- 1Chemistry Department, Moscow State University, Moscow, Russia
- 2Federal Research Center Kazan Scientific Center of Russian Academy of Sciences, Kazan, Russia
Resistive type gas sensors based on wide-bandgap semiconductor oxides are remaining one of the principal players in environmental air monitoring. The rapid development of technology and the desire to miniaturize electronics require the creation of devices with minimal energy consumption. A promising solution may be the use of photoactivation, which can initiate/accelerate physico-chemical processes at the solid-gas interface and realize detection of flammable and explosive gases at close to room temperature. This work examines the mechanism underlying the increased sensitivity to various gases under photoactivation. The review is intended to clarify the current situation in the field of light-activated gas sensors and set the vector for their further development in order to integrate with the latest technological projects.
1 Introduction
Over the past decades, the rapid development of industry has led to an increase in the number and type of pollutants and toxic substances in the atmosphere. The pollution level often exceeds maximum permissible concentrations in megapolices and industrial areas, which can lead to serious health problems and climate changes (World Health Organization, 2006; National Research Council, 2012; Breisinger, 2012). Therefore, the creation of air monitoring systems is an extremely important task (Santhanam and Ahamed, 2018). Currently, to determine trace gas concentrations research centers and technology laboratories use different complex methods as gas chromatography, mass spectrometry and infrared spectroscopy, which are not suitable for mass use because of the high cost of the instruments, their overall dimensions, large weight, high power consumption and complex maintenance (Wang et al., 2023). An alternative could be the development of miniature sensors for express and mobile gas detection (White and Turner, 1997; Nikolic et al., 2020; Zhang et al., 2016).
Resistive gas sensors are promising for wide practical application due to their simple design and low cost, high sensitivity, fast response and the possibility of integration into electronic devices (Nikolic et al., 2020; Neri, 2015; Chai et al., 2022; Korotcenkov et al., 2013). Wide gap semiconductor metal oxides (SMOs) are most often used as the sensitive materials. The analytical signal is formed during the interaction of gas with the surface layer of the semiconductor that leads to a change in resistance (Nikolic et al., 2020; Wang et al., 2010). Significant disadvantages of such sensors include insufficient selectivity and stability, as well as relatively high power consumption caused by high operating temperatures (250–500°C). The development of new strategies for the effective gas detection at close to room temperature (RT) has great potential (Joshi et al., 2018; Majhi et al., 2021a; Srinivasan et al., 2019). Light activation (Espid and Taghipour, 2017; Lee and Yoo, 2022; Wang et al., 2021; Chizhov et al., 2021; Xu and Ho, 2017; Ma Z. et al., 2021; Kumar et al., 2021; Majhi et al., 2021b) allows increasing the concentration of free charge carriers, activating and controlling the kinetics of ongoing chemical processes on the surface of semiconductor oxides, and accelerating the process of desorption of reaction products (Anothainart et al., 2003; Boukhvalov et al., 2021; Liu et al., 2014). This article provides an overview on semiconductor gas sensors operating under illumination and discusses the importance of photoactivation for optimizing gas sensor technology.
2 Photoactivated semiconductor metal oxide gas sensor systems
Over the past decades, the number of scientific publications devoted to the study of photoactivated gas sensors has increased significantly (Figure 1) that demonstrates the effectiveness of this approach in gas detection (Espid and Taghipour, 2017; Wang et al., 2021; Chizhov et al., 2021; Xu and Ho, 2017; Ma Z. et al., 2021; Kumar et al., 2020; Šetka et al., 2021).
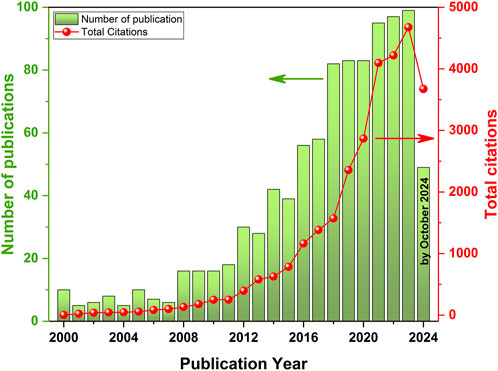
Figure 1. Literature survey of publications for gas sensors operating at RT under photoactivation from 2000 to October 2024, according to Web of Science. Core collection search keywords: TS = gas sensor AND (TS = room temperature OR TS = low temperature) AND (TI = UV OR TI = ultraviolet OR TI = light OR TI = illumination OR TI = LED OR TI = photo OR TI = irradiation OR TI = activation OR TI = photosensitive OR TI = light-activated OR TI = photo-activated).
The effect of ultraviolet (UV) and visible light irradiation with the energy exceeding band gap of semiconductor/photosensitizer, on the receptor function of wide-gap SMOs is determined by the following factors (Majhi et al., 2021b; Scandola et al., 1994).
• Increasing carrier concentration in a semiconductor matrix. When a semiconductor oxide is illuminated with radiation of appropriate energy for the band gap transition, an electron-hole pair is generated and the electron is excited to the conduction band (CB). The photogenerated hole recombines with an electron localized by oxidizing gas molecules chemisorbed on the surface. Photogenerated electrons increase the concentration of charge carriers in the CB, which provides an increase in the conductivity of the n-type semiconductor. The value of conductivity is determined by the dynamic equilibrium of the processes of adsorption and desorption of an oxidizing gas.
• Changing the type and concentration of surface adsorption sites. Photodesorption leads to a decrease in the concentration of chemisorbed oxygen, whose positions can be occupied by water molecules and hydroxyl groups according to the molecular and dissociative adsorption mechanisms, respectively. This leads to a significant increase in the hydrophilicity of the surface of SMOs under UV radiation.
• Formation of highly active radical particles. Upon detection of volatile organic compounds photolysis of analyte molecules can occur on the semiconductor’s surface that facilitates their subsequent oxidation with chemisorbed oxygen, leading to a change in the conductivity of the semiconductor.
• Decreasing the intergrain energy barrier height by changing the intergrain charge states and increasing the probability of tunneling through the intergrain barriers by decreasing the depletion layer widths in the adjacent grains for polycrystalline materials.
The mechanism of sensor signal formation on the surface of the sensitive layer of resistive gas sensors during photoactivation (UV irradiation in the case of pristine SMOs) can be explained on the basis of ionosortpion concept (Das et al., 2022; Ji et al., 2019; Staerz et al., 2022; Mishra et al., 2004; Mall et al., 2018). In air, oxygen molecules can be adsorbed on the surface of a semiconductor oxide in the form of
The polarity of the sensor response depends on the type of gas-analyte and the main charge carriers. Figure 2 shows a schematic representation of n-type (SnO2, In2O3, ZnO, WO3) and p-type (Co3O4, NiO, CuO) SMOs resistance behavior in the presence of oxidizing (NO2, O3, Cl2) and reducing (NH3, CO, H2S) gases in dark conditions and after under light irradiation. When n-type SMO interacts with oxidizing gases with higher electron affinity (Eea (NO2) = 2.27 eV, Eea (O3) = 2.10 eV) then that for O2 molecules (Eea (O2) = 0.44 eV) (Zhou et al., 2001; Zemke et al., 1972), the resistance increases due to electron depletion layer extension. The adsorption of reducing gases, on the contrary, leads to a decrease in resistance due to their reaction with chemisorbed oxygen and releasing electrons into CB. Under illumination, electron-hole pairs are generated that leads to an increase in the concentration of electrons and photodesorption of oxygen from the surface by photogenerated holes (Equation 1). The consequence of these processes is a decrease in the resistance below the baseline value in a pure air atmosphere. Oxidizing gases will be adsorbed at a higher rate under such conditions, increasing the resistance of the sensor. The amplitude of the decreasing resistance when interacting with reducing gases is smaller, since the reaction requires chemisorbed oxygen, which undergoes photodesorption.
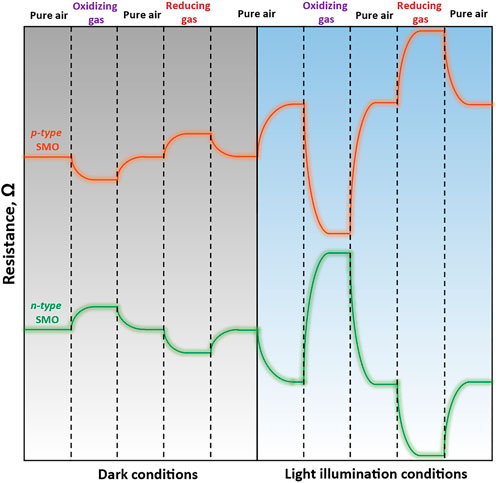
Figure 2. Schematic illustration of SMOs gas sensing response in the presence of oxidizing and reducing gases in dark conditions and under illumination.
SMOs with p-type conductivity react in a conversely manner. These materials have higher baseline resistance comparing to n-type SMOs due to the less-conducting core and semiconducting hole-accumulation shell on the particles’ surface. Their resistance decreases in the atmosphere of oxidizing gases since gas molecules capture the electrons resulting in the increase in holes concentration. When reducing gas interacts with chemisorbed oxygen species the electrons release into the CB and the hole concentration decreases leading to the increase in resistance. Light illumination can further enhance sensing effect due to availability of more charge carrier species.
2.1 Light activation of pristine SMOs
Saura (1994) found that UV illumination could improve the sensitivity of SnO2-based gas sensor towards acetone and trichloroethylene by increasing the concentration of photogenerated charge carriers and decreasing the intergranular barriers height. Later E. Comini et al. achieved an increase in response of SnO2 thin film gas sensors toward NO2 under UV light by concentrating the light flux onto the sensor surface using optical fibers (Comini et al., 2001). These results were supported by Mishra et al. (2004) who proposed a theoretical model of SMOs sensor signal under UV irradiation. They concluded that the sensitivity of sensors based on polycrystalline materials should increase with increasing light fiux density and should decrease with an increase in grain size of the sensor material. A comparative study of single crystal SnO2 nanowires when detecting NO2 under UV photoactivation and in dark conditions demonstrated high sensor response in the first case. The results showed that UV irradiation is a good alternative to thermal heating not only for activation of surface chemical reactions and products desorption, but also for increase in the sensor response towards oxidizing gases (Prades et al., 2009).
A comparative assessment of the efficiency of porous TiO2 and ZnO gas sensors when detecting formaldehyde and acetone at RT under UV light demonstrated that the responses of ZnO were 1,000 times less than the responses of TiO2. Such a huge difference between TiO2 and ZnO was explained by the quantity of chemisorbed oxygen species on the sensors surfaces under UV light confirmed by the ratio of light and dark currents for these materials (Chen et al., 2012). Additionally, mass-spectrometry analysis confirmed the possibility of oxygen photoadsorption on SMOs under UV illumination (Chizhov et al., 2022). As radiation sources, low-power miniature LEDs have certain advantages (Nam et al., 2024). Replacing UV radiation with a visible light can further reduce energy consumption. Moreover, the visible range of the spectrum accounts for the maximum intensity of solar radiation, which can be additionally used as a light source. It is worth noting that wide gap SMOs are optically transparent in the visible spectral range (Soler-Fernández et al., 2024).
Various approaches can be used to improve the selectivity and reduce the power consumption of SMOs based resistive gas sensors by controlling SMOs morphology, microstructure, type and concentration of bulk defects and surface active sites (Nikolic et al., 2020; Cho and Park, 2016). A promising direction is the creation of new optimized gas sensitive materials that absorb visible light. This approach opens up new possibilities and advantages for practical application. Firstly, visible range light sources (mainly low-power miniature LEDs) are currently widely available and inexpensive, unlike sources with high photon energy (UV range), which are still technologically and materially more expensive. Moreover, such sources consume less energy than UV sources. Secondly, as an alternative, natural sunlight can be used, a significant part of which falls in the visible range (∼5% ultraviolet, ∼43% visible and ∼52% IR range). Shifting the SMOs optical sensitivity to the long-wavelength region is possible by creating defects in their crystal structure (Zhang et al., 2017) or modifying their surface with photosensitizers (Bignozzi et al., 1995).
Commercial gas sensors used until the early 2000’s had an energy consumption of about 400 mW–1 W. They were replaced by smaller sensors with a thin film structure that consume 120–280 mW. The next step in the evolution are the sensors based on MEMS (Micro Electro Mechanical Systems) technology, which are currently considered the most energy efficient with a power consumption of 15–60 mW (Burgués and Marco, 2018). Recent studies have shown ultra-low power consumption for sensors integrated with μLEDs (micro light-emitting diodes) operating under illumination. This method can reduce energy consumption up to several hundreds of microwatts (Cho et al., 2020; Lee K. et al., 2023).
2.2 Photosensitization of SMOs
An increase in the SMOs sensitivity and selectivity when detecting various gases under visible light can be achieved using photosensitizers – metal nanoparticles with the plasmon resonance effect (Rossi et al., 2019; Zhang Q. et al., 2018; Gogurla et al., 2014), organic dye molecules (Paolesse et al., 2017; Zhang et al., 2015; Peng et al., 2011) or narrow gap semiconductors of the AIIBVI (CdS with Eg = 2.4 eV; CdSe with Eg = 1.7 eV) and AIIIBV groups (InP with Eg = 1.35 eV) (Chizhov et al., 2016; Geng et al., 2016; Vasiliev et al., 2013; Yang et al., 2014).
Plasmon resonance (PR) is known as the phenomenon of collective oscillation of valence electrons relative to the atomic core level, coinciding in frequency with external light radiation (Rossi et al., 2019; Khurgin, 2019; Basova et al., 2016). The most mentioned type of sensitizers exhibiting the PR effect under visible light are gold and silver nanoparticles possessing high extinction coefficient and stability (Suh et al., 2021; Panayotov and Morris, 2016). By varying the size and shape of nanoparticles, their extinction coefficient can be controlled over a fairly wide spectral range (Lu et al., 2009; Linic et al., 2011). The photosensitization by plasmonic nanoparticles may be associated with various effects (Chizhov et al., 2021; Rossi et al., 2019; Manjavacas et al., 2014; Kim et al., 2018; Zhang Y. et al., 2018; Ma Q. et al., 2021; Watanabe et al., 2006).
• The PR effect causes the formation of inhomogeneous electric field near metal nanoparticles, which can sufficiently influence on the formation of electron-hole pairs in a semiconductor.
• The multiple scattering of photons by plasmonic nanoparticles can increase the optical path of light.
• Plasmonic nanoparticles can be a source of local heat generation.
In work Zhang Q. et al. (2018) the authors obtained heterostructures based on polycrystalline ZnO and Ag nanoparticles. Compared with pure ZnO, the ZnO/Ag heterostructures show an increase in the sensor response towards NO2 (0.5–5 ppm) at RT under photoactivation in 365–520 nm spectral range. The maximum signal and the greatest selectivity were achieved at λ = 470 nm corresponding to the plasmon resonance of Ag nanoparticles. Encapsulation of Ag nanoparticles in zeolitic imidazolate frameworks-8 (ZIF-8) is another strategy for increasing sensitivity by combining high porous material with photoactive particles. The crucial role of the Ag nanoparticles size effect on triethylamine sensing was shown: the less the particles’ size the better effect of plasmon resonance realized, resulting in higher sensor signal (Yao et al., 2024).
Decoration of ZnO with Au nanoparticles can also lead to significant enhancement of gas sensing parameters. Thus, combination of several effects, like localized surface plasmon in Au nanoparticles, spillover effect and the enhancement of chemisorption and dissociation of gas results in the enhanced sensor response of the Au-modified ZnO nanosheet sensor to NO2 at RT (Mun et al., 2013). Sensitivity of Au-ZnO nanocomposite can also be enhanced both in UV and visible region, leading to detection of NO (Gogurla et al., 2014). Composite systems modified with bimetallic clusters, like AuAg/ZnO (Sun et al., 2024a) and Pd@Ni/ZnO (Sun et al., 2024b), showed superior CH4 sensing performance at RT under UV illumination.
Organic photosensitizers – organometallic complexes, are of particular interest because the central cation can be an active adsorption or catalytic center in solid-gas interaction, providing an increase in selectivity. Under suitable radiation, the organometallic complex goes into an excited state, the electrons first move from the highest occupied molecular orbital (HOMO) to the lowest unoccupied molecular orbital (LUMO), from where they can be transferred to the semiconductor’s CB. Photogenerated holes remaining at the HOMO level can drift to the crystal under electric field gradient and subsequently recombine with electrons localized on chemisorbed molecules of oxidizing gases. Thus, visible light irradiation of hybrid materials leads to the photodesorption of oxidizing gases (Anothainart et al., 2003).
To increase the efficiency of detecting gases under visible light using hybrid materials, a number of requirements are imposed on an organic photosensitizer (Kaushik et al., 2015).
• high molar extinction coefficient in the spectral region coinciding with the LED radiation energy;
• chemical stability, thermal stability and photostability towards probe analytes and external conditions;
• optimal alignment of energy levels to ensure energetically favorable charge transfer: the LUMO of the photosensitizer should be higher in energy than semiconductor’s CB, and the HOMO should be between the semiconductor’s CB and the oxidation reaction potential;
• optimal arrangement of the photosensitizer molecular parts in space: HOMO should be far away from the semiconductor surface, LUMO should be on the ligand or fragment of the molecule associated with the semiconductor surface.
A number of works show effective results on using organic molecules as photosensitizers. A study of the gas sensor properties of hybrid materials with heterocyclic Ru(II) complexes towards NO or NO2 in pulsed illumination mode with different wavelengths (470, 525 and 630 nm) showed that these photosensitizers allow shifting spectral sensitivity to the visible region and detecting low concentrations of nitrogen oxides at RT (Rumyantseva et al., 2018; Nasriddinov et al., 2020). Different composites based on porphyrins coated SMOs were developed, presenting promising application in the detection of VOCs (Sivalingam et al., 2013; Ekrami et al., 2018; Magna et al., 2017; Magna et al., 2014).
The authors Chizhov et al. (2016) studied the effect of the surface sensibilization of nanocrystalline ZnO, SnO2 and In2O3 with CdSe colloidal quantum dots (QD) on the interaction with NO2 at RT under green light (λ = 525 nm) corresponding to the CdSe QD absorption band. Photogenerated electrons are injected from the QD into the SMOs CB, while photoexcited holes can go into recombination with electrons localized in chemisorbed O2 and NO2 species. Besides, the photogenerated electrons enhances the interaction with the oxidizing gas NO2. The most effective photosensitization was demonstrated for the In2O3-based nanocomposites due to the maximum difference in the position of the energy level of the photoexcited electron at the CdSe QD and the bottom of the In2O3 conduction band.
In addition to all of the above materials, in work Wang et al. (2024) Bi2WO6 nanosheets supported on CuBi2O4 nanorods were used as gas sensors working at 110°C under blue light. Formation of heterojunction allowed increasing sensitivity and selectivity for n-butyl alcohol among 25 kinds of common organic gases. The authors Liu et al. (2024) obtained heterostructures based on mesoporous Cu-BTC MOF (tricarboxylic acid metal organic framework) and Bi2MoO6 nanocrystals. Such materials showed high selectivity when detecting acetone under UV light. Some other materials, like AgMoS2 and PdMoS2 (Rawat et al., 2024), CoyZn1-yFe2O4 (Ravikumar and Sivaperuman, 2024) showed enhanced light-assisted sensing response towards NH3 at RT.
Van der Waals two-dimensional (2D) materials and heterostructures, such as graphene, transition metal dichalcogenides, MXenes also have demonstrated considerable results for gas detection under photoactivation (Joshi et al., 2018; Kumar et al., 2020; Deb et al., 2024). Herein, several examples can confirm their feasible practical application for gas sensing: g-C3N4 modified ZnO composite for CH4 detection (Zhang et al., 2023), Ti3C2Tx/TiO2/graphene composite with sandwich structure for NH3 sensing (Lin et al., 2024), In2O3/rGO composites for NH3 detection in humid conditions (Nasriddinov et al., 2023), Bi2S3/Sb2S3 heterostructure for trace H2S detection (Yu et al., 2024), graphene-wrapped ZnO nanocomposite with enhanced toluene sensing (Huang et al., 2024). Theoretical analysis of the HCHO adsorption behavior on graphenylene-like ZnMgX2 (X = O, S) monolayers showed that this type of interaction results in a strong chemisorption and does not require the presence of dopant (Chang et al., 2024). Significant improvements in gas sensor characteristics by 2D materials may be associated with tunable band gaps, unusual electronic and optical characteristics, formation of p–n heterojunctions, which lead to effective charge separation.
3 Further development prospects
The creation of gas sensors working under photoactivation is a new, actively developing direction, which has a huge potential for the design of breakthrough sensor technologies. The use of photoactivated gas sensors with low power consumption and compact LEDs allows one to create miniature monitoring systems and portable gas analyzers (electronic noses) not only for indoor and outdoor air monitoring, but also in other areas including breath analysis for non-invasive medical diagnostics (Zheng and Cheng, 2019; Righettoni et al., 2015). Such tasks impose strong requirements for sensitivity and stability of gas sensors, since the concentration of gases in the exhaled air lies in the ppb range, and humidity reaches up to 100% (Güntner et al., 2019). Compared with chromatographic and spectroscopic methods, the electronic nose systems are cost-effective and allow for large-scale and rapid research (Park et al., 2019; Hu et al., 2019; Lee SW. et al., 2023; Eranna et al., 2004). Another promising application of the sensors is robotics, which will be controlled by artificial intelligence system (Chen et al., 2019). Robots similar to humans are being created now, so they need to use some kind of sensor organs close to human ones (including an artificial nose), and automatic centralized control of Smart Home systems is no longer possible without such sensors (Park et al., 2019; Kim et al., 2021; Jeong et al., 2020). An energy-efficient gas sensor integrated into an unmanned aerial vehicle (drone) will increase its battery life for monitoring the air condition in industrial cities and hard-to-reach areas with a high level of gas pollution (Rohi et al., 2020). The further works have to be done to integrate the electronic nose and electronic tongue systems into smartphones (Oletic and Bilas, 2013; Hasenfratz et al., 2012; Aydogmus et al., 2023). The general trend in the development of new sensors and organ-on-a-chip technology is to make them more and more miniature and energy-efficient (Majhi et al., 2021a; Suh et al., 2021; Burgués and Marco, 2018; Leung et al., 2022; Low et al., 2021; Ingber, 2022).
However, an understanding of the effect of light irradiation on the SMOs sensor signal has been attained only in the case of oxidizing gases (NO2, O3) detection, while the vast majority of target substances belong to reducing gases of various chemical nature. To achieve the necessary characteristics of photoactivated sensors, fundamental research is needed: development of new materials with high gas sensitivity at close to room temperature under UV or visible light; investigation of the reactivity of photo- and gas-sensitive materials in interaction with reducing gases; investigation of the sensor properties under conditions that meet the real practical problems of air monitoring.
4 Concluding remarks
In summary, this review discussed recent progress in light-activated gas sensors and illustrated that significant tasks still need to be done to address the shortcomings in the future. Wide gap semiconductor oxides can be used as a functional structure, which provides economic advantages, mass production and high stability. On the other hand, the strategy of replacing thermal activation with light irradiation realizes not only high-performance gas detection at close to room temperature, but also facilitates the development and production of portable, integrated, flexible and multifunctional sensing devices and IoT applications (Nikolic et al., 2020; Soler-Fernández et al., 2024; Cho et al., 2018). However, there are still some limitations and disadvantages in design and manufacture of light-activated composites for gas sensor systems. These pitfalls must be taken into account before a full-scale transition to a new technological production: the lifetime of the sensitive layer, especially organic dyes that tend to fade under the influence of light over the time; selectivity towards certain gases; stability in a humid atmosphere; the possibility of regenerating the sensitive layer without heating.
The negative effects of humidity can be eliminated by using porous membrane materials, zeolites, metal-organic frameworks. Such passive filters operate on a dimensional effect; pores of a certain size can only allow certain gas molecules to pass through or interact with analytical gases using the “guest-host” mechanism. Modification of organic dye molecules to obtain more stable and effective structures or its encapsulation can enhance their persistence. The use of catalytic overlayers, different additives and modifiers can further increase selectivity for detection of certain group of gases due to electronic and chemical sensitization effects. These approaches are expected to improve sensor characteristics for gas detection at room temperature and expand the scope of practical application.
Author contributions
AN: Conceptualization, Writing–original draft, Writing–review and editing. RZ: Writing–original draft, Writing–review and editing. MR: Conceptualization, Writing–original draft, Writing–review and editing.
Funding
The author(s) declare that financial support was received for the research, authorship, and/or publication of this article. This research was funded by a grant from the Ministry of Science and Higher Education of the Russian Federation, grant number 075-15-2024-646.
Conflict of interest
The authors declare that the research was conducted in the absence of any commercial or financial relationships that could be construed as a potential conflict of interest.
Generative AI statement
The author(s) declare that no Generative AI was used in the creation of this manuscript.
Publisher’s note
All claims expressed in this article are solely those of the authors and do not necessarily represent those of their affiliated organizations, or those of the publisher, the editors and the reviewers. Any product that may be evaluated in this article, or claim that may be made by its manufacturer, is not guaranteed or endorsed by the publisher.
References
Anothainart, K., Burgmair, M., Karthigeyan, A., Zimmer, M., and Eisele, I. (2003). Light enhanced NO2 gas sensing with tin oxide at room temperature: conductance and work function measurements. Sens. Actuators B 93, 580–584. doi:10.1016/S0925-4005(03)00220-X
Aydogmus, H., Hu, M., Ivancevic, L., Frimat, J. P., van den Maagdenberg, AMJM, Sarro, P. M., et al. (2023). An organ-on-chip device with integrated charge sensors and recording microelectrodes. Sci. Rep. 13, 8062. doi:10.1038/s41598-023-34786-5
Barsan, N., Koziej, D., and Weimar, U. (2007). Metal oxide-based gas sensor research: how to? Sens. Actuators B 121, 18–35. doi:10.1016/j.snb.2006.09.047
Barsan, N., Schweizer-Berberich, M., and Göpel, W. (1999). Fundamental and practical aspects in the design of nanoscaled SnO2 gas sensors: a status report. Fresenius J. Anal. Chem. 365, 287. doi:10.1007/s002160051490
Barsan, N., and Weimar, U. (2001). Conduction model of metal oxide gas sensors. J. Electroceram 7, 143–167. doi:10.1023/A:1014405811371
Basova, T. V., Mikhaleva, N. S., Hassan, A. K., and Kiselev, V. G. (2016). Thin films of fluorinated 3d-metal phthalocyanines as chemical sensors of ammonia: an optical spectroscopy study. Sens. Actuators B 227, 634–642. doi:10.1016/j.snb.2015.12.079
Bignozzi, C. A., Agazzi, R., Schoonover, J. R., Meyer, G. J., and Scandola, F. (1995). Photosensitization of wide bandgap semiconductors with antenna molecules. Sol. Energy Mater Sol. Cells 38, 187–198. doi:10.1016/0927-0248(94)00225-8
Boukhvalov, D. W., Paolucci, V., D’Olimpio, G., Cantalini, C., and Politano, A. (2021). Chemical reactions on surfaces for applications in catalysis, gas sensing, adsorption-assisted desalination and Li-ion batteries: opportunities and challenges for surface science. Phys. Chem. Chem. Phys. 23, 7541–7552. doi:10.1039/D0CP03317K
Breisinger, M. (2012). Greenhouse gas assessment emissions methodology publications. Available at: https://publications.iadb.org/publications/english/document/Greenhouse-Gas-Assessment-Emissions-Methodology.pdf (Accessed December 01, 2024)
Burgués, J., and Marco, S. (2018). Low power operation of temperature-modulated metal oxide semiconductor gas sensors. Sensors 18, 339. doi:10.3390/s18020339
Chai, H., Zheng, Z., Liu, K., Xu, J., Wu, K., Luo, Y., et al. (2022). Stability of metal oxide semiconductor gas sensors: a review. IEEE Sens. J. 22, 5470–5481. doi:10.1109/JSEN.2022.3148264
Chang, Y. H. R., Abdullahi, Y. Z., Tuh, M. H., and Lim, T. L. (2024). Ultraviolet enhanced inorganic graphenylene-like ZnMgX2 (X=O, S) for sensitive and reversible detection of toxic formaldehyde at room temperature: a first-principles study. Surf. Interfaces 44, 103722. doi:10.1016/j.surfin.2023.103722
Chen, H., Liu, Y., Xie, C., Wu, J., Zeng, D., and Liao, Y. (2012). A comparative study on UV light activated porous TiO2 and ZnO film sensors for gas sensing at room temperature. Ceram. Int. 38, 503–509. doi:10.1016/j.ceramint.2011.07.035
Chen, Z., Chen, Z., Song, Z., Ye, W., and Fan, Z. (2019). Smart gas sensor arrays powered by artificial intelligence. J. Semicond. 40, 111601. doi:10.1088/1674-4926/40/11/111601
Chizhov, A., Kutukov, P., Astafiev, A., and Rumyantseva, M. (2023). Photoactivated processes on the surface of metal oxides and gas sensitivity to oxygen. Sensors 23, 1055. doi:10.3390/s23031055
Chizhov, A., Kutukov, P., Gulin, A., Astafiev, A., and Rumyantseva, M. (2022). UV-activated NO2 gas sensing by nanocrystalline ZnO: mechanistic insights from mass spectrometry investigations. Chemosensors 10, 147. doi:10.3390/chemosensors10040147
Chizhov, A., Rumyantseva, M., and Gaskov, A. (2021). Light activation of nanocrystalline metal oxides for gas sensing: principles, achievements, challenges. Nanomaterials 11, 892. doi:10.3390/nano11040892
Chizhov, A. S., Rumyantseva, M. N., Vasiliev, R. B., Filatova, D. G., Drozdov, K. A., Krylov, I. V., et al. (2016). Visible light activation of room temperature NO2 gas sensors based on ZnO, SnO2 and In2O3 sensitized with CdSe quantum dots. Thin Solid Films 618, 253–262. doi:10.1016/j.tsf.2016.09.029
Cho, I., Cho, M., Kang, K., and Park, I. (2018). “Photo-activated NO2 sensors with 1-D nanomaterials integrated with micro-LED for high irradiation efficiency,” Measur. Scien. and Metro. 167–168. doi:10.5162/IMCS2018/AP1.6
Cho, I., Sim, Y. S., Cho, M., Cho, Y.-H., and Park, I. (2020). Monolithic micro light-emitting diode/metal oxide nanowire gas sensor with microwatt-level power consumption. ACS Sens. 5, 563–570. doi:10.1021/acssensors.9b02487
Cho, M., and Park, I. (2016). Recent trends of light-enhanced metal oxide gas sensors: review. J. Sens. Sci. Technol. 25, 103–109. doi:10.5369/JSST.2016.25.2.103
Comini, E., Faglia, G., and Sberveglieri, G. (2001). UV light activation of tin oxide thin films for NO2 sensing at low temperatures. Sens. Actuators B 78, 73–77. doi:10.1016/S0925-4005(01)00796-1
Das, S., Mojumder, S., Saha, D., and Pal, M. (2022). Influence of major parameters on the sensing mechanism of semiconductor metal oxide based chemiresistive gas sensors: a review focused on personalized healthcare. Sens. Actuators B 352, 131066. doi:10.1016/j.snb.2021.131066
Deb, S., Mondal, A., and Ashok Kumar Reddy, Y. (2024). Review on development of metal-oxide and 2-D material based gas sensors under light-activation. Curr. Opin. Solid State Mater Sci. 30, 101160. doi:10.1016/j.cossms.2024.101160
Ekrami, M., Magna, G., Emam-Djomeh, Z., Yarmand, M. S., Paolesse, R., and Di Natale, C. (2018). Porphyrin-functionalized zinc oxide nanostructures for sensor applications. Sensors 18, 2279. doi:10.3390/s18072279
Eranna, G., Joshi, B. C., Runthala, D. P., and Gupta, R. P. (2004). Oxide materials for development of integrated gas sensors - a comprehensive review. Crit. Rev. Solid State Mater Sci. 29, 111–188. doi:10.1080/10408430490888977
Espid, E., and Taghipour, F. (2017). UV-LED photo-activated chemical gas sensors: a review. Crit. Rev. Solid State Mater Sci. 42, 416–432. doi:10.1080/10408436.2016.1226161
Geng, X., Zhang, C., and Debliquy, M. (2016). Cadmium sulfide activated zinc oxide coatings deposited by liquid plasma spray for room temperature nitrogen dioxide detection under visible light illumination. Ceram. Int. 42, 4845–4852. doi:10.1016/j.ceramint.2015.11.170
Gogurla, N., Sinha, A. K., Santra, S., Manna, S., and Ray, S. K. (2014). Multifunctional Au-ZnO plasmonic nanostructures for enhanced UV photodetector and room temperature NO sensing devices. Sci. Rep. 4, 6483. doi:10.1038/srep06483
Güntner, A. T., Abegg, S., Königstein, K., Gerber, P. A., Schmidt-Trucksäss, A., and Pratsinis, S. E. (2019). Breath sensors for health monitoring. ACS Sens. 4, 268–280. doi:10.1021/acssensors.8b00937
Hasenfratz, D., Saukh, O., Sturzenegger, S., and Thiele, L. (2012). Participatory air pollution monitoring using smartphones. IPSN’12 - Proc. 11th Int. Conf. Inf. Process Sens. Netw., 1–5.
Hu, W., Wan, L., Jian, Y., Ren, C., Jin, K., Su, X., et al. (2019). Electronic noses: from advanced materials to sensors aided with data processing. Adv. Mater Technol. 4, 1800488. doi:10.1002/admt.201800488
Huang, Q., Wu, J., Zeng, D., and Zhou, P. (2024). Graphene-wrapped ZnO nanocomposite with enhanced room-temperature photoactivated toluene sensing properties. Materials 17, 1009. doi:10.3390/ma17051009
Ingber, D. E. (2022). Human organs-on-chips for disease modelling, drug development and personalized medicine. Nat. Rev. Genet. 23, 467–491. doi:10.1038/s41576-022-00466-9
Jeong, S. Y., Kim, J. S., and Lee, J. H. (2020). Rational design of semiconductor-based chemiresistors and their libraries for next-generation artificial olfaction. Adv. Mat. 32, 2002075. doi:10.1002/adma.202002075
Ji, H., Zeng, W., and Li, Y. (2019). Gas sensing mechanisms of metal oxide semiconductors: a focus review. Nanoscale 11, 22664–22684. doi:10.1039/C9NR07699A
Joshi, N., Hayasaka, T., Liu, Y., Liu, H., Oliveira, O. N., and Lin, L. (2018). A review on chemiresistive room temperature gas sensors based on metal oxide nanostructures, graphene and 2D transition metal dichalcogenides. Microchim. Acta. 185, 213. doi:10.1007/s00604-018-2750-5
Kaushik, A., Kumar, R., Arya, S. K., Nair, M., Malhotra, B. D., and Bhansali, S. (2015). Organic-Inorganic hybrid nanocomposite-based gas sensors for environmental monitoring. Chem. Rev. 115, 4571–4606. doi:10.1021/cr400659h
Khurgin, J. B. (2019). Hot carriers generated by plasmons: where are they generated and where do they go from there? Faraday Discuss. 214, 35–58. doi:10.1039/C8FD00200B
Kim, S., Brady, J., Al-Badani, F., Yu, S., Hart, J., Jung, S., et al. (2021). Nanoengineering approaches toward artificial nose. Front. Chem. 9, 629329. doi:10.3389/fchem.2021.629329
Kim, Y., Smith, J. G., and Jain, P. K. (2018). Harvesting multiple electron-hole pairs generated through plasmonic excitation of Au nanoparticles. Nat. Chem. 10, 763–769. doi:10.1038/s41557-018-0054-3
Korotcenkov, G., Han, S. H., and Cho, B. K. (2013). Material design for metal oxide chemiresistive gas sensors. J. Sens. Sci. Technol. 22, 1–17. doi:10.5369/JSST.2013.22.1.1
Kumar, R., Liu, X., Zhang, J., and Kumar, M. (2020). Room-temperature gas sensors under photoactivation: from metal oxides to 2D materials. Nano-Micro Lett. 12:164. doi:10.1007/s40820-020-00503-4
Kumar, R. R., Murugesan, T., Dash, A., Hsu, C. H., Gupta, S., Manikandan, A., et al. (2021). Ultrasensitive and light-activated NO2 gas sensor based on networked MoS2/ZnO nanohybrid with adsorption/desorption kinetics study. Appl. Surf. Sci. 536, 147933. doi:10.1016/j.apsusc.2020.147933
Lee, D. H., and Yoo, H. (2022). Recent advances in Photo−Activated chemical sensors. Sensors 22, 9228. doi:10.3390/s22239228
Lee, K., Cho, I., Kang, M., Jeong, J., Choi, M., Woo, K. Y., et al. (2023a). Ultra-low-power E-nose system based on multi-micro-LED-integrated, nanostructured gas sensors and deep learning. ACS Nano 17, 539–551. doi:10.1021/acsnano.2c09314
Lee, S. W., Kim, B. H., and Seo, Y. H. (2023b). Olfactory system-inspired electronic nose system using numerous low-cost homogenous and hetrogenous sensors. PLoS One 18, e0295703. doi:10.1371/journal.pone.0295703
Leung, C. M., de Haan, P., Ronaldson-Bouchard, K., Kim, G. A., Ko, J., Rho, H. S., et al. (2022). A guide to the organ-on-a-chip. Nat. Rev. Methods Prim. 2, 33. doi:10.1038/s43586-022-00118-6
Lin, M., Huang, Y., Lei, Z., Liu, N., Huang, C., Qi, F., et al. (2024). UV-promoted NH3 sensor based on Ti3C2Tx/TiO2/graphene sandwich structure with ultrasensitive RT sensing performances for human health detection. Sens. Actuators B 410, 135681. doi:10.1016/j.snb.2024.135681
Linic, S., Christopher, P., and Ingram, D. B. (2011). Plasmonic-metal nanostructures for efficient conversion of solar to chemical energy. Nat. Mater 10, 911–921. doi:10.1038/nmat3151
Liu, W., Tkatchenko, A., and Scheffler, M. (2014). Modeling adsorption and reactions of organic molecules at metal surfaces. Acc. Chem. Res. 47, 3369–3377. doi:10.1021/ar500118y
Liu, Z., Lv, H., Li, S., Sun, Y., Chen, X., and Xu, Y. (2024). Dual regulation of hierarchical porosity and heterogeneous interfaces in Cu-BTC/Bi2MoO6 for thermally-driven and UV-light-activated selective acetone sensing. J. Mater Chem. A 12, 6318–6328. doi:10.1039/D4TA00080C
Low, L. A., Mummery, C., Berridge, B. R., Austin, C. P., and Tagle, D. A. (2021). Organs-on-chips: into the next decade. Nat. Rev. Drug Discov. 20, 345–361. doi:10.1038/s41573-020-0079-3
Lu, X., Rycenga, M., Skrabalak, S. E., Wiley, B., and Xia, Y. (2009). Chemical synthesis of novel plasmonic nanoparticles. Annu. Rev. Phys. Chem. 60, 167–192. doi:10.1146/annurev.physchem.040808.090434
Ma, Q., Zhong, C., Ma, J., Ye, C., Zhao, Y., Liu, Y., et al. (2021b). Comprehensive study about the photolysis of nitrates on mineral oxides. Environ. Sci. Technol. 55, 8604–8612. doi:10.1021/acs.est.1c02182
Ma, Z., Wang, Z., and Gao, L. (2021a). Light-assisted enhancement of gas sensing property for micro-nanostructure electronic device: a mini review. Front. Chem. 9, 811074. doi:10.3389/fchem.2021.811074
Magna, G., Catini, A., Kumar, R., Palmacci, M., Martinelli, E., Paolesse, R., et al. (2017). Conductive photo-activated porphyrin-ZnO nanostructured gas sensor array. Sensors 17, 747. doi:10.3390/s17040747
Magna, G., Sivalingam, Y., Martinelli, E., Pomarico, G., Basoli, F., Paolesse, R., et al. (2014). The influence of film morphology and illumination conditions on the sensitivity of porphyrins-coated ZnO nanorods. Anal. Chim. Acta 810, 86–93. doi:10.1016/j.aca.2013.12.008
Majhi, S. M., Mirzaei, A., Kim, H. W., Kim, S. S., and Kim, T. W. (2021a). Recent advances in energy-saving chemiresistive gas sensors: a review. Nano Energy 79, 105369. doi:10.1016/j.nanoen.2020.105369
Majhi, S. M., Mirzaei, A., Navale, S., Kim, H. W., and Kim, S. S. (2021b). Boosting the sensing properties of resistive-based gas sensors by irradiation techniques: a review. Nanoscale 13, 4728–4757. doi:10.1039/D0NR08448D
Mall, K., Shukla, A., Tripathi, U. N., and Mishra, M. (2018). A study of detection mechanism of metal oxide gas sensor under UV radiation. J. Ultra Sci. Phys. Sci. 30, 57–63. doi:10.22147/jusps-B/300403
Manjavacas, A., Liu, J. G., Kulkarni, V., and Nordlander, P. (2014). Plasmon-induced hot carriers in metallic nanoparticles. ACS Nano 8, 7630–7638. doi:10.1021/nn502445f
Mishra, S., Ghanshyam, C., Ram, N., Bajpai, R. P., and Bedi, R. K. (2004). Detection mechanism of metal oxide gas sensor under UV radiation. Sens. Actuators B 97, 387–390. doi:10.1016/j.snb.2003.09.017
Mun, Y., Park, S., An, S., Lee, C., and Kim, H. W. (2013). NO2 gas sensing properties of Au-functionalized porous ZnO nanosheets enhanced by UV irradiation. Ceram. Int. 39, 8615–8622. doi:10.1016/j.ceramint.2013.04.035
Nam, G. B., Ryu, J. El, Eom, T. H., Kim, S. J., Suh, J. M., Lee, S., et al. (2024). Real-time tunable gas sensing platform based on SnO2 nanoparticles activated by blue micro-light-emitting diodes. Nano-Micro Lett. 16, 261. doi:10.1007/s40820-024-01486-2
Nasriddinov, A., Rumyantseva, M., Shatalova, T., Tokarev, S., Yaltseva, P., Fedorova, O., et al. (2020). Organic-inorganic hybrid materials for room temperature light-activated sub-ppm NO detection. Nanomaterials 10, 70. doi:10.3390/nano10010070
Nasriddinov, A., Shatalova, T., Maksimov, S., Li, X., and Rumyantseva, M. (2023). Humidity effect on low-temperature NH3 sensing behavior of In2O3/rGO composites under UV activation. Sensors 23, 1517. doi:10.3390/s23031517
National Research Council (2012). Acute exposure guideline levels for selected airborne chemicals: volume 11. Washington, DC: The National Academies Press. doi:10.17226/13374
Neri, G. (2015). First fifty years of chemoresistive gas sensors. Chemosensors 3, 1–20. doi:10.3390/chemosensors3010001
Nikolic, M. V., Milovanovic, V., Vasiljevic, Z. Z., and Stamenkovic, Z. (2020). Semiconductor gas sensors: materials, technology, design, and application. Sensors 20, 6694. doi:10.3390/s20226694
Oletic, D., and Bilas, V. (2013). Empowering smartphone users with sensor node for air quality measurement. J. Phys. Conf. Ser. 450, 012028. doi:10.1088/1742-6596/450/1/012028
Panayotov, D. A., and Morris, J. R. (2016). Surface chemistry of Au/TiO2: thermally and photolytically activated reactions. Surf. Sci. Rep. 71, 77–271. doi:10.1016/j.surfrep.2016.01.002
Paolesse, R., Nardis, S., Monti, D., Stefanelli, M., and Di Natale, C. (2017). Porphyrinoids for chemical sensor applications. Chem. Rev. 117, 2517–2583. doi:10.1021/acs.chemrev.6b00361
Park, S. Y., Kim, Y., Kim, T., Eom, T. H., Kim, S. Y., and Jang, H. W. (2019). Chemoresistive materials for electronic nose: progress, perspectives, and challenges. InfoMat 1, 289–316. doi:10.1002/inf2.12029
Peng, L., Qin, P., Zeng, Q., Song, H., Lei, M., Mwangi, J. J. N., et al. (2011). Improvement of formaldehyde sensitivity of ZnO nanorods by modifying with Ru(dcbpy)2(NCS)2. Sens. Actuators B 60, 39–45. doi:10.1016/j.snb.2011.07.008
Prades, J. D., Jimenez-Diaz, R., Hernandez-Ramirez, F., Barth, S., Cirera, A., Romano-Rodriguez, A., et al. (2009). Equivalence between thermal and room temperature UV light-modulated responses of gas sensors based on individual SnO2 nanowires. Sens. Actuators B 140, 337–341. doi:10.1016/j.snb.2009.04.070
Ravikumar, T., and Sivaperuman, K. (2024). Fabrication of bare and cobalt-doped ZnFe2O4 thin film as NH3 gas sensor with enhanced response through UV-light illumination. Mater Today Chem. 38, 102049. doi:10.1016/j.mtchem.2024.102049
Rawat, S., Bamola, P., Negi, S., Karishma, N., Rani, C., Dangwal, S., et al. (2024). Light-assisted AgMoS2 and PdMoS2 hybrid gas sensors for room-temperature detection of ammonia. ACS Appl. Nano Mater 7, 746–755. doi:10.1021/acsanm.3c04787
Righettoni, M., Amann, A., and Pratsinis, S. E. (2015). Breath analysis by nanostructured metal oxides as chemo-resistive gas sensors. Mater Today 18, 163–171. doi:10.1016/j.mattod.2014.08.017
Rohi, G., Ejofodomi, O., and Ofualagba, G. (2020). Autonomous monitoring, analysis, and countering of air pollution using environmental drones. Heliyon 6, 3252. doi:10.1016/j.heliyon.2020.e03252
Rossi, T. P., Shegai, T., Erhart, P., and Antosiewicz, T. J. (2019). Strong plasmon-molecule coupling at the nanoscale revealed by first-principles modeling. Nat. Commun. 10, 3336. doi:10.1038/s41467-019-11315-5
Rumyantseva, M., Nasriddinov, A., Vladimirova, S., Tokarev, S., Fedorova, O., Krylov, I., et al. (2018). Photosensitive organic-inorganic hybrid materials for room temperature gas sensor applications. Nanomaterials 8, 671. doi:10.3390/nano8090671
Santhanam, K. S. V., and Ahamed, N. N. N. (2018). Greenhouse gas sensors fabricated with new materials for climatic usage: a review. ChemEngineering 2, 38. doi:10.3390/chemengineering2030038
Saura, J. (1994). Gas-sensing properties of SnO2 pyrolytic films subjected to ultraviolet radiation. Sens. Actuators B 17 (3), 211–214. doi:10.1016/0925-4005(93)00874-X
Scandola, F., Argazzi, R., Bignozzi, C. A., and Indelli, M. T. (1994). Photoinduced energy and electron transfer in inorganic covalently linked systems. J. Photochem Photobiol. 82:191. 202. doi:10.1016/1010-6030(94)02016-7
Šetka, M., Claros, M., Chmela, O., and Vallejos, S. (2021). Photoactivated materials and sensors for NO2 monitoring. J. Mater Chem. C 9, 16804–16827. doi:10.1039/D1TC04247E
Sivalingam, Y., Magna, G., Pomarico, G., Catini, A., Martinelli, E., Paolesse, R., et al. (2013). The light enhanced gas selectivity of one-pot grown porphyrins coated ZnO nanorods. Sens. Actuators B 188, 475–481. doi:10.1016/j.snb.2013.07.044
Soler-Fernández, J. L., Casals, O., Fàbrega, C., Li, H., Diéguez, A., Daniel Prades, J. D., et al. (2024). A verilog-A model for a light-activated semiconductor gas sensor. IEEE Sens. J. 24, 22530–22538. doi:10.1109/JSEN.2024.3407651
Srinivasan, P., Ezhilan, M., Kulandaisamy, A. J., Babu, K. J., and Rayappan, J. B. B. (2019). Room temperature chemiresistive gas sensors: challenges and strategies—a mini review. J. Mater Sci. Mater Electron. 30, 15825–15847. doi:10.1007/s10854-019-02025-1
Staerz, A., Weimar, U., and Barsan, N. (2022). Current state of knowledge on the metal oxide based gas sensing mechanism. Sens. Actuators B 358, 131531. doi:10.1016/j.snb.2022.131531
Suh, J. M., Eom, T. H., Cho, S. H., Kim, T., and Jang, H. W. (2021). Light-activated gas sensing: a perspective of integration with micro-LEDs and plasmonic nanoparticles. Mater Adv. 2, 827–844. doi:10.1039/D0MA00685H
Sun, X., Tang, M., Yu, M., Fan, Y., Qin, C., Cao, J., et al. (2024b). UV-activated CH4 gas sensor based on Pd@Ni/ZnO microspheres. Mater Today Commun. 40, 109551. doi:10.1016/j.mtcomm.2024.109551
Sun, X., Zhang, Y., Wang, Y., Li, M., Qin, C., Cao, J., et al. (2024a). UV-activated AuAg/ZnO microspheres for high-performance methane sensor at room temperature. Ceram. Int. 50, 30552–30559. doi:10.1016/j.ceramint.2024.05.352
Vasiliev, R. B., Babynina, A. V., Maslova, O. A., Rumyantseva, M. N., Ryabova, L. I., Dobrovolsky, A. A., et al. (2013). Photoconductivity of nanocrystalline SnO2 sensitized with colloidal CdSe quantum dots. J. Mater Chem. C 1, 1005–1010. doi:10.1039/C2TC00236A
Wang, C., Yin, L., Zhang, L., Xiang, D., and Gao, R. (2010). Metal oxide gas sensors: sensitivity and influencing factors. Sensors 10, 2088–2106. doi:10.3390/s100302088
Wang, J., Shen, H., Xia, Y., and Komarneni, S. (2021). Light-activated room-temperature gas sensors based on metal oxide nanostructures: a review on recent advances. Ceram. Int. 47, 7353–7368. doi:10.1016/j.ceramint.2020.11.187
Wang, J. C., Ma, H., Shi, W., Li, W., Zhang, Z., Hou, Y., et al. (2024). Designed synthesized step-scheme heterojunction of Bi2WO6 nanosheet supported on CuBi2O4 nanorod with remarkable photo-assisted gas sensing for N-butyl alcohol. J. Environ. Chem. Eng. 12, 112698. doi:10.1016/j.jece.2024.112698
Wang, Y., Wang, B., and Wang, R. (2023). Current status of detection technologies for indoor hazardous air pollutants and particulate matter. Aerosol Air Qual. Res. 23, 230193. doi:10.4209/aaqr.230193
Watanabe, K., Menzel, D., Nilius, N., and Freund, H. J. (2006). Photochemistry on metal nanoparticles. Chem. Rev. 106, 4301–4320. doi:10.1021/cr050167g
White, N. M., and Turner, J. D. (1997). Thick-film sensors: past, present and future. Meas. Sci. Technol. 8, 1–20. doi:10.1088/0957-0233/8/1/002
World Health Organization (2006). Air quality guidelines. Global update 2005. Available at: https://www.who.int/publications/i/item/WHO-SDE-PHE-OEH-06.02.
Xu, F., and Ho, H. P. (2017). Light-activated metal oxide gas sensors: a review. Micromachines 8, 333. doi:10.3390/mi8110333
Yang, Z., Guo, L., Zu, B., Guo, Y., Xu, T., and Dou, X. (2014). CdS/ZnO Core/Shell nanowire-built films for enhanced photodetecting and optoelectronic gas-sensing applications. Adv. Opt. Mater 2, 738–745. doi:10.1002/adom.201400086
Yao, Y., Li, Z., Song, Y., Zhang, Y., and Xie, K. (2024). Atomically dispersed Ag implanted zeolitic imidazolate Frameworks-8 for Visible-Light enhanced plasmonic opto-chemical sensor. Chem. Eng. J. 487, 150769. doi:10.1016/j.cej.2024.150769
Yu, M., Li, J., Yin, D., Zhou, Z., Wei, C., Wang, Y., et al. (2024). Enhanced oxygen anions generation on Bi2S3/Sb2S3 heterostructure by visible light for trace H2S detection at room temperature. J. Hazard Mat. 476, 134932. doi:10.1016/j.jhazmat.2024.134932
Zemke, W. T., Das, G., and Wahl, A. C. (1972). Theoretical determination of the electron affinity of O2 molecule from the binding energy of O2-. Chem. Phys. Lett. 14, 310–314. doi:10.1016/0009-2614(72)80121-0
Zhang, C., Geng, X., Li, J., Luo, Y., and Lu, P. (2017). Role of oxygen vacancy in tuning of optical, electrical and NO2 sensing properties of ZnO1-x coatings at room temperature. Sens. Actuators B 248, 886–893. doi:10.1016/j.snb.2017.01.105
Zhang, C., Wang, J., Olivier, M. G., and Debliquy, M. (2015). Room temperature nitrogen dioxide sensors based on N719-dye sensitized amorphous zinc oxide sensors performed under visible-light illumination. Sens. Actuators B 209, 69–77. doi:10.1016/j.snb.2014.11.090
Zhang, H., Wang, Y., Sun, X., Wang, Y., Li, M., Cao, J., et al. (2023). Enhanced CH4 sensing performances of g-C3N4 modified ZnO nanospheres sensors under visible-light irradiation. Mater Res. Bull. 165, 112290. doi:10.1016/j.materresbull.2023.112290
Zhang, J., Liu, X., Neri, G., and Pinna, N. (2016). Nanostructured materials for room-temperature gas sensors. Adv. Mat. 28, 795–831. doi:10.1002/adma.201503825
Zhang, Q., Xie, G., Xu, M., Su, Y., Tai, H., Du, H., et al. (2018a). Visible light-assisted room temperature gas sensing with ZnO-Ag heterostructure nanoparticles. Sens. Actuators B 259, 269–281. doi:10.1016/j.snb.2017.12.052
Zhang, Y., He, S., Guo, W., Hu, Y., Huang, J., Mulcahy, J. R., et al. (2018b). Surface-plasmon-Driven hot electron photochemistry. Chem. Rev. 118, 2927–2954. doi:10.1021/acs.chemrev.7b00430
Zheng, X. Q., and Cheng, H. Y. (2019). Flexible and stretchable metal oxide gas sensors for healthcare. Sci. China Technol. Sci. 62, 209–223. doi:10.1007/s11431-018-9397-5
Keywords: semiconductor gas sensor, low operating temperature, ultraviolet/visible light illumination, photosensitive materials, light-activation, photo-irradiation, energy consumption
Citation: Nasriddinov A, Zairov R and Rumyantseva M (2025) Light-activated semiconductor gas sensors: pathways to improve sensitivity and reduce energy consumption. Front. Chem. 13:1538217. doi: 10.3389/fchem.2025.1538217
Received: 04 December 2024; Accepted: 06 February 2025;
Published: 25 February 2025.
Edited by:
Wen Zeng, Chongqing University, ChinaReviewed by:
Hyo-Ryoung Lim, Pukyong National University, Republic of KoreaCopyright © 2025 Nasriddinov, Zairov and Rumyantseva. This is an open-access article distributed under the terms of the Creative Commons Attribution License (CC BY). The use, distribution or reproduction in other forums is permitted, provided the original author(s) and the copyright owner(s) are credited and that the original publication in this journal is cited, in accordance with accepted academic practice. No use, distribution or reproduction is permitted which does not comply with these terms.
*Correspondence: Marina Rumyantseva, cm91bUBpbm9yZy5jaGVtLm1zdS5ydQ==