- 1Department of Orthopedics, Renmin Hospital, Wuhan University, Wuhan, China
- 2Department of Mechanical and Aerospace Engineering, Hong Kong University of Science and Technology, Hong Kong, China
- 3The Institute of Technological Sciences, Wuhan University, Wuhan, China
Customized porous structures are widely used in bone tissue engineering as bone substitutes. In natural bone, the bone tissue relies on the blood vessels in the vesicular structure for nutrition supply. When a defect appears, the biomimetic porous scaffold becomes a good candidate for the formation of new blood vessels and helps bone tissue ingrowth. To construct an osteoconductive bone scaffold that is suitable for the defect region, the powder-based three-dimensional printing technology holds great promise, since it can deal with biocompatible, and biodegradable materials. In this mini-review, we introduce several types of powder-based manufacturing technologies and their corresponding printable materials for the construction of porous structures. In addition, some research cases are highlighted to illustrate the recent development of some research directions related to powder-based 3D printed bone scaffolds.
Introduction
The three-dimensional (3D) porous structure has always received considerable attention in different fields, such as materials science and bone tissue engineering, due to its ability to enhance the mechanical properties as well as its excellent biomimetic design as bone scaffolds. For example, when forming this structure inside materials with a high Young's modulus, it can help enhance the deformability of the material system and improve the fracture strain (Wu et al., 2017); based on that, some stretchable devices were made in 2016 (Shasha et al., 2016). When used as an implanted bone scaffold, a porous shape similar to the natural bone with a porosity of 50–90 vol% makes it a good candidate for mechanically supporting cell growth and inducing angiogenesis (Peltola et al., 2008; Senatov et al., 2016). However, this small inner structure with complex geometry could not be realized by traditional manufacturing processes, such as computer numerical control (CNC) machining, before the development of neoteric 3D printing technology. Using neoteric 3D printing technology, a custom structure with different sizes (radii) and shapes of the pores, as well as porosity can be achieved to meet the needs of real applications.
This review paper describes the powder-based 3D printing technologies and biomaterials used for the fabrication of porous structures in tissue engineering. Furthermore, as the 3D printed porous structure is mostly used as implanted scaffolds for bone defects, the recent research status of 3D printed porous scaffolds for bone defect repairing is also included.
Manufacturing Technologies and Printable Biomaterials
Powder-Based 3D Printing Technology
Over the past decades, the development of 3D printing technology has enabled the fabrication of small and complex structured scaffolds, and this technology has significantly improved the treatment of orthopedic diseases. Compared to treatments such as autografts and allografts, the use of 3D printed scaffolds for bone tissue regeneration can be patient-specific and avoid further damage to a potential donor site (Asadi-Eydivand et al., 2016; Ran et al., 2018). Nowadays, 3D printed scaffolds are mostly fabricated by powder-based 3D printing techniques, such as selective laser sintering (SLS), selective laser melting (SLM), and binder inkjet printing (BIP). These three printing methods have some similarities, such as using a roller to roll the powder from the powder supply platform to the fabrication platform and forming the structure layer by layer from bottom to top; however, different methods are used to construct each layer. Therefore, based on the forming methods, it can be categorized into two types, which are laser-induced methods and the binder-induced methods, and the fabrication details of these methods will be introduced in this section.
The laser-induced methods include selective laser sintering (SLS) and selective laser melting (SLM). As shown in Figure 1A, during the SLS process, the material powder is first put on the powder supply platform with a pre-set powder bed temperature below its melting point and is rolled to the fabrication platform. After one layer of powder has been laid down on the platform, the scanner usually employs CO2 and Nd in a YAG laser with different working powers to selectively scan and sinter each powder layer. After repeating this laser scanning process, it can form porous scaffolds based on the STL files (Kuscer and Shen, 2014; Yuan et al., 2018). In SLS, the material powder is sintered but not fully melted to connect; however, in the SLM process, the temperature is high enough to fully melt the powder and fuse it into one metal block with better mechanical compliance. Therefore, the main difference between SLS and SLM relates to different types of materials. SLS can cover plastic, ceramic, and metal alloys without a particular melting point, however, when dealing with pure metal such as titanium, SLM is a good choice.
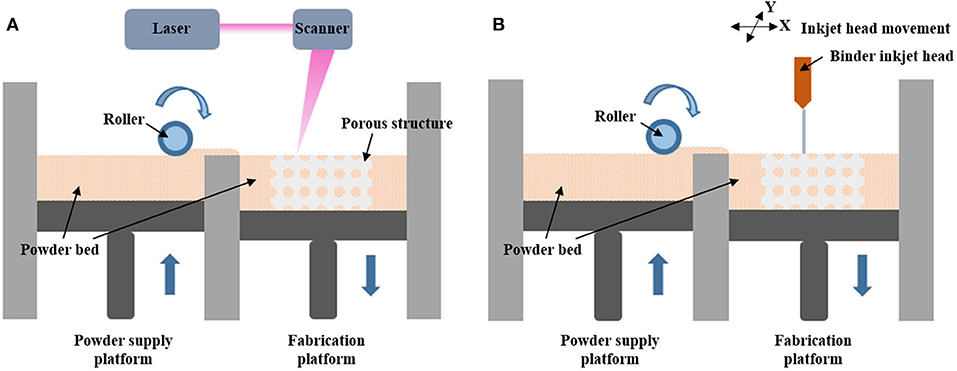
Figure 1. (A) Schematic diagram of the working principle of selective laser sintering and selective laser melting. (B) Schematic diagram of the working principle of binder inkjet printing.
The binder inkjet printing is another way of forming a porous structure, as illustrated in Figure 1B; it shares similar processes of powder preparation with the laser type. Once the roller passes a layer of powder to the fabrication platform in the x-y plane, a binder solution is selectively delivered from the binder inkjet head on the bottom powders to bind the powders in a certain region (Cox et al., 2015). The diluted phosphoric acid-based binder solution is mostly used when fabricating osteoid-like bone scaffolds (Table 1), since it can react with β-tricalcium phosphate (β-TCP) to form bioresorbable calcium phosphates and degrade over time (Bergmann et al., 2010; Tarafder et al., 2012; Inzana et al., 2014). Besides, researchers also used organic based benders like polyvinyl pyrrolidone (PVP) and polyvinyl alcohol (PVA), for example, recent study has shown that 1.0 wt% PVA is most suitable as bender for HA bioceramic bone scaffold (Chai et al., 2020).
Commonly Used 3D Printable Biomaterials
It is widely accepted that the porous structures inside scaffolds are designed to support the cells and tissue during the bone ingrowth during the recovery period, and the scaffold itself degrades over time when the bone is recovered and absorbed by the human body. Based on this, the printable biomaterial powder should comply with certain rules, for example, (1) it should be biocompatible and biodegradable, (2) should not have harmful byproducts after degradation, (3) should provide sufficient mechanical strength. These characteristics are mostly found in some biopolymers, bioceramics, and other composite materials.
One of the most famous classes of materials is calcium phosphates, especially β-tricalcium phosphate (β-TCP), which shares a similar mineral composition with natural bone and can be absorbed over time. As mentioned in printing technology, this material is mostly used together with a phosphoric acid-based binder solution in binder inkjet printing. Taking advantage of its osteoconductive property, TCP scaffolds with different pores sizes have been fabricated and studied both in vitro bone cell and in vivo by implanting scaffolds in male Sprague-Dawley rats (Tarafder et al., 2012). Apart from this, composite granules of β-TCP with other materials, such as biocompatible glass, collagen, and hydroxyapatite, have also been studied and their feasibility as bone scaffolds has been verified by some researchers (Bergmann et al., 2010; Wang et al., 2014). By incorporating collagen, which is of great importance in the formation of the extracellular matrix, the fabricated porous structure can achieve better osteoconductive and osteoinductive properties (Inzana et al., 2014). Most recently, a piezoelectric bone scaffold was fabricated by the barium strontium titanite/β-TCP composite material. This study shows the potential of the piezoelectrical ceramic materials in bone scaffolds fabrication (Tariverdian et al., 2019). Another important material is polycaprolactone (PCL), a biodegradable polymer that remains firmly and non-toxically inside the body for 6 months, then gradually degrades and gets completely absorbed in 2–3 years (Guvendiren et al., 2017); therefore, PCL is an excellent candidate for bone and cartilage scaffolds (Dávila et al., 2016). As shown in Table 1, PCL is the most preferred polymer in the SLS system. Together with hydroxyapatite (HA), porous scaffold based on PCL/HA composite microspheres is fabricated by the SLS technology. Here, the HA is added to substitute the mineral component in the natural bone to help enhance the connection between the cells and the scaffolds (Du et al., 2017). In addition, type II collagen and gelatin are also studied as surface modification materials for PCL scaffold, an in-vitro study shows that using type II collagen for surface modification is more effective than gelatin in the PCL scaffold when stimulating ECM protein secretion (Chen et al., 2014). Apart from TCP and PCL, composite powder particles of Poly(vinyl)alcohol (PVOH) /HA have also been applied in bone scaffolds.
Application as Bone Scaffold
Although the above-mentioned 3D printing technologies allow the fabrication of bone scaffolds, when designing bone scaffolds, other factors that can support cell and tissue growth and blood vessel formation in practical applications should also be considered. One of the major issues to be addressed is how to improve the formation of the highly vascularized tissues when implanted scaffolds inside the natural bone. To evaluate that, some biological testing like in vitro cell culture testing and in vivo osteogenesis evaluation are mostly used and these are also included in Table 1. To address the issue, researchers from the University of Oklahoma found that the presence of the Arg-Gly-Asp (RGD) phage could lead to more vascularized bone tissue growth in vivo without external osteogenic supplements. When the RGD phage is combined with the 3D printed porous structured HA/β-TCP scaffold, more bone forming cells form inside the microporous scaffolds, which help the bone repair process (Wang et al., 2014). Other researchers have also found that using the biocompatible Akermanite (AKM) may be a good choice when AKM powders are added to poly (3-hydroxybutyrate-co-3-hydroxyvalerate; PHBV), which helps speed up the degradation process. These AKM powders, after forming the porous scaffolds, exist both inside and outside the scaffold surface, which improves cell adhesion and strengthens the whole structure, respectively (Diermann et al., 2019). Another issue is the optimization of the porous structure. Researchers suggest that the porosity and pore size can not only affect the degradation of the scaffold material and its mechanical properties, but also control cell seeding processes, such as cell distribution and cell attachment that are vital for tissue growth. For instance, in a study that focuses on the effect of scaffold porosity, the material infill density of several scaffolds ranging from 20 to 80% has been studied. In-vivo cellular testing shows that a 40% infill density with pore size of 0.8 mm is optimal for human adipose-derived stem cells (hASCs) seeding (Temple et al., 2014). In this area, researchers have also discussed the effect of different technical parameters, such as pore size, porosity, layer thickness, etc., which may affect the manufacturing process. Three cylindrical structures with unit cells of a fixed strut size (0.6 mm) with different pore sizes (0.4, 0.6, and 0.8 mm) were designed for fabricated scaffolds with different porosity in a research group in Malaysia. These three structures are then fabricated with different layer thicknesses and powder delivery speeds. The experimental results give the optimal technologic parameters of 89-μm layer thickness and 300-ms layer spread delay time for the highest quality scaffolds (Asadi-Eydivand et al., 2016).
Summary
The advancement of 3D printing technology enables the fabrication of complex porous scaffolds for bone tissue regeneration. In the past decades, bone scaffolds fabricated using different printing technologies from various biocompatible materials have been widely studied. Therefore, in this mini-review, we first summarize the three main powder-based printing methods, namely, SLS, SLM, and binder inkjet printing. Some commonly used printable materials are also included. In addition, a few shortcomings of the porous scaffolds in practical application have been discussed. For the fabrication of good quality scaffolds, different technical parameters of 3D printing machines should be considered. In addition, the porosity and pore size of the bone scaffolds are of great importance for the cell seeding process, which should be carefully designed and tested both in vitro and in vivo experiments. Thus, some research progress related to the above issues has also been reported in this mini-review.
Author Contributions
YZ and YH have drafted the manuscript and ZL, ZW, and XY have checked it for final submission. All authors contributed to the article and approved the submitted version.
Funding
We are greatly appreciate the financial support from the National Natural Science Foundation of China (Grant No. 81802159).
Conflict of Interest
The authors declare that the research was conducted in the absence of any commercial or financial relationships that could be construed as a potential conflict of interest.
References
Asadi-Eydivand, M., Solati-Hashjin, M., Farzad, A., and Abu Osman, N. A. (2016). Effect of technical parameters on porous structure and strength of 3d printed calcium sulfate prototypes. Robot. Comp. Integr. Manuf. 37, 57–67. doi: 10.1016/j.rcim.2015.06.005
Bergmann, C., Markus, L., Wen, Z., Karolina, K., Armin, K., Rainer, T., et al. (2010). 3D printing of bone substitute implants using calcium phosphate and bioactive glasses. J. Europ. Ceramic Soc. 30, 2563–2567. doi: 10.1016/j.jeurceramsoc.2010.04.037
Caravaggi, P., Liverani, E., Leardini, A., Fortunato, A., Belvedere, C., Baruffaldi, F., et al. (2019). CoCr porous scaffolds manufactured via selective laser melting in orthopedics: topographical, mechanical, and biological characterization. J. Biomed. Mater. Res. B Appl. Biomater. 107, 2343–2353. doi: 10.1002/jbm.b.34328
Chai, W., Qinghua, W., Mingming, Y., Kang, J., Yuhong, G., Shengmin, W., et al. (2020). The printability of three water based polymeric binders and their effects on the properties of 3D printed hydroxyapatite bone scaffold. Ceramics Int. 46, 6663–6671. doi: 10.1016/j.ceramint.2019.11.154
Chen, C. H., Lee, M. Y., Shyu, V. B., Chen, Y. C., Chen, C. T., and Chen, J. P. (2014). Surface modification of polycaprolactone scaffolds fabricated via selective laser sintering for cartilage tissue engineering. Mater. Sci. Eng. C 40, 389–397. doi: 10.1016/j.msec.2014.04.029
Cox, S. C., Thornby, J. A., Gibbons, G. J., Williams, M. A., and Mallick, K. K. (2015). 3D printing of porous hydroxyapatite scaffolds intended for use in bone tissue engineering applications. Mater. Sci. Eng. C 47, 237–247. doi: 10.1016/j.msec.2014.11.024
Dávila, J. L., Freitas, M. S., Inforçatti Neto, P., Silveira, Z. C., Silva, J. V. L., and d'Ávila, M. A. (2016). Fabrication of PCL/β-TCP scaffolds by 3D mini-screw extrusion printing. J. Appl. Polym. Sci. 133. doi: 10.1002/app.43031
Diermann, S. H., Lu, M., Dargusch, M., Grøndahl, L., and Huang, H. (2019). Akermanite reinforced PHBV scaffolds manufactured using selective laser sintering. J. Biomed. Mater. Res. Part B Appl. Biomater. 107, 2596–2610. doi: 10.1002/jbm.b.34349
Du, Y., Liu, H., Yang, Q., Wang, S., Wang, J., Ma, J., et al. (2017). Selective laser sintering scaffold with hierarchical architecture and gradient composition for osteochondral repair in rabbits. Biomaterials 137, 37–48. doi: 10.1016/j.biomaterials.2017.05.021
Guvendiren, M., Joseph, M., Rosane, M. D. S., and Joachim, K. (2017). Designing biomaterials for 3D printing. ACS Biomater Sci Eng. 2, 1679–1693. doi: 10.1021/acsbiomaterials.6b00121
Han, C., Li, Y., Wang, Q., Wen, S., Wei, Q., Yan, C., et al. (2018). Continuous functionally graded porous titanium scaffolds manufactured by selective laser melting for bone implants. J. Mech. Behav. Biomed. Mater. 80, 119–127. doi: 10.1016/j.jmbbm.2018.01.013
Inzana, J. A., Olvera, D., Fuller, S. M., Kelly, J. P., Graeve, O. A., Schwarz, E. M., et al. (2014). Biomaterials 3D printing of composite calcium phosphate and collagen scaffolds for bone regeneration. Biomaterials 35, 4026–4034. doi: 10.1016/j.biomaterials.2014.01.064
Kuscer, D., and Shen, J. Z. (2014). Advanced Direct Forming Processes for the Future. Advanced Ceramics for Dentistry. Ljubljiana: Elsevier Inc. doi: 10.1016/B978-0-12-394619-5.00023-7
Lai, Y., Li, Y., Cao, H., Long, J., Wang, X., Li, L., et al. (2019). Osteogenic magnesium incorporated into PLGA/TCP porous scaffold by 3D printing for repairing challenging bone defect. Biomaterials 197, 207–219. doi: 10.1016/j.biomaterials.2019.01.013
Nandi, S. K., Fielding, G., Banerjee, D., Bandyopadhyay, A., and Bose, S. (2018). 3D-Printed β-TCP bone tissue engineering scaffolds: effects of chemistry on in vivo biological properties in a rabbit tibia model. J. Mater. Res 33, 1939–1947. doi: 10.1557/jmr.2018.233
Peltola, S. M., Melchels, F. P., Grijpma, D. W., and Kellomäki, M. (2008). A review of rapid prototyping techniques for tissue engineering purposes. Ann. Med. 40, 268–280. doi: 10.1080/07853890701881788
Ran, Q., Yang, W., Hu, Y., Shen, X., Yu, Y., Xiang, Y., et al. (2018). Osteogenesis of 3D printed porous Ti6Al4V implants with different pore sizes. J. Mech. Behav. Biomed. Mater. 84, 1–11. doi: 10.1016/j.jmbbm.2018.04.010
Senatov, F. S., Niaza, K. V., Zadorozhnyy, M. Y., Maksimkin, A. V., Kaloshkin, S. D., and Estrin, Y. Z. (2016). Mechanical properties and shape memory effect of 3d-printed pla-based porous scaffolds. J. Mech. Behav. Biomed. Mater 57, 139–148. doi: 10.1016/j.jmbbm.2015.11.036
Shasha, D., Ke, Y., and Zhihui, W. (2016). Fabrication of highly stretchable conductors based on 3D printed porous poly(dimethylsiloxane) and conductive carbon nanotubes/ graphene network. Appl. Mater. Interfaces 8, 8–13. doi: 10.1021/acsami.5b10791
Tan, K. H., Chua, C. K., Leong, K. F., Cheah, C. M., Cheang, P., Abu Bakar, M. S., et al. (2003). Scaffold development using selective laser sintering of polyetheretherketone-hydroxyapatite biocomposite blends. Biomaterials 24, 3115–3123. doi: 10.1016/S0142-9612(03)00131-5
Tarafder, S., Balla, V. K., Davies, N. M., Bandyopadhyay, A., and Bose, S. (2012). Microwave-sintered 3D printed tricalcium phosphate scaffolds for bone tissue engineering. J. Tissue Eng. Regen. Med. 7, 631–641. doi: 10.1002/term.555
Tariverdian, T., Ghader, A. B., Milan, P. B., Barzegar-Bafrooei, H., and Mozafari, M. (2019). 3D-printed barium strontium titanate-based piezoelectric scaffolds for bone tissue engineering. Ceramics Int. 45, 14029–14038. doi: 10.1016/j.ceramint.2019.04.102
Temple, J. P., Hutton, D. L., Hung, B. P., Huri, P. Y., Cook, C. A., Kondragunta, R., et al. (2014). Engineering anatomically shaped vascularized bone grafts with HASCs and 3D-printed PCL scaffolds. Soc. Biomater. 102, 1–9. doi: 10.1002/jbm.a.35107
Wang, J., Yang, M., Zhu, Y., Wang, L., Tomsia, A. P., and Mao, C. (2014). Phage nanofi bers induce vascularized osteogenesis in 3D printed bone scaffolds. Adv. Mater. 26, 1–6. doi: 10.1002/adma.201400154
Weißmann, V., Bader, R., Hansmann, H., and Laufer, N. (2016). Influence of the structural orientation on the mechanical properties of selective laser melted Ti6Al4V open-porous scaffolds. Mater. Design 95, 188–197. doi: 10.1016/j.matdes.2016.01.095
Wiria, F. E., Leong, K. F., Chua, C. K., and Liu, Y. (2007). Poly-ε-caprolactone/hydroxyapatite for tissue engineering scaffold fabrication via selective laser sintering. Acta Biomater 3, 1–12. doi: 10.1016/j.actbio.2006.07.008
Wu, S., Zhang, J., Ladani, R. B., Ravindran, A. R., Mouritz, A. P., Kinloch, A. J., et al. (2017). Novel electrically conductive porous PDMS / carbon nano fi ber composites for deformable strain sensors and conductors. ACS Appl. Mater. Interfaces 9:16 doi: 10.1021/acsami.7b00847
Keywords: 3D printing, selective laser sintering, binder inkjet printing, osteochondral scaffold, bone tissue engineering
Citation: Zhao Y, Hou Y, Li Z, Wang Z and Yan X (2020) Powder-Based 3D Printed Porous Structure and Its Application as Bone Scaffold. Front. Mater. 7:150. doi: 10.3389/fmats.2020.00150
Received: 04 March 2020; Accepted: 28 April 2020;
Published: 30 June 2020.
Edited by:
Francesco Baino, Politecnico di Torino, ItalyReviewed by:
Sergey V. Dorozhkin, Independent Researcher, Moscow, RussiaAgata Przekora, Medical University of Lublin, Poland
Copyright © 2020 Zhao, Hou, Li, Wang and Yan. This is an open-access article distributed under the terms of the Creative Commons Attribution License (CC BY). The use, distribution or reproduction in other forums is permitted, provided the original author(s) and the copyright owner(s) are credited and that the original publication in this journal is cited, in accordance with accepted academic practice. No use, distribution or reproduction is permitted which does not comply with these terms.
*Correspondence: Xinxin Yan, eGlueGlueWFuQHdodS5lZHUuY24=
†These authors have contributed equally to this work