by low contrast so the percentage change reported combines contextual effects on the standard and comparison. We also measured perceived contrast of high and low prior context intervals against a simultaneously displayed isolated comparison placed at an unadapted location at the opposite side of the fixation point.
Perceived duration at isoluminance
The chromatic contrast experiment was procedurally identical to the first experiment; however, the stimuli were not modulated in luminance, but in chromaticity. Subjects were tested (in separate sessions) in three different conditions: equiluminance, minimum red luminance – maximum green luminance, minimum green luminance – maximum green luminance. One of the subjects (Alan Johnston) was also tested in other two intermediate luminance levels. Equiluminance was determined individually before the beginning of the experiment with the minimum-motion technique (Anstis and Cavanagh, 1983).
In the chromatic direction discrimination experiment the stimuli were equiluminant chromatic gratings. The stimulus configuration was the same as the first experiment but subjects reported on the drift direction of the stimuli rather than their duration. Threshold was determined using a two alternative forced choice task. The chromatic contrast of the two tests (which had fixed duration – 500 ms – and drifted in opposite directions) was varied over trials between 2% and 50% and subjects reported which one of the tests appeared to drift in the upper right direction. The drift temporal frequency of the stimuli was 20 Hz. The 75% point of the psychometric function provided an estimate of the direction discrimination threshold.
Observers
Two authors (Alan Johnston, Aurelio Bruno) and a naïve subject (IA) participated in the perceived duration, temporal order, retinotopic “gain”, perceived temporal frequency and in the static background experiment. Two authors (Alan Johnston, Aurelio Bruno) and two naïve subjects (IA, AR) participated in the direct comparison experiment. Two authors (Alan Johnston, Aurelio Bruno) and a naïve subject (IA) participated in the perceived temporal frequency and static background tasks. Four subjects (two authors – Alan Johnston, Aurelio Bruno – and two naives – AA, FB) participated in the chromatic contrast experiment. One author (Aurelio Bruno) and two naives (IA, AR) participated in both the perceived contrast experiments. Finally, two authors (Alan Johnston, Aurelio Bruno) participated in the direction discrimination experiment.
Statistical Analysis
The PSIGNIFIT toolbox version 2.5.6 for Matlab (which implements the maximum-likelihood method described by Wichmann and Hill (2001a), see http://bootstrap-software.org/psignifit/) was used to compare the psychometric functions of the two contrast sequences (10% or 90% contrast inducer, unless otherwise noted in the text) for different conditions and for each subject individually. A function was fitted to the data of each sequence separately (d1 and d2) and also for the two sequences combined. The observed differences between PSEs (P2–P1) and slopes (s2–s1) of the two sequences were noted. PSIGNIFIT then performs Monte Carlo simulations of d1 and of d2 under the assumption that they were both generated by binomial processes from the same function, i.e., from the combined fit. The result is a Monte Carlo distribution of threshold differences and slope differences, against which the observed difference (P2–P1, s2–s1) is compared using two-dimensional density estimation with a Gaussian kernel. A density value is computed for each point of the distribution, and for the observed value. The significance value p is then given by an estimate of the probability that densities from the Monte Carlo distribution are smaller than that from the observed difference.
Since the comparison between the two distributions performed by PSIGNIFIT is equally affected by differences in PSE and slope of the two psychometric functions a statistically significant result might in principle have been due to a large difference between the two slopes, even in the absence of a substantial difference between PSEs. We are mainly interested in differences between PSEs; when a significant difference between the two psychometric functions is detected by PSIGNIFIT, we also report the individual PSEs and the 95% confidence intervals (also calculated by PSIGNIFIT). Means, standard errors and individual subject data are shown in the figures. The results of the analyses for individual psychometric functions that give rise to significant differences are shown in Table 1.
Results
Effects of Luminance Contrast Gain on Perceived Duration Judgments
In the first experiment, observers were presented with two test intervals containing a drifting grating of 50% contrast. In one condition, the first interval was preceded by a high contrast (90%) and the second by a low contrast (10%) oscillating sine grating (Figure 1A). In a second condition (not shown), this pattern was reversed to control for position in the sequence effects. To avoid the effects of slow temporal frequency adaptation on duration perception (Johnston et al., 2006), the sequence location was randomized within a notional annulus centered on fixation from trial to trial. To reduce cortical, orientation-specific adaptation, stimuli in the test intervals were rotated by 90° relative to those in the adaptation intervals. Both test and inducer gratings drifted at the same speed and we tested a range of different speeds between 2 and 20°/s. The subject’s task was to report which of the 50% contrast intervals appeared to last longer. The duration of one of the test intervals was varied systematically to generate a psychometric function. The PSE provided a measure of the difference in perceived duration induced by the contrast context. In Figure 1B, we plotted the change in apparent duration (relative to the actual duration) for a 50% contrast test following a 90% contrast interval relative to when it preceded by a 10% contrast interval. We can see that, for high temporal frequencies (≥10 Hz, °/s) the duration effect was less than 0 indicating that the 50% contrast test interval was perceived as shorter when preceded by a 90% contrast interval compared to when preceded by a 10% contrast interval. Means and standard errors are shown in Figure 1B. The results of statistical tests on individual subjects are shown in Table 1. All subjects showed a significant effect at 20 Hz, while only two of them showed an effect at 10 Hz. The duration effect was absent at low drift rates (2 and 5 Hz, °/s). The specificity of the contrast induced change in perceived duration to medium and high temporal frequencies, along with the physiological evidence that fast contrast adaptation occurs principally in the magnocellular pathway, implicates the magnocellular pathway in adaptation-based time compression.
To check the difference in duration in the first experiment was not related to sensory processing times, we separately measured the perceptual latency of the endpoints of the comparison pattern by asking subjects to report on the temporal order of the onset or offset of the test interval and an auditory noise burst (Johnston et al., 2006). The relative timing of the pair was varied to determine a psychometric function for temporal frequencies of 2 or 20 Hz. The duration of the interval expressed as the apparent time of offset minus onset (dark cyan circles, Figure 1B) did not significantly differ across contrast contexts or temporal frequencies (no significant differences for any of the tested subjects were observed between the psychometric functions for high and low context for 2 and 20 Hz, see section Materials and methods for details).
If the site of the adaptation effect is early in the visual pathway we would expect the changes in perceived duration to be selective for position on the retina rather than position in visual space. Burr et al. (2007) have reported that slow adaptation-based duration compression is spatiotopic. We have not found evidence for spatiotopic adaptation but consistently observed strong retinotopic adaptation effects (Bruno et al., 2010). In order to determine whether there is a purely retinotopic effect of contrast context on perceived duration, we asked subjects to track a fixation spot that slowly translated across the screen with the stimuli (which were the same as used in the first experiment). In this case the position of the stimuli changed continuously relative to the head, but remained constant relative to the eye. We asked subjects to report duration judgments at 5 and 20 Hz. In both cases the results (light gray triangles in Figure 1B) showed a pattern, which did not significantly differ from that observed during fixation, indicating the contrast-based temporal compression can occur within a retinotopic coordinate frame.
If the effect of contrast context on the perceived duration was mediated by the magnocellular pathway then we would not expect to see any effect of static inducers on the perceived duration of the test intervals. Data for a condition in which only the test stimuli drifted and the contrast context was static is shown in Figure 2A. We measured apparent duration for drifting 50% contrast intervals just as in the first experiment. There no significant difference for any of the subjects between static high and low contrast prior contexts.
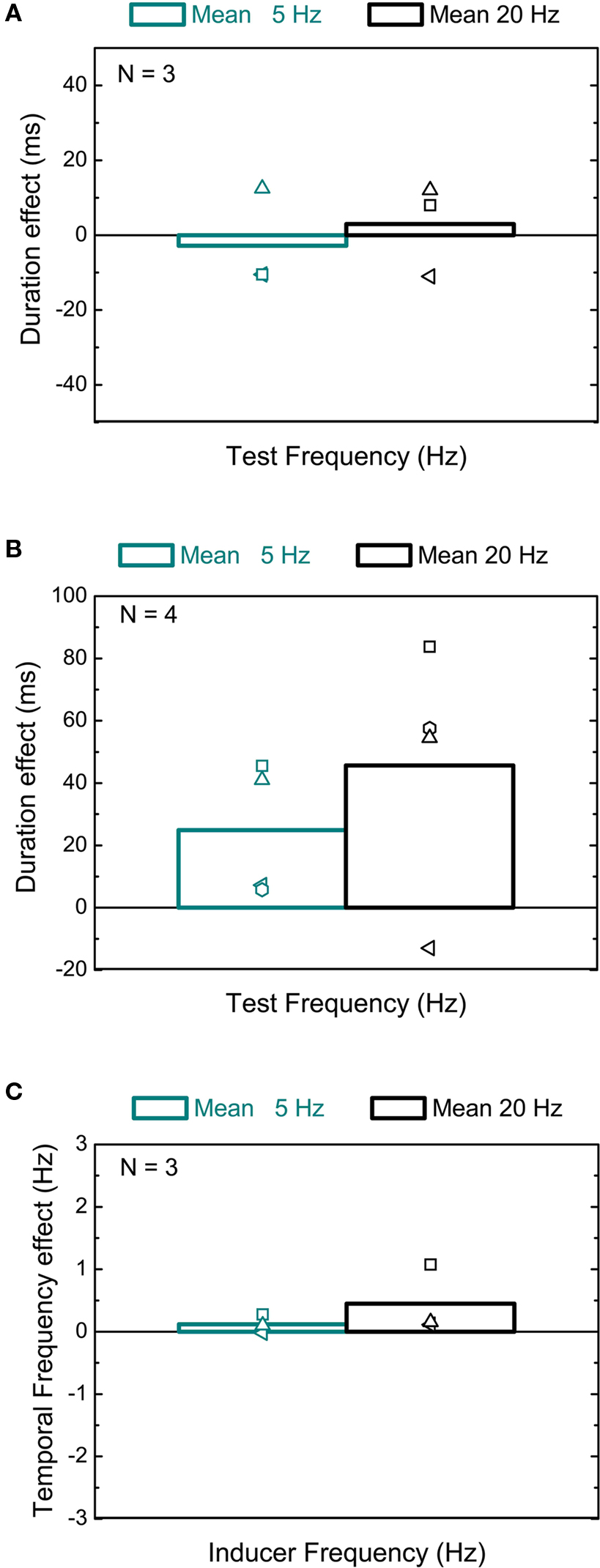
Figure 2. Effects of static contrast context and direct comparison on perceived duration and of luminance contrast on perceived speed. (A) Effects of static contrast context. The differences in perceived duration between the high and low contrast context are plotted against the drift frequency of the test patterns (5 Hz, dark cyan column; 20 Hz, black column) averaged across three different subjects. Empty symbols indicate individual data. (B) Mean duration judgments of a high contrast (90%) compared to a low contrast (10%) interval embedded in a 50% contrast context at 5 (dark cyan column) and 20 Hz (black column). (C) Results for the perceived speed experiment averaged across three different subjects. Dark cyan column indicates the difference in apparent speed between the two contrast contexts at 5 Hz, while black column indicates the same difference at 20 Hz.
A direct comparison of perceived duration between high and low contrast is complicated by two factors. First, contrast gain changes are quick but nevertheless take some time to occur and therefore will be occurring during the measurement interval. Second, the apparent onset and offset of low contrast intervals may differ from those of high contrast intervals. For these reasons virtually all the reported experiments examine the effect of prior contrast on identical intervals. Nevertheless, for completeness, we also measured perceived duration for the direct comparison between a high (90%) and a low (10%) contrast interval embedded in a 50% contrast sequence at 5 and 20 Hz. A general tendency to see the high contrast stimulus as longer than its actual duration (500 ms) was observed at both 5 and 20 Hz (Figure 2B), but no statistical difference emerged from the comparison between the two temporal frequencies for any of the four subjects that we tested.
Effects of Luminance Contrast Gain on Perceived Temporal Frequency and Contrast
Perceived duration has been shown to increase with temporal frequency although this effect saturates above around 6 Hz (Kanai et al., 2006). In order to check whether the changes in duration after high contrast adaptation are mediated by changes in temporal frequency we measured the apparent drift frequency of both a 5 and a 20 Hz standard (50% contrast) following a high (90%) or low (10%) contrast interval. The drift speed of the comparison stimulus (50% contrast) was varied on a trial-by-trial basis and observers reported which of the tests drifted at a faster rate. In general, the shift in perceived frequency was minimal (Figure 2C; only one subject showed a significant increase in apparent frequency at 20 Hz: AJ PSE: 1.026 Hz, 95% CI: 0.23–1.88 Hz). More importantly no significant difference between the two tested contrast sequences was observed, indicating that any change in apparent temporal frequency cannot account for the change in perceived duration.
In order to check whether a possible effect of contrast context on apparent duration might mediate the duration effect, we measured the apparent contrast of a 50% contrast test interval preceded by a high (90%) relative to a low (10%) contrast prior context. Note that if the test interval is preceded by high contrast the comparison will be preceded by low contrast so the percentage change reported combines contextual effects on the standard and comparison. We found around a 15% shift in relative apparent contrast (Figure 3A), which did not depend on the contrast sequence (no significant difference between the individual psychometric functions). The graph shows data for the apparent contrast of a 50% interval following 90% contrast relative to the apparent contrast of a 50% interval following 10% contrast. This shift was almost entirely due to a reduction in apparent contrast induced by high contrast intervals, as demonstrated by comparing a standard (preceded by a high or low contrast interval) to a comparison displayed simultaneously in an unadapted region (see Figure 3B). The reduction in apparent contrast (from 50%) is significant only after a high contrast inducer for all subjects and for both temporal frequencies (see Table 1). Though this change in perceived contrast was slightly bigger at 20 Hz, the fact that there is also a substantial shift at 5 Hz indicates that there is not a direct effect of apparent contrast on duration. Thus the change in contrast per se does not explain the apparent duration compression.
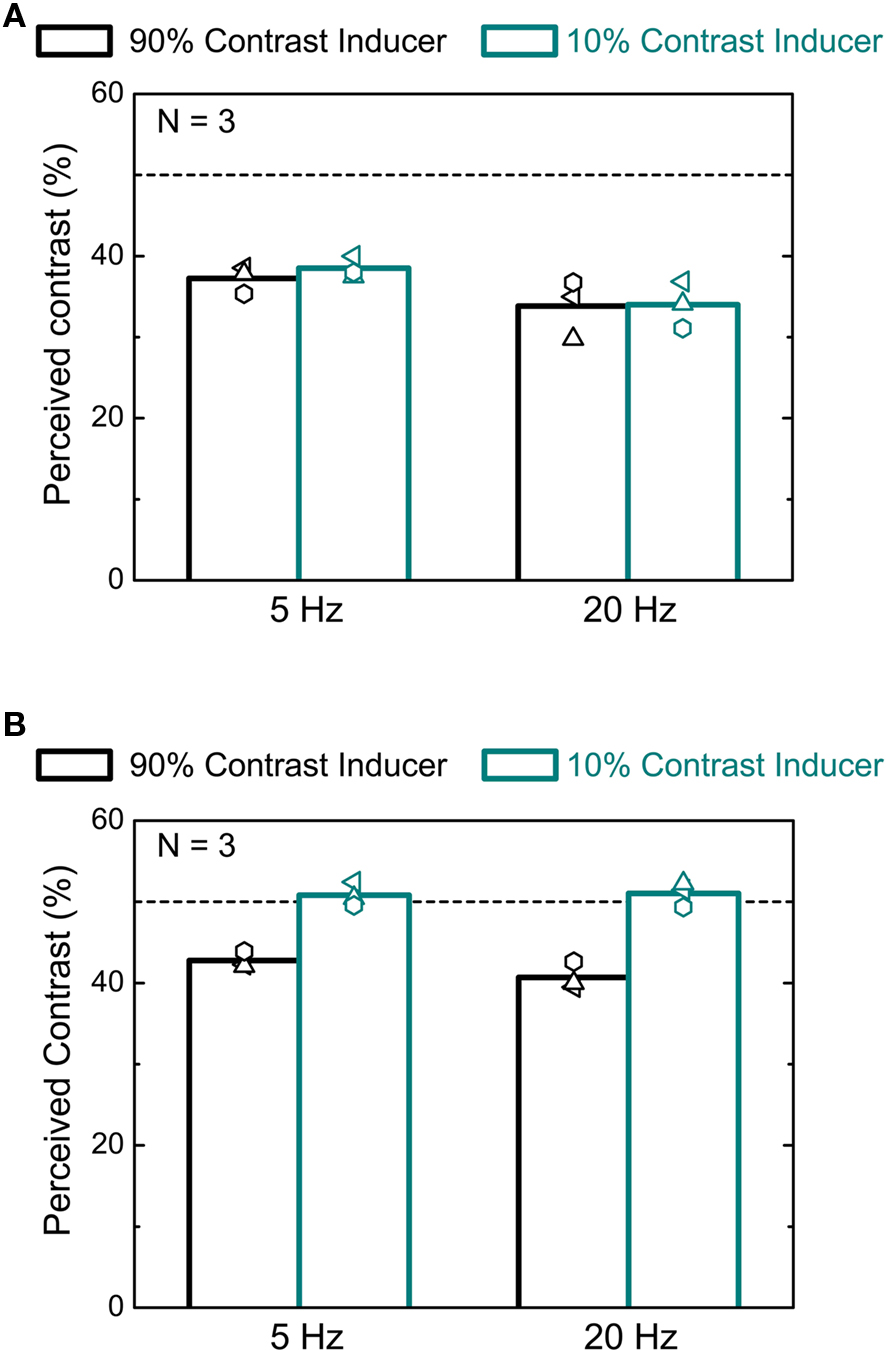
Figure 3. Contrast context induced effects on perceived contrast. (A) Effect of preceding contrast on apparent contrast. In different conditions the standard followed either the 90% (black columns) and 10% (dark cyan columns) intervals. In the graph, for both conditions, we plot the average effects on the perceived contrast of the test interval following 90% contrast as a function of the stimulus frequency. Empty symbols indicate individual data. (B) Estimated contrast (PSE) of a 50% contrast interval after a 90% (black columns) or 10% (dark cyan columns) contrast inducer relative to a simultaneously displayed variable contrast interval displayed in an unadapted position. Data for three subjects and for two temporal frequencies are reported.
Effects of Chromatic Contrast on the Apparent Duration of a Visual Stimulus
If adaptation-based temporal compression was mediated by the magnocellular system, we would not expect to see compression for stimuli defined by chromatic contrast rather than luminance contrast. We first determined the equiluminant point for each subject individually using the minimum-motion method (Anstis and Cavanagh, 1983). We then measured the apparent duration of intervals containing an equiluminant red–green pattern embedded in either a high or a low chromatic contrast context. Otherwise the experimental procedure was identical to the first experiment. Data were also collected with the green or the red components set to 0. At near equiluminance the difference between the condition in which the 50% contrast test was preceded by a 90% contrast interval relative to when it followed a 10% contrast interval is negligible (Figure 4A). When the stimulus was defined by luminance change there was clear duration compression in the high relative to the low contrast context for all subjects (see Table 1), confirming the results reported in Figure 1. Observers are less sensitive to flicker for chromatic contrast than for luminance contrast (Shady et al., 2004) although the critical flicker fusion limit is higher for drifting patterns as compared to flickering patterns (Watanabe and Nishida, 2007). We therefore measured, the direction discrimination threshold at equiluminance for two subjects (data not shown). We found that thresholds were around 10% contrast for the high contrast context (AJHigh, PSE: 13.43, SE: ± 0.02; ABHigh, PSE: 8.96, SE: ± 0.01) and between 5% and 10% for the low contrast context (AJLow, PSE: 9.01, SE: ± 0.01; ABLow, PSE: 5.93, SE: ± 0.01). Since the low contrast level in the chromatic experiment (10%) was close to threshold, we also measured perceived duration for two subjects at a value well above threshold (20%). The difference between the equiluminant and the luminance contrast conditions was statistically unchanged (empty symbols, Figure 4B).
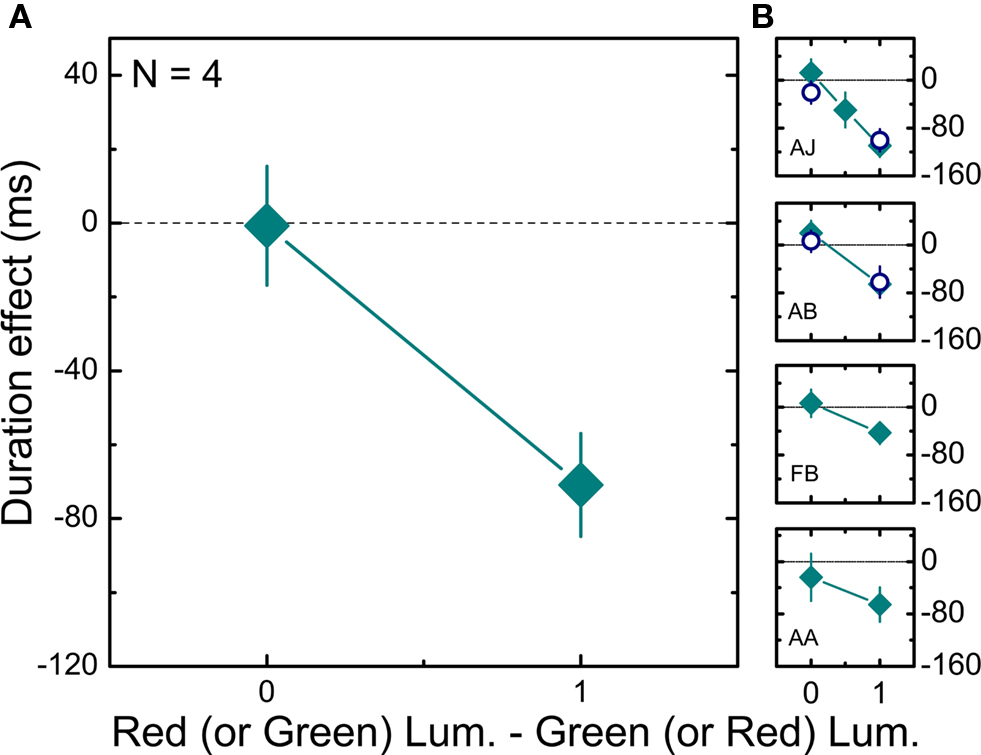
Figure 4. Effects of chromatic contrast on duration judgments. (A) Mean differences (averaged across four subjects) between the estimated duration (PSE) of a 50% chromatic contrast interval when preceded by a high (90%) chromatic contrast interval and when preceded by a low (10%) chromatic contrast interval are plotted as a function of the difference between the amplitude of red and green luminance gratings (0 on the x axis corresponds to isoluminance). Error bars show ± 1 SEM. (B) Individual results for four different subjects. Filled squares describe the results obtained when the minimum contrast level was 10%, while empty circles describe the results obtained when the minimum contrast level was 20%. Error bars show ± 1 SEM.
Discussion
Our results show that the luminance contrast context of a visual stimulus has an effect on its perceived duration. In particular, we showed that fast as well as slow contrast adaptation (Johnston et al., 2006) to oscillating motion produces duration distortions that are limited to high temporal frequency stimulation. If the fast adaptation effect depends upon magnocellular adaptation then we should expect the temporal compression to disappear if we use a static contrast context. Moreover, since M cells have poor sensitivity to equiluminant chromatic contrast (Wiesel and Hubel, 1966; Derrington et al., 1984) we should also expect contrast gain-based duration compression to disappear at equiluminance. The observation that this effect is limited to high drift velocities and that it is absent for static adapters and equiluminant stimuli is consistent with a magnocellular involvement in vision-based timing mechanisms.
The effects of adaptation on temporal frequency can be dissociated from those on apparent duration (Johnston et al., 2006, 2008; Ayhan et al., 2009), however to determine whether changes in apparent duration were mediated by changes in apparent temporal frequency in this paradigm, we measured the perceived drift frequency and we found no substantive shift after adaptation. This rules out the possibility that the effect of luminance contrast context on duration is simply due to a misperception of temporal frequency or speed.
Kanai et al. (2009) have recently shown that the apparent timing of a transient event which is defined as a novel object is delayed relative to when it is defined as a change in a feature of a previously displayed object. In our study, inducer and test grating intervals differ in orientation as well as contrast, therefore the transition between them might be seen as the appearance of a new object rather than a feature change in the same object. However, the absence of substantial changes in apparent onset/offset of our stimuli (Figure 1B, dark cyan circles) implies that the observed duration effect cannot be attributed to different processing times to construct a more stable neuronal representation as suggested by Kanai et al. (2009).
For slow adaptation, the apparent contrast of a high contrast pattern is not affected by adaptation to a lower contrast, although a high contrast pattern will reduce the apparent contrast of a low contrast pattern (Georgeson, 1985). Our results show that the changes in apparent contrast caused by a high or a low contrast inducer are not substantially different between 5 and 20 Hz drift, though we only see changes in apparent duration at high temporal frequencies. This implies that the effects of luminance contrast context on apparent contrast can also be dissociated from the effects on perceived duration.
Our results together with the evidence that time compression induced by slow adaptation to high temporal frequencies is not orientation specific (Johnston et al., 2006), that it can be induced even by invisible flicker (Johnston et al., 2008) and that it is narrowly space-specific (Ayhan et al., 2009) seems to point to an early locus in the visual system for adaptation-based compression. Saccade-based time compression has been associated with remapping of receptive fields in LIP (Morrone et al., 2005; Burr et al., 2007). Saccade-based compression may have a separate neural basis, or, these phenomena may be linked, since there is evidence for suppression of the magnocellular pathway (Burr et al., 1994) and a shortening of the temporal impulse response function during saccades (Burr and Morrone, 1996).
The current study based on fast contrast adaption supports the previous studies on slow contrast adaptation (Johnston et al., 2006, 2008) in suggesting an early site for the locus of the adaptation effect. Contrast adaptation changes the temporal tuning characteristics of the early visual system. In particular the temporal impulse response sharpens and advances (Shapley and Victor, 1978; Benardete and Kaplan, 1999; Stromeyer and Martini, 2003). This change occurs in M cells but not P cells and therefore it provides a signature of magnocellular involvement (Solomon et al., 2004).
A link between the changes in the temporal tuning of the neurons and subsequent biases in duration estimation has been hypothesized in the form of a content-dependent clock model (Johnston, 2010), according to which our brain might employ a “predict and compare” strategy to estimate time intervals. A prediction about what the input image will look like in the near future (for instance, in 100 ms) is continuously compared and cross-correlated with the current image of the visual world provided by the sensory input. When the prediction matches the visual input (peak of the cross-correlation), the clock acknowledges that 100 ms have passed and it ticks, resetting the prediction. In this clock model timing is intimately related to perceptual processing and sensory calibration. To predict forward in time we require a temporal derivative in order to construct a Taylor series expansion of the time series of image brightness at a point. A temporal derivative operator has a biphasic impulse response, as do transient magnocellular neurons, whereas parvocellular neurons are temporally low-pass.
Magnocellular neurons are more prone to adaptation than parvocellular neurons and adaptation has two effects – a reduction in sensitivity and a shift in temporal tuning which causes a phase advance in their response (Benardete and Kaplan, 1999; Kaplan and Benardete, 2001). We show here and in previous studies (Johnston et al., 2006, 2008; Ayhan et al., 2009) that the effects of temporal frequency adaptation on duration can be dissociated from changes in apparent temporal frequency. We proposed that relative changes in changes in sensitivity of temporal channels result in changes in apparent temporal frequency whereas changes in apparent duration are linked to the phase advance. The phase advance (after a high contrast inducer) shifts the prediction forward in time. Consequently, it will take longer for the prediction (carried by the magnocellular system) to match the current input (carried by the parvocellular system) and for the clock to tick, which results in a compression of apparent duration (i.e., less many ticks will be stored in the accumulator for a given interval). The comparison may not be possible until early stages of cortical processing when magnocellular and parvocellular signals combine (Sincich and Horton, 2005).
Morgan et al. (2008) showed a set size effect for a task in which subjects had to report a longer or shorter than average duration target embedded in a field of asynchronously appearing and disappearing elements. They concluded that this means judging duration requires attention. We agree, but this does not preclude the idea that the locus of adaption in adaptation-based compression may be early in the visual time pathway. Note we do not propose that signal duration is extracted in the retina or the LGN. Time judgments will require elaborate perceptual routines specialized to extract temporal information from visual signals. However, changing temporal tuning provides a means of manipulating perceived duration and as such it provides a window on visual time perception.
In conclusion we have shown that a high contrast prior context can reduce the perceived duration of an intermediate contrast interval but only for high temporal frequencies and luminance rather than chromatic contrast. This complements prior results showing a similar compression following slow adaptation to high temporal frequency drifting gratings. These two paradigms are linked through the conditions for the sharpening of the tuning of bandpass temporal filters associated with the magnocellular pathway. These adaptive changes in magnocellular temporal filters are functionally linked to time perception through their proposed role in providing a forward prediction of visual pattern within a “predict and compare” visual clock.
Conflict of Interest Statement
The authors declare that the research was conducted in the absence of any commercial or financial relationships that could be construed as a potential conflict of interest.
References
Anstis, S., and Cavanagh, P. (1983). “A minimum motion technique for judging equiluminance,” in Colour Vision, eds J. D. Mollon L.T. Sharpe (London: Academic Press), 155–166.
Ayhan, I., Bruno, A., Nishida, S. Y., and Johnston, A. (2009). The spatial tuning of adaptation-based time compression. J. Vis. 9, 1–12.
Benardete, E. A., and Kaplan, E. (1999). The dynamics of primate M retinal ganglion cells. Vis. Neurosci. 16, 355–368.
Bruno, A., Ayhan, I., and Johnston, A. (2010). Retinotopic adaptation-based visual duration compression. J. Vis. 10, 1–18.
Burr, D., Tozzi, A., and Morrone, M. C. (2007). Neural mechanisms for timing visual events are spatially selective in real-world coordinates. Nat. Neurosci. 10, 423–425.
Burr, D. C., and Morrone, M. C. (1996). Temporal impulse response functions for luminance and colour during saccades. Vis. Res. 36, 2069–2078.
Burr, D. C., Morrone, M. C., and Ross, J. (1994). Selective suppression of the magnocellular visual pathway during saccadic eye movements. Nature 371, 511–513.
Clifford, C. W., Ibbotson, M. R., and Langley, K. (1997). An adaptive Reichardt detector model of motion adaptation in insects and mammals. Vis. Neurosci. 14, 741–749.
Derrington, A. M., Krauskopf, J., and Lennie, P. (1984). Chromatic mechanisms in lateral geniculate nucleus of macaque. J. Physiol. 357, 241–265.
Derrington, A. M., and Lennie, P. (1984). Spatial and temporal contrast sensitivities of neurones in lateral geniculate nucleus of macaque. J. Physiol. 357, 219–240.
Duong, T., and Freeman, R. D. (2007). Spatial frequency-specific contrast adaptation originates in the primary visual cortex. J. Neurophysiol. 98, 187–195.
Georgeson, M. A. (1985). The effect of spatial adaptation on perceived contrast. Spat. Vis. 1, 103–112.
Ibbotson, M. R. (2005). Contrast and temporal frequency-related adaptation in the pretectal nucleus of the optic tract. J. Neurophysiol. 94, 136–146.
Johnston, A. (2010). “Modulation of time perception by visual adaptation,” in Attention and Time, eds A. C. Nobre and J. T. Coull (Oxford: Oxford University Press), 187–220.
Johnston, A., Arnold, D. H., and Nishida, S. (2006). Spatially localized distortions of event time. Curr. Biol. 16, 472–479.
Johnston, A., Bruno, A., Watanabe, J., Quansah, B., Patel, N., Dakin, S., and Nishida, S. (2008). Visually-based temporal distortion in dyslexia. Vision Res. 48, 1852–1858.
Kanai, R., Carlson, T. A., Verstraten, F. A., and Walsh, V. (2009). Perceived timing of new objects and feature changes. J. Vis. 9, 5.
Kanai, R., Paffen, C. L., Hogendoorn, H., and Verstraten, F. A. (2006). Time dilation in dynamic visual display. J. Vis. 6, 1421–1430.
Kaplan, E., and Benardete, E. (2001). The dynamics of primate retinal ganglion cells. Prog. Brain Res. 134, 17–34.
Mante, V., Bonin, V., and Carandini, M. (2008). Functional mechanisms shaping lateral geniculate responses to artificial and natural stimuli. Neuron 58, 625–638.
Morgan, M. J., Giora, E., and Solomon, J. A. (2008). A single “stopwatch” for duration estimation, a single “ruler” for size. J. Vis. 8, 141–148.
Morrone, M. C., Ross, J., and Burr, D. (2005). Saccadic eye movements cause compression of time as well as space. Nat. Neurosci. 8, 950–954.
Ross, J., Morrone, M. C., and Burr, D. C. (1997). Compression of visual space before saccades. Nature 386, 598–601.
Shady, S., MacLeod, D. I., and Fisher, H. S. (2004). Adaptation from invisible flicker. Proc. Natl. Acad. Sci. U.S.A. 101, 5170–5173.
Shapley, R. M., and Victor, J. D. (1978). The effect of contrast on the transfer properties of cat retinal ganglion cells. J. Physiol. (Lond.) 285, 275–298.
Sincich, L. C., and Horton, J. C. (2005). The circuitry of V1 and V2: integration of color, form, and motion. Annu. Rev. Neurosci. 28, 303–326.
Solomon, S. G., Peirce, J. W., Dhruv, N. T., and Lennie, P. (2004). Profound contrast adaptation early in the visual pathway. Neuron 42, 155–162.
Stromeyer, C. F., 3rd, and Martini, P. (2003). Human temporal impulse response speeds up with increased stimulus contrast. Vision Res. 43, 285–298.
Terao, M., Watanabe, J., Yagi, A., and Nishida, S. (2008). Reduction of stimulus visibility compresses apparent time intervals. Nat. Neurosci. 11, 541–542.
Watanabe, J., and Nishida, S. (2007). Veridical perception of moving colors by trajectory integration of input signals. J. Vis. 7, 1–16.
Wichmann, F.A., and Hill, N.J. (2001a). The psychometric function: I. Fitting, sampling and goodness of fit. Percept. Psychophys. 63, 1293–1313.
Keywords: perceived duration, contrast gain, short-term adaptation, magnocellular pathway
Citation: Bruno A and Johnston A (2010) Contrast gain shapes visual time. Front. Psychology 1:170. doi: 10.3389/fpsyg.2010.00170
Received: 07 July 2010;
Paper pending published: 26 July 2010;
Accepted: 25 September 2010;
Published online: 21 October 2010
Edited by:
Frans Verstraten, Universiteit Utrecht, NetherlandsCopyright: © 2010 Bruno and Johnston. This is an open-access article subject to an exclusive license agreement between the authors and the Frontiers Research Foundation, which permits unrestricted use, distribution, and reproduction in any medium, provided the original authors and source are credited.
*Correspondence: Aurelio Bruno, Department of Cognition, Perception and Brain Sciences, University College London, 26 Bedford Way, London WC1H 0AP, UK. e-mail:YS5icnVub0B1Y2wuYWMudWs=