- 1 Donders Institute for Brain, Cognition, and Behaviour, Radboud University, Nijmegen, Netherlands
- 2 Max Planck Institute for Neurological Research, Cologne, Germany
When our brain detects an error, this process changes how we react on ensuing trials. People show post-error adaptations, potentially to improve their performance in the near future. At least three types of behavioral post-error adjustments have been observed. These are post-error slowing (PES), post-error reduction of interference, and post-error improvement in accuracy (PIA). Apart from these behavioral changes, post-error adaptations have also been observed on a neuronal level with functional magnetic resonance imaging and electroencephalography. Neuronal post-error adaptations comprise activity increase in task-relevant brain areas, activity decrease in distracter-encoding brain areas, activity modulations in the motor system, and mid-frontal theta power increases. Here, we review the current literature with respect to these post-error adjustments, discuss under which circumstances these adjustments can be observed, and whether the different types of adjustments are linked to each other. We also evaluate different approaches for explaining the functional role of PES. In addition, we report reanalyzed and follow-up data from a flanker task and a moving dots interference task showing (1) that PES and PIA are not necessarily correlated, (2) that PES depends on the response–stimulus interval, and (3) that PES is reliable on a within-subject level over periods as long as several months.
When we realize that we have just committed an error, we often stop our current movement for a brief moment or at least we slow down a little bit. This might help us to focus on our task again – that is, to pay more attention to the relevant aspects of the task and ignore irrelevant information – or to realize why we committed an error or what exactly the error was. Alternatively, we slow down just because we are surprised about the unexpected event of committing an error. Sometimes our performance improves after the commission of an error. However, it is yet unknown under which exact circumstances we improve. At least three types of post-error adjustments have been observed. These are post-error slowing (PES; e.g., Rabbitt, 1966; Debener et al., 2005; Eichele et al., 2010), post-error reduction of interference (PERI; Ridderinkhof et al., 2002; King et al., 2010), and post-error improvements in accuracy (PIA; e.g., Laming, 1968; Marco-Pallares et al., 2008; Danielmeier et al., 2011). There is increasing evidence that PES is independent from the other post-error adjustments, but that two or more post-error adjustment processes might occur in parallel. In the following, we will summarize what is known about each of these post-error adjustments and complement this with own data on PES.
Post-Error Slowing
Post-error slowing describes the prolonged reaction time (RT) in trials subsequent to an error compared to RTs in trials following correct trials (Rabbitt, 1966; Laming, 1968). PES has been observed in a variety of different tasks, for instance in flanker (Debener et al., 2005; Krämer et al., 2007; Cavanagh et al., 2009; Eichele et al., 2010), Stroop (Gehring and Fencsik, 2001), Simon (King et al., 2010; Danielmeier et al., 2011), or categorization (Jentzsch and Dudschig, 2009) tasks. However, other studies reported conditions under which no PES effects were observed (e.g., Ullsperger and Szymanowski, 2004; Fiehler et al., 2005). Two recent experiments found PES only in conditions where error trials were infrequent, while observing post-correct slowing when correct trials were infrequent (Notebaert et al., 2009; Nunez Castellar et al., 2010). Thus, it is yet unclear under which conditions PES can be observed, and what the underlying mechanisms are. It has been suggested that PES is either related to cognitive control processes associated with the error (Ridderinkhof et al., 2004), or that it is related to inhibitory motor processes (Marco-Pallares et al., 2008), or that it reflects attentional re-orientation (orienting account; Notebaert et al., 2009) not fulfilling any specific and direct function in terms of performance improvement. In the following we present these theoretical accounts in more detail, then we discuss various factors that might influence PES. We also report two experiments investigating (1) the influence of trial timing on PES, and (2) the intra-individual reliability of PES.
Three Accounts Explaining PES
On the one hand it has been argued that PES is related to cognitive control mechanisms which are implemented after the commission of errors (Botvinick et al., 2001). On the other hand there is evidence that PES reflects an orienting response following infrequent events like errors (Notebaert et al., 2009). Additionally, an inhibition account is supported by functional and structural anatomical studies and EEG experiments showing that motor inhibition is related to PES (Ridderinkhof, 2002b; Marco-Pallares et al., 2008). In the following we will discuss these three explanations for PES, which, to our opinion, are not mutually exclusive.
Cognitive control account
Gehring and Fencsik (2001) suggested that PES is a compensatory control mechanism serving to improve subsequent performance. Thus, in contrast to the orienting account, it is assumed that PES serves a functionally meaningful purpose (cf. Carter and van Veen, 2007). Specifically, it has been suggested that PES serves to buy time to enable more controlled responding (Ridderinkhof et al., 2004). Alternatively, it has been suggested that cognitive control in post-error trials leads to engagement of attentional top-down modulations (MacDonald et al., 2000).
Within the conflict monitoring theory (Botvinick et al., 2001) PES is explained in terms of decreased activity in the response priming unit, which equals an increased motor threshold. This strongly links the cognitive control account with the inhibition account (see below). The assumption of decreased activity in the response unit is supported by fMRI results showing reduced motor activity in post-error trials (King et al., 2010) which is negatively correlated with PES (Danielmeier et al., 2011), i.e., participants with less motor activity in post-error trials show larger PES effects. Motor activity, in turn, was predicted by activity in the performance monitoring network, comprising the posterior median frontal cortex (pMFC), in preceding error trials, with more error-related pMFC activity predicting less motor activity. Since this is a correlational finding one cannot conclude whether pMFC activity causes the decrease in motor activity, for instance via a purposeful adjustment in speed–accuracy thresholds as suggested by Botvinick et al. (2001), or whether the observed activity adjustments just occur coincidentally together. Increased pMFC activity might be the reaction to an error, which also leads to an orienting response that in turn causes the slowing of motor responses.
The cognitive control theory assumes that post-error adjustments are triggered by top-down signals from the performance monitoring system, which has been associated with the pMFC. Several fMRI findings support this assumption by showing a correlation between pMFC activity and PES (Garavan et al., 2002; Kerns et al., 2004; Klein et al., 2007a; Chevrier and Schachar, 2010). Also, the amplitude of the error-related negativity (ERN; Gehring et al., 1993; Debener et al., 2005; West and Travers, 2008; Wessel and Ullsperger, 2011) as well as a mid-frontal power increase (Cavanagh et al., 2009) have been shown to predict the amount of PES in EEG experiments. Some fMRI studies associated PES with increased dorsolateral prefrontal activity in post-error trials (Kerns et al., 2004), which is thought to be driven by pMFC activations (for a review see Carter and van Veen, 2007).
However, some studies did not find any correlation between pMFC activity and PES (Gehring and Fencsik, 2001), while others found a correlation between PES and the error positivity (Pe), instead of the ERN (Nieuwenhuis et al., 2001; Hajcak et al., 2003). Although these results might seem contradictory, one potential reason for some studies finding a correlation and others not, might be that pMFC activity and PES are linked only indirectly. It has been shown that PES is linked to pMFC activity in error trials via an activity decrease in the motor system (King et al., 2010; Danielmeier et al., 2011).
Thus, there is evidence that PES is related to activity in the performance monitoring system, whether directly or indirectly, and therefore, PES might reflect the implementation of cognitive control.
Orienting account
Notebaert et al. (2009) suggested that PES reflects an orienting response to an unexpected event. Since errors are usually rare, they represent unexpected, motivationally salient events. Thus, according to this account, PES is independent from cognitive control processes. Based on this idea, Notebaert et al. (2009) compared the reaction to errors with the reaction to oddball stimuli, which are also infrequent events. Usually, RT slowing can also be observed after surprising (infrequent) events. This might be due to an orienting response elicited by these stimuli. A similar orienting response might be elicited by errors and can be observed as PES. Notebaert et al. (2009) compared an experimental condition where errors were relatively infrequent with another condition where errors were committed frequently and correct trials were infrequent. With infrequent errors, PES was observed as expected. However, when correct trials were infrequent, they observed a post-correct slowing instead. This indicates that PES is not necessarily only reflecting post-error adaptation processes, but depends on the relative frequency of errors. Thus, PES might be an orienting response to an unexpected event, instead of an error-driven cognitive control adjustment.
In an EEG experiment, Nunez Castellar et al. (2010) observed that post-error RTs correlate with the P3 amplitude. The P3, specifically the P3a, has been associated with the novelty processing of an orienting response (Friedman et al., 2001). Thus, the orienting account is corroborated by this ERP finding.
It has also been suggested that PES results from a failure to disengage from the error (Carp and Compton, 2009) or, more generally, from a failure to disengage from performance problems including increased response conflict (Compton et al., 2011). Usually people briefly disengage from the task after correct responses. This has been observed as alpha power increase (indicating a more relaxed status) after correct trials compared to periods following high conflict (e.g., errors). Reduced alpha power after erroneous responses might reflect heightened arousal or orienting responses and therefore could also support the orienting account.
Studies that potentially support the orienting idea of PES are those where PES only occurs in conditions where fewer errors are committed. For instance, Ullsperger and Szymanowski (2004) found PES only in the accuracy condition, where participants committed fewer errors than in the speed condition. Also, Fiehler et al. (2005) divided their subjects in two groups: one group was explicitly instructed to correct their errors immediately, whereas the second group was not aware that they could correct their errors. The second group committed fewer errors than the first group, and only the second group showed PES.
If, in contrast, PES reflected cognitive control processes in the sense that PES serves to improve behavior, one would expect improvements in post-error performance (PIA) to co-occur with PES. Indeed, there are several studies reporting both PES and PIA (Laming, 1968, 1979; Marco-Pallares et al., 2008; Danielmeier et al., 2011). But there are also studies reporting PES in combination with decreased accuracy in post-error trials (Rabbitt and Rodgers, 1977; Fiehler et al., 2005).
However, even if both PES and PIA are shown within the same experiment, this does not necessarily involve a correlation between both factors (Carp and Compton, 2009), i.e., participants with a large PES effect are not necessarily the same participants who show large post-error improvement rates (but see Hajcak et al., 2003, who did find a correlation between PES and PIA). In one of our experiments (Danielmeier et al., 2011) we found both PES and PIA, but the effects did not correlate on the individual subjects level (r = 0.25, p = 0.28; Figure 1). The fact that PES does not necessarily go along with a subsequent performance improvement seems to speak against a direct role for PES in enhancing accuracy. Also, PERI, which is assumed to reflect strategic adjustments in response to errors, and therefore could represent the result of cognitive control processes, does not correlate with PES (King et al., 2010). One possibility could be that PES and cognitive control processes sometimes co-occur within a similar time period, but that these processes are more or less independent. Although, we suggest that PES might have a permissive effect on top-down modulations.
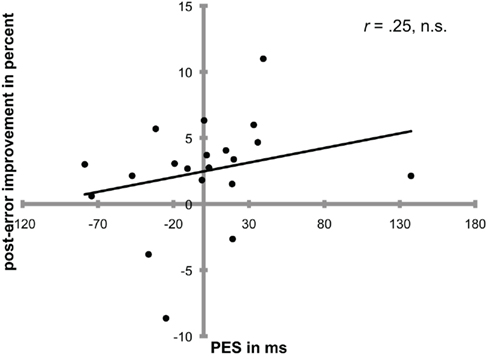
Figure 1. Post-error slowing and post-error improvement in accuracy (PIA) did not correlate significantly in a colored-moving dot interference task (Danielmeier et al., 2011). Participants showed either both PES and PIA (upper right quadrant) or only PIA without PES (upper left quadrant). One participant showed only PES without improvement and two subjects showed neither PES nor PIA following errors.
However, if PES just represented a general orienting response to surprising events, one would expect a comparable response after errors and after surprising false feedback (i.e., signaling the subject an error although the response was correct). But at least three studies have shown that subjects slow down only after self-committed errors, but not after inserted or externally induced errors, i.e., when subjects actually responded correctly (De Bruijn et al., 2004b; Logan and Crump, 2010; Steinhauser and Kiesel, 2011). This suggests that, although there is slowing to a certain extent following infrequent events (e.g., Notebaert et al., 2009), a surprising external event is not always sufficient to evoke PES.
Inhibitory account
Within the activation–suppression hypothesis Ridderinkhof (2002b) already suggested that the commission of an error leads to an increase in selective suppression in the post-error trial. This was revealed by more negative slopes in the RT delta plots of post-error compared to post-correct trials. RT delta plots depict the interference effect as function of response speed, with negative slopes indicating a decreased or reversed interference effect with increasing RTs. In general, the activation of the incorrect response tendency should lead to more inhibition in the subsequent trial (Ridderinkhof, 2002a). There is growing evidence, that a right-hemispheric network, consisting of presupplementary motor area (pre-SMA), lateral inferior frontal cortex (IFC), and subthalamic nucleus (STN) is involved in PES. This network has been associated with motor stopping or slowing (Aron et al., 2007), thus linking PES to motor inhibition. The following studies provide evidence for this assumption.
The inhibition hypothesis of PES was supported by data of Marco-Pallares et al. (2008). They showed that PES correlates with an increase in beta band power in an EEG experiment. The increase in beta band power has been associated with inhibitory processes, and specifically with motor inhibition (Kühn et al., 2004; Marco-Pallares et al., 2008; Swann et al., 2009). In contrast, suppressed beta band oscillations have been associated with faster responses (van Ede et al., 2011). Furthermore, inhibition-related beta band modulations have been associated with the STN (Kühn et al., 2004) and the right IFC (rIFC; Swann et al., 2009). The pre-SMA has been shown to modulate the inhibitory influence of the rIFC (Neubert et al., 2010). The STN, rIFC, and pre-SMA are interconnected and constitute a network that has been associated with motor stopping or slowing (Aron et al., 2007) and action reprogramming (Neubert et al., 2010). Chevrier and Schachar (2010) reported that activity in the right SMA and the dorsal substantia nigra (among other areas) is positively correlated with PES. Since the dorsal substantia nigra is directly adjacent to the STN, it might be difficult to distinguish these areas in fMRI contrasts. We recently found a correlation between individual PES values and structural measures in this right-hemispheric pre-SMA–IFC–STN network (Danielmeier et al., 2011), indicating that this inhibition network also contributes to PES, and complementing the findings that this network operates in the beta band frequency, which in turn is correlated with PES.
The primary motor cortex (M1) is a downstream target of inhibitory control (Swann et al., 2009). In two of our studies, we showed reduced motor cortex activity in post-error trials (King et al., 2010; Danielmeier et al., 2011). This reduction was related to PES, that is, the less M1 activity in post-error trials the more PES was observed in that person. This result supports both the inhibitory account of PES as well as the suggestion of the conflict monitoring theory (Botvinick et al., 2001) that PES reflects an increased response threshold in post-error trials. Furthermore, PES is also correlated with baseline cortisol levels, which in turn have been associated with inhibitory behavior (Tops and Boksem, 2011), suggesting an additional link between PES and behavioral inhibition.
Together, there is evidence that motor inhibition plays a crucial role during PES. However, this does not exclude the possibility that other, probably more cognitive, processes or orienting responses are executed simultaneously. Indeed, we showed that other adjustments can be observed in extrastriate visual areas in post-error trials (King et al., 2010; Danielmeier et al., 2011), but these adjustments were not directly related to PES.
PES is Modulated by Trial Timing
Jentzsch and Dudschig (2009) showed that the PES effect is influenced by the response–stimulus interval (RSI). They manipulated the RSI in a categorization task either between subjects or within subjects in a block-wise fashion. The RSI was either short (50 ms in Experiment 1 or 100 ms in Experiment 2) or long (1000 ms). Although they found significant PES effects in both conditions, PES was considerably larger under short RSI conditions. One question that arises from this experiment is, whether parts of this effect are due to long-term adjustments (macro-adjustments; Ridderinkhof, 2002b), that is, subjects adapt to the higher frequency of stimulus presentation, and thus larger time–pressure, in one block compared to the other block, or whether this effect can also be observed when the RSI varies from trial to trial and subjects cannot adapt to a general stimulus frequency. In Experiment 1, we replicated the timing-dependent effect of PES with a different task, more RSI intervals, and with a randomized trial-to-trial variation of the RSI instead of a block-wise manipulation to exclude the effect of macro-adjustments.
Experiment 1
We conducted an experiment that employed a modified flanker task (Eriksen and Eriksen, 1974). While Jentzsch and Dudschig(2009; Experiment 2) kept the RSI constant for the first half of their experiment and switched to the other RSI in the second half, here RSIs varied from trial to trial in pseudo-random order.
Participants. Nineteen healthy volunteers (eight male; mean age: 24 years; range: 20–27 years of age) participated in this experiment after signing informed consent. All participants had normal or corrected-to-normal vision, one participant was left-handed according to the Edinburgh Handedness Inventory (Oldfield, 1971). The experiment was carried out in accordance with the Declaration of Helsinki.
Task. An arrow version of the flanker task was employed as described in Danielmeier et al. (2009). The only difference to that previous study was the introduction of four different RSIs, which was either 200, 750, 1500, or 3000 ms. In this task a left- or right-pointing arrow was presented as a target in the center of a screen for 50 ms. Two flanker arrows were presented above the target and two below the target. Flanker arrows could either point in the same direction as the target (congruent condition) or in the opposite direction (incongruent condition). Flankers were either presented close to the target (CLOSE condition, i.e., 1.75° and 3.5° above and below the target) or further away from the target (FAR condition, i.e., 4.0° and 6.5° above and below the target), thereby creating modulations in response conflict (cf. Danielmeier et al., 2009). Flanker presentation preceded the target presentation by 80 ms. Speed and accuracy were emphasized equally in the instructions. After the participants’ response, a black screen was presented for the durations of one of four possible RSIs. Each RSI occurred equally often in every experimental condition, but RSI duration varied in pseudo-randomized order. In case no response was recorded, the next trial started after 2000 ms. In total, 996 trials were presented. Stimuli were presented using Presentation 13.1 (Neurobehavioral Systems, San Francisco, CA, USA).
Results and discussion. The effects of congruency and flanker distance showed the same results as in Danielmeier et al. (2009) for each RSI. These results are omitted for brevity, since they are not relevant for our conclusion here. Error rates were 9.12, 9.97, 9.0, and 10.23% for RSI 250, 750, 1500, and 3000 ms, respectively. The RSIs here refer to the time interval following an error, because this interval is crucial for post-error adaptations. There was a trend for error rates being different for the four RSIs [F(3,16) = 2.68; p = 0.08]. However, note that there was no way for participants to influence the RSI following their errors, because the order of RSIs was random and predetermined.
Post-error changes in accuracy were evaluated by comparing post-error error rates with post-correct error rates. There was no significant change in any RSI. PES values were calculated as mean post-error RT minus mean post-correct RT, with post-correct trials being correctly performed trials preceded by at least two and followed by at least one other correct trial. This analysis was done for each RSI separately to investigate the effect of RSI on PES. The indicated RSI here was that between error and post-error trial.
Post-error slowing differed significantly between RSIs [F(3,16) = 4.97; p = 0.013], with shorter RSIs leading to more PES [mean PES values (SEM): 59 ms (21), 11 ms (12), −9 ms (10), and −1 ms (9) for RSI 200, 750, 1500, and 3000, respectively; Figure 2]. Only for RSI 200, the RT in post-error trials was significantly slower than RTs in post-correct trials [t(18) = 2.68; p = 0.015].
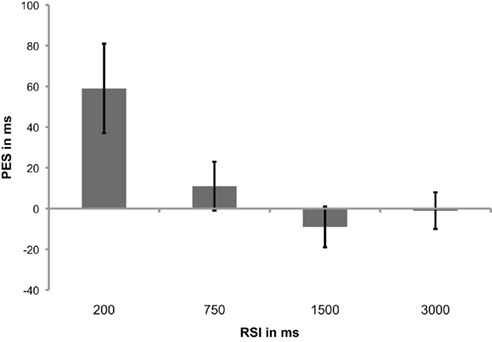
Figure 2. Mean post-error slowing (PES) values (and SEM) for each response–stimulus interval (RSI). PES for RSI 200 is significantly larger than for RSI 1500 and 3000 (both p < 0.005), and there is a trend for a difference between RSI 200 and 750 (p = 0.088). There is also a marginally significant difference between RSI 750 and 1500 (p = 0.088). RSI 1500 and 3000 do not differ.
Thus, we replicated the finding by Jentzsch and Dudschig (2009), showing that PES varies with RSI. Slowing is most pronounced if error and post-error trials are separated only by short time intervals. With longer intervals -in the present task with an RSI of 750 ms or longer- PES was not observed anymore. We extended the results by Jentzsch and Dudschig (2009) by showing that the RSI-dependent PES effect is not exclusively due to macro-adjustments, but is also present when the experimental timing is more variable. That is, the RSI-dependent PES effect in their experiment cannot solely be attributed to the assumption that participants are in a general “speed mode” during one half of the experiment.
Furthermore, the RSI-dependent PES effect can be observed in different types of tasks. It seems like there is a “decay” of PES over time that is independent of the exact task. However, in other experiments PES has been observed with much longer RSIs than 750 ms. For instance, in some fMRI studies there was a substantial PES effect even after 4–5 s (King et al., 2010; Danielmeier et al., 2011). We would speculate that also in those experiments a PES decay takes place, but that the exact timing of this decay might depend on specific requirements of the task, e.g., on task difficulty or whether the general task timing is faster or slower. For instance, Jentzsch and Dudschig (2009) demonstrated a significant PES effect also for RSIs of 1000 ms. As described above, in their task design, RSIs were varied in a block-wise manner, that is the general timing of the task was either very fast (with short RSIs of 50 or 100 ms) or slower for longer periods of time.
PES is Larger after Conscious Error Perception than after Unnoticed Errors
Several studies have investigated conscious error perception or “error awareness.” These tasks aim at evoking both consciously perceived errors and errors that go unnoticed. PES has rarely been observed in “error awareness” tasks, that is, in this type of tasks there seems to be no general PES effect. However, if trials are split into consciously perceived and unperceived errors, an RT slowing has been described following perceived errors, but not after errors that were unnoticed (Nieuwenhuis et al., 2001; Wessel et al., 2011). This suggests that conscious error perception is correlated with PES. However, in a very similar antisaccade task, no PES was observed, neither over all post-error trials nor for perceived errors only, although a subgroup of participants did show PES (Klein et al., 2007a). In this study by Klein et al., however, the average trial duration was 6 s, which presumably reduced the chances of observing PES (cf. Jentzsch and Dudschig, 2009, and Experiment 1). In a Go–Nogo task, Cohen et al. (2009) reported a significant PES effect after unnoticed Nogo errors, although this effect was rather small in magnitude (3.1 ms). However, PES following conscious Nogo errors was considerably larger than PES following unnoticed errors. Hester et al. (2005) investigated post-error RTs in a different error awareness task. They reported PES only for unaware errors, but not for aware errors. Conclusions from this task with respect to PES are limited though, because in the trial directly following an aware error, subjects were required to signal their previous error instead of responding to the stimulus. That is, subjects did not need to process the stimulus in the post-(aware-)error trial. Therefore, it is not surprising that this study found a post-error decrease in RT instead of an increase. In a follow-up study, Hester et al. (2007b) demonstrated that cocaine users do show PES after consciously perceived errors while control subjects did not show PES in the second trial after error commission.
Thus, there is preliminary evidence that PES is larger after consciously perceived errors than after unnoticed errors. However, in order to evaluate the difference between aware and unaware errors on post-error adjustments, further studies employing a larger variety of error awareness tasks are necessary. Ideally, these studies would also be controlled for possible RSI effects.
PES is Greatest when Accuracy is Important
In addition to RSI and possibly error awareness, PES also depends on the importance of responding correctly. When participants were asked to perform the same experiment twice, once with an instruction stressing speeded responding and once with an instruction emphasizing accuracy, participants showed PES only in the accuracy condition, but not under speed instructions (Ullsperger and Szymanowski, 2004). Note that participants also committed fewer errors in the accuracy condition.
Fiehler et al. (2005) compared two groups of participants: one group was instructed to immediately correct their errors, whereas the other group was not instructed to correct themselves, and therefore was unaware that correction responses were recorded. PES was only observed in the non-instructed group. The correction instruction might have reduced subjective error relevance, because of the possibility to correct mistakes. Note that the non-instructed group also committed fewer errors.
These experiments both suggest, that PES is only pronounced if participants try hard to avoid errors and belief that accuracy is crucial. Participants might adapt their motor threshold according to speed or accuracy requirements. This could lead to more PES when accuracy is emphasized. Alternatively, these results suggest that PES occurs predominantly under conditions, when errors are rather infrequent. The latter interpretation is corroborated by findings from Notebaert et al. (Notebaert et al., 2009; Nunez Castellar et al., 2010). They also observed PES only when errors were infrequent, but not when errors were committed frequently.
The Reliability of PES
It has been shown that the ERN amplitude is rather stable within individuals (Segalowitz et al., 2010). Thus, the question arises whether post-error adjustment processes, such as PES, are also reliable within subjects. To investigate whether the PES effect is rather stable over time, we ran a longitudinal study, where participants performed the same flanker task twice at intervals of several months.
Experiment 2
Participants and task. Fifteen volunteers (nine women; mean age: 23.3 years; range: 18–32 years of age) with normal or corrected-to-normal vision participated in this experiment after signing informed consent. All but one participant were right-handed. The experiment was carried out in accordance with the Declaration of Helsinki. Participants were tested twice. On average, the second experimental session took place 4.6 month after the first session (range: 4–6 months).
The task was the same as in Danielmeier et al. (2009). This was the same arrow version of the flanker task as in Experiment 1, except that the RSI did not vary systematically, but was set to 1000 ms. As in the original experiment, participants were instructed to signal their errors. Because participants would not reliably follow this instruction with an RSI of 1000 ms, the RSI was prolonged by 800 ms in case an error was signaled. One thousand trials were presented during the course of the experimental session.
Results and discussion. Error rates were 16.8% (SEM: 1.16%) in the first experimental session, and 14.9% (SEM: 1.78%) in session 2. Error rates did not differ between sessions [t(14) = 1.42; p = 0.18]. In session 1, the average post-error RT (only correct trials following errors) was 366 ms, and post-correct RT was 358 ms. In session 2, the average post-error RT was 364 ms, and post-correct RT was 359 ms. To account for potential baseline differences in RTs between sessions, PES was calculated here as percent RT change in post-error trials compared to RT in correct trials. Although the average PES effect was rather small in this experiment [2.8% RT increase in session 1 (SEM: 0.94% ms) and 1.5% in session 2 (SEM: 1.0%)], individual PES values of both sessions showed a significant correlation (Spearman’s rho = 0.54; p = 0.04; Figure 3). A t-test revealed that PES did not differ between sessions [t(14) = 1.33; p = 0.20].
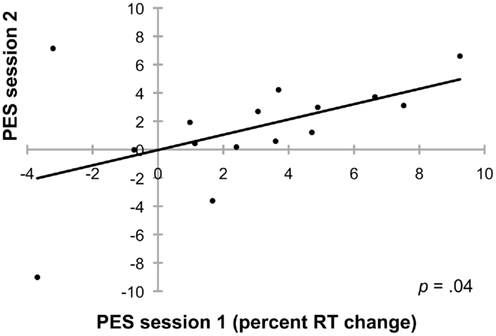
Figure 3. Individual PES values (calculated as RT change in post-error trials relative to RT in correct trials) show a significant correlation between experimental session 1 and experimental session 2. There were on average 4.6 months between the first and the second session.
In sum, the size of the individual PES effect seems to be rather stable over a time period of several months, extending the findings by Segalowitz et al. (2010), who reported intra-individual reliability of PES over periods between 20 min and a couple of weeks. This reliability of PES is corroborated by the fact that the size of the PES effect corresponds to structural differences in white matter (WM) tracts (Danielmeier et al., 2011), which presumably are also stable over time.
Neuroanatomical Basis of PES
Only few fMRI, diffusion-weighted imaging, and patient studies have provided evidence for PES-related brain structures. Probably due to this scarce evidence, the results are not unequivocal yet, although several studies seem to converge in that a network consisting of pre-SMA, lateral IFC, and the STN of the right hemisphere plays a crucial role during the emergence of PES. This network has originally been described by Aron et al. (2007) by using both diffusion-weighted tractography and fMRI. They have associated these brain areas with conflict-related slowing in a conditional-stopping task.
A recent study of our own group directly investigated PES correlates of both diffusion-weighted imaging and fMRI (Danielmeier et al., 2011). We showed that PES is correlated with fractional anisotropy (FA) values in white matter tracts beneath the right pre-SMA. Probabilistic tractography revealed that this WM area belongs to those tracts described by Aron et al. (2007) connecting pre-SMA with lateral IFC and the STN. This suggests that PES and conflict-related slowing engage the same network of brain areas. Additionally, we found FA values in the vicinity of the left anterior midcingulate region to be correlated with PES. This region is part of a network connecting the anterior midcingulate region with dorsolateral prefrontal cortex and more posterior parts of the brain. This could point to the fact that at least two different processes contribute to PES: first, an inhibitory process acting on the motor system, and second, the implementation of cognitive control processes in dorsolateral frontal areas.
By using a voxel-based lesion symptom mapping (VLSM) approach, Molenberghs et al. (2009) showed that the middle third of the right inferior frontal sulcus is crucial for PES. Patients with lesions in this brain area did not show any PES in the Sustained Attention to Response task, while patients with other brain lesions did show PES. This finding corroborates the PES tractography result, demonstrating that the network consisting of right pre-SMA, IFC (or probably more specific: inferior frontal sulcus), and STN is crucial for PES. Furthermore, di Pellegrino et al. (2007) showed that patients with lesions in the medial frontal cortex did not show PES, in contrast to patient with lesions outside the frontal cortex who showed a PES effect comparable to the healthy control group. There is also evidence from an fMRI study with healthy participants that pre-SMA activity is correlated with PES (Klein et al., 2007a).
In the functional data of our recent study, we found that PES is negatively correlated with the activation level in the motor system in post-error trials (Danielmeier et al., 2011). The activity decrease in the motor system in turn is predicted by the level of pMFC activity in the preceding error trial. Thus, pMFC activity (including pre-SMA) seems to downregulate the motor system which leads to PES. A negative correlation between motor activity and the individual PES effect has been shown before in a different task (King et al., 2010). This study by King et al. (2010) also found a positive correlation between PES and the right IFJ, a frontolateral brain area at the junction of the inferior frontal sulcus and the precentral sulcus (Derrfuss et al., 2009).
However, there seem to be two functionally separable brain areas in close proximity in this region of the brain: whereas the posterior inferior frontal gyrus (pIFG) has been associated with response inhibition, the IFJ has been associated with the processing of infrequent events (Chikazoe et al., 2009). Both functions might be crucial for PES. While the response inhibition function of the pIFG would support the inhibitory account, the processing of infrequent events in the IFJ would corroborate the orienting account of PES. A recent TMS study also differentiated IFJ and pIFG of the right hemisphere functionally (Verbruggen et al., 2010). This study concluded that IFJ detects visual changes in stimulus features, whereas the pIFG is responsible for updating action plans.
Taken together, there is converging evidence that the right hemisphere is crucial for PES, and especially a network consisting of pre-SMA, lateral inferior frontal areas, and the STN.
Conclusions on PES
Together, PES seems to be more pronounced (a) with short than with long RSIs, (b) following consciously perceived errors than following unperceived errors, (c) when errors are infrequent events, and (d) when accuracy is emphasized over speed.
In conclusion, there is evidence for all three accounts explaining PES: the cognitive control account, the orienting account and the inhibitory account. We do not think that these three accounts are mutually exclusive. There are elements in each account linking it to another account. For instance, an orienting response most likely recruits inhibitory processes (cf. Ursin, 2005). Also, the model by Botvinick et al. (2001) links cognitive control, and specifically PES, to increases in motor threshold, which could be implemented via inhibitory processes. However, PES seems to be independent from top-down control, but it might facilitate top-down modulations by providing time for attentional adjustments.
Whether cognitive processes co-occur with PES or not might depend, for instance, on task difficulty. If a task is not too difficult, it might be worth it to try to improve performance via top-down modulations. However, if a task is too hard (e.g., because stimuli are visually degraded to an extent that it seems impossible to encode them) additional recruitment of cognitive control processes might not improve performance anymore. Likewise, if the time interval between two stimuli is too short, so that cognitive control processes cannot be implemented to their full extent, we presumably cannot expect performance improvements after errors.
Post-Error Reduction of Interference
Ridderinkhof et al. (2002) described for the first time that the interference effect in a flanker task, i.e., the difference in RTs between compatible and incompatible trials, is reduced after errors. This is the so-called PERI effect, which is thought to reflect cognitive control processes, leading to improvements in interference resolution in post-error trials (e.g., King et al., 2010). PERI is not restricted to flanker tasks, but has also been shown in Simon tasks (Ridderinkhof, 2002b; King et al., 2010).
Interestingly, the PERI effect is influenced by macro-adjustments (Ridderinkhof, 2002b), i.e., adaptations to the broader context of a task that do not vary from trial to trial, but that reflect long-term adjustments, e.g., to be more cautious in difficult tasks. In the study by Ridderinkhof (2002b) the amount of incongruent trials was varied between blocks. When more congruent than incongruent trials were presented, PERI was observed. In contrast, when more incongruent than congruent trials were presented, there was no PERI effect. Ridderinkhof (2002b) argued that in the latter case micro-adjustments, specific to post-error trials, were precluded by long-term macro-adjustments, that is, an adaptation to the circumstance that most trials were incongruent. When the irrelevant stimulus-dimension corresponds to the incorrect response most of the time, this information will be more suppressed in general compared to situations when the irrelevant stimulus-dimension is often associated with the correct response.
Two studies investigated the effect of pharmacological treatments on the PERI effect. De Bruijn et al. (2004a) demonstrated that the PERI effect is absent under lorazepam, a benzodiazepine that modulates fast inhibitory transmission of GABAA receptors. In contrast, under the noradrenergic and specific serotonergic antidepressant mirtazapine, PERI was not different from the control condition without any pharmacological stimulation. Ridderinkhof et al. (2002) showed that PERI is absent after alcohol intake, whereas control subjects did show this reduced interference effect following erroneous trials. Alcohol intake also modulates GABAA receptors (e.g., Steffensen et al., 2011), amongst others.
In a recent fMRI study King et al. (2010) investigated the neuronal correlates of PERI in a face-version of the Simon task, where participants responded to faces and ignored their position. They showed that post-error activations within the left superior frontal sulcus, the left superior colliculus, and, most importantly, the fusiform face area were modulated by the individual PERI effect. This suggests that modulations in task-relevant visual brain areas are crucial for post-error interference reductions. The more activity in these areas was recorded, the greater was the PERI effect, that is, the resolution of interference in post-error trials.
Note that PERI and PES seem to be implemented in different neuronal networks (for a direct comparison see King et al., 2010), suggesting that interference resolution relies more on task-specific brain areas, whereas PES is more associated with brain areas that modulate or are modulated by the motor threshold, independent from the task at hand. Also, the underlying neurotransmitter systems seem to differ between PERI and PES: while PERI was abolished when lorazepam was administered, PES was unaffected by this GABAA-modulating drug.
Post-Error Improvement in Accuracy
One crucial question is whether humans can learn from their errors or otherwise improve their performance after committing an error. PIA can be observed on different time-scales. On the one hand, there are long-term learning effects following errors or negative feedback (e.g., Klein et al., 2007b; Hester et al., 2008) or adjustments that are observed only several trials after an error (Hester et al., 2007a), on the other hand, e.g., in interference tasks, there are short-term or trial-to-trial adjustments that are represented in decreased error rates directly after error commission. We here focus on the latter type of PIAs.
The behavioral findings are not unequivocal with respect to accuracy improvements after errors. Several studies demonstrated improved accuracy directly after error commission (Laming, 1968, 1979; Marco-Pallares et al., 2008; Danielmeier et al., 2011; Maier et al., 2011). Klein et al. (2007a) reported improved performance only after errors that were consciously perceived by the subject, but not after unnoticed errors. Other studies did not find any difference between post-error and post-correct error rates (cf. Experiment 1; Hajcak et al., 2003; Hajcak and Simons, 2008) or even a decrease in accuracy following errors, at least in certain experimental conditions (Rabbitt and Rodgers, 1977; Fiehler et al., 2005). Carp and Compton (2009) showed that both ERN and Pe amplitude correlate with post-error accuracy, in that larger ERN/Pe amplitudes go along with better post-error accuracy. A recent study by Seifert et al. (2011) showed that PIA and PES are abolished in patients with thalamic lesions, whereas healthy controls did show PIA and PES.
Since PES and PIA do not always occur together, they seem to represent different processes. PES and PIA might follow different time courses. While PES is strongest following short RSIs (see Experiment 1), accuracy decreases after errors under short RSI conditions (Jentzsch and Dudschig, 2009). In contrast, with longer RSIs, PES decreases and there is no difference anymore between post-error and post-correct error rates. Post-error improvements in accuracy might predominantly be observed with even longer inter-trial intervals (e.g., mean ITI between 900 and 2250 ms in Marco-Pallares et al., 2008). Thus, time courses of PES and PIA seem to be dissociable.
Another reason, why PIA effects are not unequivocal, might be that there could be large inter-individual differences in the time course of attentional top-down control, which results in PIA. These might additionally interact with the type of task. The task context could be a further factor for variations in PIA results. PIA is presumably only observable when participants really have a chance to improve their behavior after an error. If a task is extremely difficult, i.e., task parameters are set to yield a high percentage of errors (e.g., stimuli are degraded and thus difficult to encode), participants might not have a chance to significantly improve their accuracy. Further studies are needed to specify the conditions, under which PIA can be observed, more precisely.
A practical problem regarding PIA measures arises when participants commit too many or not enough errors in a certain task, leading to ceiling or floor effects in post-accuracy measures. Thus, one needs to keep in mind that PIA results potentially depend on the number of trials/errors in an experiment. Related to this, researchers need to evaluate carefully, whether possible PIA values are fine-grained enough. For instance, if a subject commits 10 errors in a given task, every single double error leads to an increase of the post-error error rate by 10%. Similarly, post-error accuracy cannot be evaluated reliably, when experiments evoke “streaks” of errors, e.g., when experiments are designed in an (over)adaptive fashion so that the task becomes too hard for a too long time, if error rates are too low, and too easy, if error rates are too high. These externally triggered task adaptations potentially lead to error accumulations during periods when the task is harder which cannot be counteracted by internal post-error adaptation. Thus, whenever there is the possibility that error streaks are task-inherent, one should evaluate PIA with caution.
A recent paper by Maier et al. (2011) suggested that attentional post-error adjustments, which presumably are the prerequisite for performance improvements, only occur after certain error types. They employed a flanker task and found post-error attentional adjustments only after so-called flanker errors, but not after other error types. Flanker errors are those errors where subjects were misled by the irrelevant stimulus feature, i.e., the flankers. This suggests that, in order to investigate PIA in interference tasks, it is worthwhile to distinguish between post-error adaptations following those error, that are due to interference with irrelevant stimulus features, and other types of errors.
General Conclusion
We suggest that in most tasks there are at least two post-error processes taking place in parallel: On the one hand there is PES which is associated with inhibitions in the motor system. On the other hand there are adjustments in task-related brain areas, i.e., areas that are directly involved in encoding the stimuli. These latter adjustments might be interpreted in terms of attentional processes or post-error focusing (Verguts et al., 2011), forming the basis for PERI or PIA, which seem to be independent from PES. King et al. (2010) showed that enhanced activity in the task-relevant visual area is accompanied with post-error speeding, not PES.
Thus, although PES seems to be independent from PERI and PIA, it still might have facilitating effects for these other post-error adjustments (but see Verguts et al., 2011, suggesting that slowing and post-error focusing could also counteract each other). The tricky part in investigating this question is that different adjustments might follow different time courses. That is, there might be inhibitory or orienting processes following error commission, but if the time interval between the error and the following trial is too long, this slowing can most likely not be observed in the post-error RT anymore. The processes that lead to PES seem to decay with time. Furthermore, for PERI and PEA there might be other optimal time intervals to observe these effects, and these time intervals presumably also interact with the task context.
From a neuroanatomical perspective, PES is strongly associated with frontal and subcortical structures of the right hemisphere and also with activity decreases in motor areas. PERI and other attentional adjustments act more on task-related visual areas. However, all post-error adjustments seem to be triggered by the pMFC (including pre-SMA and ACC), supporting the idea that error-related pMFC activity signals the need for adjustments.
Conflict of Interest Statement
The authors declare that the research was conducted in the absence of any commercial or financial relationships that could be construed as a potential conflict of interest.
Acknowledgments
We thank S. Aabaid, J. Barbeln, and T. Schueller for help with data collection.
References
Aron, A. R., Behrens, T. E., Smith, S., Frank, M. J., and Poldrack, R. A. (2007). Triangulating a cognitive control network using diffusion-weighted magnetic resonance imaging (MRI) and functional MRI. J. Neurosci. 27, 3743–3752.
Botvinick, M. M., Braver, T. S., Barch, D. M., Carter, C. S., and Cohen, J. D. (2001). Conflict monitoring and cognitive control. Psychol. Rev. 108, 624–652.
Carp, J., and Compton, R. J. (2009). Alpha power is influenced by performance errors. Psychophysiology 46, 336–343.
Carter, C. S., and van Veen, V. (2007). Anterior cingulate cortex and conflict detection: an update of theory and data. Cogn. Affect. Behav. Neurosci. 7, 367–379.
Cavanagh, J. F., Cohen, M. X., and Allen, J. J. (2009). Prelude to and resolution of an error: EEG phase synchrony reveals cognitive control dynamics during action monitoring. J. Neurosci. 29, 98–105.
Chevrier, A., and Schachar, R. J. (2010). Error detection in the stop signal task. Neuroimage 53, 664–673.
Chikazoe, J., Jimura, K., Asari, T., Yamashita, K., Morimoto, H., Hirose, S., Miyashita, Y., and Konishi, S. (2009). Functional dissociation in right inferior frontal cortex during performance of go/no-go task. Cereb. Cortex 19, 146–152.
Cohen, M. X., Van Gaal, S., Ridderinkhof, K. R., and Lamme, V. A. (2009). Unconscious errors enhance prefrontal-occipital oscillatory synchrony. Front. Hum. Neurosci. 3:54. doi: 10.3389/neuro.09.054.2009
Compton, R. J., Arnstein, D., Freedman, G., Dainer-Best, J., and Liss, A. (2011). Cognitive control in the intertrial interval: evidence from EEG alpha power. Psychophysiology 48, 583–590.
Danielmeier, C., Eichele, T., Forstmann, B. U., Tittgemeyer, M., and Ullsperger, M. (2011). Posterior medial frontal cortex activity predicts post-error adaptations in task-related visual and motor areas. J. Neurosci. 31, 1780–1789.
Danielmeier, C., Wessel, J. R., Steinhauser, M., and Ullsperger, M. (2009). Modulation of the error-related negativity by response conflict. Psychophysiology 46, 1288–1298.
De Bruijn, E. R., Hulstijn, W., Verkes, R. J., Ruigt, G. S., and Sabbe, B. G. (2004a). Drug-induced stimulation and suppression of action monitoring in healthy volunteers. Psychopharmacology (Berl.) 177, 151–160.
De Bruijn, E. R. A., Mars, R. B., and Hulstijn, W. (2004b). “It wasn’t me...or was it? How false feedback affects performance,” in Errors, Conflicts, and the Brain. Current Opinions on Performance Monitoring, eds M. Ullsperger and M. Falkenstein (Leipzig: MPI for Human Cognitive and Brain Sciences), 118–124.
Debener, S., Ullsperger, M., Siegel, M., Fiehler, K., Von Cramon, D. Y., and Engel, A. K. (2005). Trial-by-trial coupling of concurrent electroencephalogram and functional magnetic resonance imaging identifies the dynamics of performance monitoring. J. Neurosci. 25, 11730–11737.
Derrfuss, J., Brass, M., Von Cramon, D. Y., Lohmann, G., and Amunts, K. (2009). Neural activations at the junction of the inferior frontal sulcus and the inferior precentral sulcus: interindividual variability, reliability, and association with sulcal morphology. Hum. Brain Mapp. 30, 299–311.
di Pellegrino, G., Ciaramelli, E., and Ladavas, E. (2007). The regulation of cognitive control following rostral anterior cingulate cortex lesion in humans. J. Cogn. Neurosci. 19, 275–286.
Eichele, H., Juvodden, H. T., Ullsperger, M., and Eichele, T. (2010). Mal-adaptation of event-related EEG responses preceding performance errors. Front. Hum. Neurosci. 4:65. doi: 10.3389/fnhum.2010.00065
Eriksen, B. A., and Eriksen, C. W. (1974). Effects of noise letters upon the identification of a target letter in a nonsearch task. Percept. Psychophys. 16, 143–149.
Fiehler, K., Ullsperger, M., and Von Cramon, D. Y. (2005). Electrophysiological correlates of error correction. Psychophysiology 42, 72–82.
Friedman, D., Cycowicz, Y. M., and Gaeta, H. (2001). The novelty P3: an event-related brain potential (ERP) sign of the brain’s evaluation of novelty. Neurosci. Biobehav. Rev. 25, 355–373.
Garavan, H., Ross, T. J., Murphy, K., Roche, R. A., and Stein, E. A. (2002). Dissociable executive functions in the dynamic control of behavior: inhibition, error detection, and correction. Neuroimage 17, 1820–1829.
Gehring, W. J., and Fencsik, D. E. (2001). Functions of the medial frontal cortex in the processing of conflict and errors. J. Neurosci. 21, 9430–9437.
Gehring, W. J., Goss, B., Coles, M. G. H., Meyer, D. E., and Donchin, E. (1993). A Neural system for error-detection and compensation. Psychol. Sci. 4, 385–390.
Hajcak, G., Mcdonald, N., and Simons, R. F. (2003). To err is autonomic: error-related brain potentials, ANS activity, and post-error compensatory behavior. Psychophysiology 40, 895–903.
Hajcak, G., and Simons, R. F. (2008). Oops!.. I did it again: an ERP and behavioral study of double-errors. Brain Cogn. 68, 15–21.
Hester, R., Barre, N., Mattingley, J. B., Foxe, J. J., and Garavan, H. (2007a). Avoiding another mistake: error and posterror neural activity associated with adaptive posterror behavior change. Cogn. Affect. Behav. Neurosci. 7, 317–326.
Hester, R., Simoes-Franklin, C., and Garavan, H. (2007b). Post-error behavior in active cocaine users: poor awareness of errors in the presence of intact performance adjustments. Neuropsychopharmacology 32, 1974–1984.
Hester, R., Barre, N., Murphy, K., Silk, T. J., and Mattingley, J. B. (2008). Human medial frontal cortex activity predicts learning from errors. Cereb. Cortex 18, 1933–1940.
Hester, R., Foxe, J. J., Molholm, S., Shpaner, M., and Garavan, H. (2005). Neural mechanisms involved in error processing: a comparison of errors made with and without awareness. Neuroimage 27, 602–608.
Jentzsch, I., and Dudschig, C. (2009). Why do we slow down after an error? Mechanisms underlying the effects of posterror slowing. Q. J. Exp. Psychol. (Colchester) 62, 209–218.
Kerns, J. G., Cohen, J. D., Macdonald, R. A. W., Cho, R. Y., Stenger, V. A., and Carter, C. S. (2004). Anterior cingulate conflict monitoring and adjustments in control. Science 303, 1023–1026.
King, J. A., Korb, F. M., Von Cramon, D. Y., and Ullsperger, M. (2010). Post-error behavioral adjustments are facilitated by activation and suppression of task-relevant and task-irrelevant information processing. J. Neurosci. 30, 12759–12769.
Klein, T. A., Endrass, T., Kathmann, N., Neumann, J., Von Cramon, D. Y., and Ullsperger, M. (2007a). Neural correlates of error awareness. Neuroimage 34, 1774–1781.
Klein, T. A., Neumann, J., Reuter, M., Hennig, J., Von Cramon, D. Y., and Ullsperger, M. (2007b). Genetically determined differences in learning from errors. Science 318, 1642–1645.
Krämer, U. M., Cunillera, T., Camara, E., Marco-Pallares, J., Cucurell, D., Nager, W., Bauer, P., Schule, R., Schols, L., Rodriguez-Fornells, A., and Munte, T. F. (2007). The impact of catechol-O-methyltransferase and dopamine D4 receptor genotypes on neurophysiological markers of performance monitoring. J. Neurosci. 27, 14190–14198.
Kühn, A. A., Williams, D., Kupsch, A., Limousin, P., Hariz, M., Schneider, G. H., Yarrow, K., and Brown, P. (2004). Event-related beta desynchronization in human subthalamic nucleus correlates with motor performance. Brain 127, 735–746.
Laming, D. (1979). Choice reaction performance following an error. Acta Psychol. (Amst.) 43, 199–224.
Logan, G. D., and Crump, M. J. (2010). Cognitive illusions of authorship reveal hierarchical error detection in skilled typists. Science 330, 683–686.
MacDonald, R. A. W., Cohen, J. D., Stenger, V. A., and Carter, C. S. (2000). Dissociating the role of the dorsolateral prefrontal and anterior cingulate cortex in cognitive control. Science 288, 1835–1838.
Maier, M. E., Yeung, N., and Steinhauser, M. (2011). Error-related brain activity and adjustments of selective attention following errors. Neuroimage 56, 2339–2347.
Marco-Pallares, J., Camara, E., Munte, T. F., and Rodriguez-Fornells, A. (2008). Neural mechanisms underlying adaptive actions after slips. J. Cogn. Neurosci. 20, 1595–1610.
Molenberghs, P., Gillebert, C. R., Schoofs, H., Dupont, P., Peeters, R., and Vandenberghe, R. (2009). Lesion neuroanatomy of the sustained attention to response task. Neuropsychologia 47, 2866–2875.
Neubert, F. X., Mars, R. B., Buch, E. R., Olivier, E., and Rushworth, M. F. (2010). Cortical and subcortical interactions during action reprogramming and their related white matter pathways. Proc. Natl. Acad. Sci. U.S.A. 107, 13240–13245.
Nieuwenhuis, S., Ridderinkhof, K. R., Blom, J., Band, G. P., and Kok, A. (2001). Error-related brain potentials are differentially related to awareness of response errors: evidence from an antisaccade task. Psychophysiology 38, 752–760.
Notebaert, W., Houtman, F., Opstal, F. V., Gevers, W., Fias, W., and Verguts, T. (2009). Post-error slowing: an orienting account. Cognition 111, 275–279.
Nunez Castellar, E., Kuhn, S., Fias, W., and Notebaert, W. (2010). Outcome expectancy and not accuracy determines posterror slowing: ERP support. Cogn. Affect. Behav. Neurosci. 10, 270–278.
Oldfield, R. C. (1971). The assessment and analysis of handedness: the Edinburgh inventory. Neuropsychologia 9, 97–113.
Rabbitt, P., and Rodgers, B. (1977). What does a man do after he makes an error? An analysis of response programming. Q. J. Exp. Psychol. 29, 727–743.
Rabbitt, P. M. (1966). Errors and error correction in choice-response tasks. J. Exp. Psychol. 71, 264–272.
Ridderinkhof, K. R. (2002a). “Activation and suppression in conflict tasks: empirical clarification through distributional analyses,” in Common Mechanisms in Perception and Action, eds W. Prinz and B. Hommel (Oxford: Oxford University Press), 494–519.
Ridderinkhof, K. R. (2002b). Micro- and macro-adjustments of task set: activation and suppression in conflict tasks. Psychol. Res. 66, 312–323.
Ridderinkhof, K. R., De Vlugt, Y., Bramlage, A., Spaan, M., Elton, M., Snel, J., and Band, G. P. (2002). Alcohol consumption impairs detection of performance errors in mediofrontal cortex. Science 298, 2209–2211.
Ridderinkhof, K. R., Van Den Wildenberg, W. P. M., Wijnen, J., and Burle, B. (2004). “Response inhibition in conflict tasks is revealed in delta plots,” in Cognitive Neuroscience of Attention, ed. M. I. Posner (New York: Guilford Press), 369–377.
Segalowitz, S. J., Santesso, D. L., Murphy, T. I., Homan, D., Chantziantoniou, D. K., and Khan, S. (2010). Retest reliability of medial frontal negativities during performance monitoring. Psychophysiology 47, 260–270.
Seifert, S., Von Cramon, D. Y., Imperati, D., Tittgemeyer, M., and Ullsperger, M. (2011). Thalamocingulate interactions in performance monitoring. J. Neurosci. 31, 3375–3383.
Steffensen, S. C., Bradley, K. D., Hansen, D. M., Wilcox, J. D., Wilcox, R. S., Allison, D. W., Merrill, C. B., and Edwards, J. G. (2011). The role of connexin-36 gap junctions in alcohol intoxication and consumption. Synapse 65, 695–707.
Steinhauser, M., and Kiesel, A. (2011). Performance monitoring and the causal attribution of errors. Cogn. Affect. Behav. Neurosci. 11, 309–320.
Swann, N., Tandon, N., Canolty, R., Ellmore, T. M., Mcevoy, L. K., Dreyer, S., Disano, M., and Aron, A. R. (2009). Intracranial EEG reveals a time- and frequency-specific role for the right inferior frontal gyrus and primary motor cortex in stopping initiated responses. J. Neurosci. 29, 12675–12685.
Tops, M., and Boksem, M. A. (2011). Cortisol involvement in mechanisms of behavioral inhibition. Psychophysiology 48, 723–732.
Ullsperger, M., and Szymanowski, F. (2004). “ERP Correlates of error relevance,” in Errors, Conflicts, and the Brain. Current Opinions on Performance Monitoring, eds M. Ullsperger and M. Falkenstein (Leipzig: MPI for Human Cognitive and Brain Sciences), 171–184.
Ursin, H. (2005). Press stop to start: the role of inhibition for choice and health. Psychoneuroendocrinology 30, 1059–1065.
van Ede, F., De Lange, F., Jensen, O., and Maris, E. (2011). Orienting attention to an upcoming tactile event involves a spatially and temporally specific modulation of sensorimotor alpha- and beta-band oscillations. J. Neurosci. 31, 2016–2024.
Verbruggen, F., Aron, A. R., Stevens, M. A., and Chambers, C. D. (2010). Theta burst stimulation dissociates attention and action updating in human inferior frontal cortex. Proc. Natl. Acad. Sci. U.S.A. 107, 13966–13971.
Verguts, T., Notebaert, W., Kunde, W., and Wuhr, P. (2011). Post-conflict slowing: cognitive adaptation after conflict processing. Psychon. Bull. Rev. 18, 76–82.
Wessel, J. R., Danielmeier, C., and Ullsperger, M. (2011). Error awareness revisited: accumulation of multimodal evidence from central and autonomic nervous systems. J. Cogn. Neurosci. 23, 3021–3036.
Wessel, J. R., and Ullsperger, M. (2011). Selection of independent components representing event-related brain potentials: a data-driven approach for greater objectivity. Neuroimage 54, 2105–2115.
Keywords: post-error slowing, post-error reduction of interference, post-error improvement in accuracy, cognitive control, orienting response, inhibition, posterior medial frontal cortex
Citation: Danielmeier C and Ullsperger M (2011) Post-error adjustments. Front. Psychology 2:233. doi: 10.3389/fpsyg.2011.00233
Received: 30 June 2011;
Paper pending published: 12 July 2011;
Accepted: 29 August 2011;
Published online: 15 September 2011.
Edited by:
Wim Notebaert, Ghent University, BelgiumReviewed by:
Mattie Tops, University of Groningen, NetherlandsJames F. Cavanagh, Brown University, USA
Copyright: © 2011 Danielmeier and Ullsperger. This is an open-access article subject to a non-exclusive license between the authors and Frontiers Media SA, which permits use, distribution and reproduction in other forums, provided the original authors and source are credited and other Frontiers conditions are complied with.
*Correspondence: Claudia Danielmeier, Donders Institute for Brain, Cognition, and Behaviour, Radboud University, Montessorilaan 3, 6525 HR Nijmegen, Netherlands. e-mail:Yy5kYW5pZWxtZWllckBnbWFpbC5jb20=