- IMT Lille Douai, Institut Mines-Télécom, University of Lille, Energy and Environment Centre, Lille, France
Coming from natural and anthropogenic sources, hydrogen sulfide gas (H2S) is a smelly hazardous substance at the sub-ppm level, which can lead to poisoning deaths at higher concentrations. Researchers have been working for decades to design sensors with sufficient/good/robust metrological properties and good stability in order to monitor and control in real time the risk associated with this gas. Among the devices proposed, chemiresistive sensors based on conductive polymer appear as a good alternative to the most common solutions such as electrochemical and optical sensors. They present various advantages in terms of design (easy fabrication, easy tuning of physical and chemical properties, low cost, etc.) and performances (good sensitivity, good reproducibility, room temperature operation, short response time, etc.). In this review, we summarize the progresses made on conductive polymer sensors dedicated to H2S detection, including the performance of the different materials and sensing mechanisms. Finally, we identify the limitations of these sensors and highlight the most promising approaches to enable the use of these technologies in real-world applications.
Introduction
Corrosive, colorless, water-soluble, and flammable in ambient conditions, hydrogen sulfide (H2S) is a smelly hazardous substance at the sub-ppm level, which can lead to poisoning deaths at higher concentrations (Malone Rubright et al., 2017). The exposure toward this gas is mainly linked to geothermal activity (e.g., crude petroleum, natural gas, hot spring) and organic decomposition from sewers, wastewater treatment plants, landfills, Sargasse seaweeds, etc. It is also produced by the industry as a by-product of paper manufacturing and tanneries or synthetized intentionally for its use as an agricultural disinfectant and an intermediate in sulfuric acid production [(National Research Council (US) Committee on Acute Exposure Guideline Levels, 2010)]. According to the Occupational Safety and Health Administration and the Bureau of Labor Statistics, H2S is one of the most dangerous gases in the workplace (Malone Rubright et al., 2017; Occupational Safety Health Administration, 2020). International public safety organizations have established several exposure limits from 1 ppb to 100 ppm in order to (i) limit the olfactory disturbance from the natural and industrial production of H2S and to (ii) protect the workers from acute and chronic exposures (Malone Rubright et al., 2017). Humans can smell H2S at low concentrations (0.5 to 300 ppb) (Beauchamp et al., 1984), and the gas can produce severe damages such as possible nausea (~2 ppm), loss of smell (~20 ppm), severe lung/nose/throat irritation (~100 ppm), and death (>250 ppm) (Habeeb et al., 2018). Moreover, H2S is present in various and complex environments, in which the humidity as well as the concentration in co-pollutants (e.g., SO2, CO in geothermal activity) are continuously changing (Malone Rubright et al., 2017). Therefore, monitoring equipment working in different ranges of concentration and environmental conditions is needed.
Analytical methods such as gas chromatography and/or spectrometry are commonly used to quantify H2S concentration in air. Possessing high sensitivity toward low concentration, they present many drawbacks such as complex sampling and analyzing processes, high cost, and low temporal resolution or a posteriori measurements (Tranchida et al., 2016; Zoccali et al., 2019). Odor monitoring and safety control demand cost-effective, user-friendly, and continuous real-time measurements. As standardized methods are not fully fulfilling those requirements, many researches have been done to develop fast, portable, and low-cost devices (Llobet et al., 2017). Among the devices developed, chemiresistive, electrochemical, and optical sensors are the most popular in H2S sensing (Pandey et al., 2012; Serban et al., 2014). Even if few sensors have been commercialized, their sensitivity and stability need to be optimized.
Among the H2S sensors in vogue, the chemiresistive sensor is the simplest transducing system (Wong et al., 2020). Translating chemical information in an electrical signal, this device is based on a two-point contact measurement of the resistance of a sensitive material. Metal oxides (MOx) appear as a model sensitive material in gas sensing (Korotcenkov and Cho, 2017; Joshi et al., 2018) and more specifically in H2S sensing (Guo et al., 2015; Mirzaei et al., 2018) given their high sensitivity, fast response, and easy integration in compact electronic devices. Nevertheless, MOx-based sensors present a limited selectivity and a high dependence on relative humidity and generally require a high operating temperature (>100°C).
An alternative to MOx is the use of conductive polymer (CP) as a sensitizing surface. Showing electronic properties of metals and semiconductors while retaining mechanical properties and processing advantages of polymers, CPs have appeared as promising materials in the field of gas sensor in early 1980s (Nylabder et al., 1983). Since then, many works have been done to increase their metrological performances (Bai and Shi, 2007; Fratoddi et al., 2015; Park et al., 2017). Indeed, CPs present the advantages of being easily synthetized and modified through chemical or electrochemical processes, being easily included in low-cost and miniaturized devices, and being gas sensitive at room temperature, but their selectivity toward H2S and their long-term stability need to be enhanced.
The aim of this paper is to give an overview of the roles played by CPs in chemiresistive sensors dedicated to H2S. After a presentation of the mechanism of detection and sensing performances of pure CPs, the discussion focuses on their hybridization with inorganic fillers. Indeed, these materials trying to combine the advantages of CPs and inorganic nano-objects have awakened extensive interest in the last decade (Hangarter et al., 2013; Kaushik et al., 2015; Park et al., 2017; Iqbal and Ahmad, 2018). Mechanisms and performances are addressed for each type of inorganic fillers (i.e., noble metal, MOx, metal chloride, and carbon-based nanostructures). Finally, a cross-comparison of the sensing properties and the potential for future application of the different sensitive surfaces is done in order to highlight the promising approaches and the investigation needed for achieving those technologies employed in the field. The discussion is based on comparative tables (Supplementary Material) ordered by class of material (e.g., pure CPs, noble metal composites, etc.), where the sensing performance of the materials (i.e., limit of detection and response time) and properties allowing their access to real-field application (i.e., selectivity, stability, reversibility, operating temperature, behavior under humidity changes, etc.) are presented.
Sensors Based On Intrinsic Conductive Polymers
Intrinsic CPs are π-conjugated systems presenting a multilevel structure at the scale of the monomers, the chains, and the bulk of the material. This chemical organization makes their properties highly tunable at different scales from combination of different monomers and chain conformation modification by additive to formation of various morphologies at nanoscale/microscale (Skotheim and Reynolds, 2007).
The π-conjugation along the polymer chain allows the formation of delocalized electrons at the origin of the electric conduction of CPs. Actually, electrons in double bonds are free to move along the carbon skeleton given the overlap of the pz orbitals (Dongmin Kang and Jeffrey Snyder, 2017). Due to the presence of imperfections [e.g., localization of charges induced by Peierls distortion (Anderson and Roth, 1994), short conjugation length, weak degree of crystallinity (Le et al., 2017)], this phenomenon provides low conductivity to CPs in neutral state. Dopants are often used to remove or add electrons from the chain to increase conductivity and form p-type and n-type doped CPs, respectively. In p-type doping, the electrons move from the polymer to the dopant (oxidation process), creating holes along the polymer chain. Conversely, the electron density increases in the n-type doped polymer by the movement of the electron from the dopant to the polymer (reduction process) (Le et al., 2017). Thereby, CPs display conductivity which can be modulated by redox reaction or protonation from near-insulator to metallic conductor (Bai and Shi, 2007).
As a nucleophilic (i.e., reducing) gas, H2S is expected to decrease the conductivity of p-type CPs and conversely increase the conductivity of n-type CPs (Wong et al., 2020). However, this classical behavior is observed only in few studies concerning polyaniline (PANI) deposited by thermal evaporation (Agbor et al., 1995), PANI doped in maleic acid with dodecyl hydrogen sulfate studied under nitrogen (Palaniappan and Saravanan, 2010), polycarbazole (Joshi et al., 2014), and polyazomethines studied in air (Patil et al., 2019). Those p-type materials show effectively a decrease in conductivity under H2S (Figure 1A). On the contrary, the other sensors based on pristine CPs display an increasing conductivity in the presence of H2S. In fact, in the presence of water at room temperature, H2S tends to dissociate into H+ and HS− (Agbor et al., 1995). H+ protonates the nitrogen atoms from PANI (Agbor et al., 1995; Dong et al., 2016), polypyrrole (PPy) (Kriván et al., 2000; Garg et al., 2015), or poly(N-propylaniline) (PNPA) (Chabukswar et al., 2013) and leads to an increase in conductivity toward H2S (Figure 1B). The reducing effect of H2S and the dissociation of the gas followed by the protonation of CPs are the two mechanisms at the origin of the resistance modulation of the H2S sensors based on CPs exclusively.
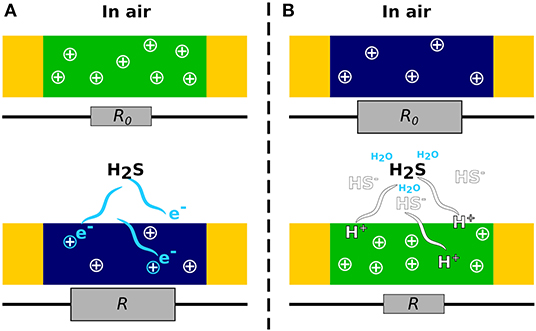
Figure 1. Mechanisms of H2S detection by CPs. (A) The reducing gas H2S induces a reduction of the major charge carrier (holes, +) resulting in an increase in CP resistance (R0 < R). (B) In the presence of water, H2S releases protons, which increase the charge carrier in the polymer and thus decrease the resistivity (R0 > R).
In chemiresistive sensors, the presence of gaseous analyte induces changes in electronic conductivity of a sensitive surface. Therefore, the sensing performance depends on (i) the interaction between the gas and the sensitive material and on (ii) the charge transport (i.e., capacity to transmit the changes to the electrodes) (Park et al., 2017). Each property of the material affecting one of these two parameters will alter the sensing performance of the device.
Given the mechanism of action of H2S, the interactions of gas/polymer are mainly driven by the number of active sites (i.e., redox groups or atoms able to receive proton) capable of interacting with the gas. Beside the molecular structure of the polymer chain, which delimits the total number of active sites, the morphology controls the accessibility to the sites. This parameter can influence the sensitivity as well as the response time of the sensor. Indeed, an open structure (e.g., a porous material) facilitates the adsorption/desorption of the gas in the polymer. For example, the porous agglomerate of nanoparticles (50–100 nm) of PNPA insures a high surface-to-volume ratio leading to the best response from pure-CP sensor toward H2S, with a modulation of −25% of the resistance at 500 ppb and a response time lower than 60 s at 100 ppm (Chabukswar et al., 2013).
Then, the charge transport in CPs is achieved by two mechanisms, the intra-chain transport (i.e., along the chain) and the inter-chain transport, which are both influenced by many factors. The intra-chain transport is fast and localized along the conjugated backbone. It depends mainly on the charge carrier and the conjugation length (Kivelson and Heeger, 1988; Phillips and Wu, 1991). The inter-chain is based on hopping or tunneling of the charge carrier and depends mainly on the conformation of the chain and their organization, which can influence the distance between chains and the degree of crystallinity (Hangarter et al., 2013). Therefore, the disorder of the structure of the polymer can affect its sensing performance.
In the case of H2S detection by CPs, two parameters have shown a large impact on the performance of the sensor: the dopant and the process. The dopant is known to affect the charge transport and the morphology (Krukiewicz and Katunin, 2016). Thus, the size and nature of the dopant can influence the sensing performance of the CPs and their stability. For example, PANI doped with hydrochloride acid (HCl) has a response five times higher than the same material doped with sulfosalicylic acid (SSA) tested toward 100 ppm of H2S (Dong et al., 2016). SSA anions being bigger, they lead to larger chain spacing. The inter-chain transport is less effective, resulting in a lower activity. Moreover, PANI–HCl is less stable over 50 days. Small anions (as HCl) are known to be less stable (Li and Wan, 1999). They are more likely to migrate and volatilize from the CPs, leading to a drift of the resistance with time. Moreover, functional groups, such as the presence of both polar and non-polar groups on SSA, can increase the decomposition temperature (Dong et al., 2016). Those two parameters influence directly the stability of CPs.
Besides influencing the morphology of the sensitive surface, the process of fabrication can affect even the mechanism of variation of the conductivity of CPs. As illustration, PANI in emeraldine base form deposited on Au-IDE by spin-coating (1 μm thick) or thermal evaporation (210 nm) shows, respectively, an reversible increase or an irreversible decrease in the conductivity in the presence of H2S under dry nitrogen (Agbor et al., 1995). The increase in the conductivity from the spin-coated film is due to the protonation of PANI when H2S dissociates into H+ and HS− in contact with water from the film, whereas in the case of thermal evaporation, the film does not contain water and H2S acts as a reducing gas decreasing the conductivity of the film.
CPs have shown their ability to detect H2S from 0.5 to 1,000 ppm. They present the advantage of having a good sensitivity at room temperature and of being highly tunable chemically and physically, giving rise to many optimization approaches. However, as reported in Supplementary Table 1, intrinsic CPs dedicated to H2S sensing are often instable and present a low selectivity. Each reducing or oxidizing gas can affect differently their resistance depending on their mutual affinities. For example, PPy nanowires present an interesting 53% resistance decrease in contact with 200 ppm H2S but they also suffer a larger increase in resistance (143%) in the presence of ammonia (Garg et al., 2015). In order to overcome those main drawbacks, many works dedicated to H2S sensing by CPs are a focus on the hybridization of those materials with inorganic fillers.
Hybrids Conductive Polymer Sensors: Organic-Inorganic Composites
Noble Metal Composites
Noble metals are often used in MOx sensors as sensitizing agent (Ramgir et al., 2013). Indeed, they provide additional absorption sites, promoting a chemical reaction occurring on the surface (chemisorption, redox). This strategy has been applied to CPs hybridized by surface functionalization or bulk inclusion of noble metal nanoparticles (NPs) or clusters in order to increase the sensitivity and/or selectivity toward H2S gas. PANI, PPy, polycarbazole, and polyimide have been used as a matrix when Ag and Au have been used as metallic additives (Supplementary Table 2).
The mechanisms at the origin of the sensing performance of polymer/metal hybrids are not perfectly understood. As shown in Figure 2, the metallic particles can have two effects on the response of CPs toward H2S. Firstly, they present high affinity with analytes carrying sulfur atoms, which facilitates the interaction gas/material (Joshi et al., 2014). Indeed, by reacting with the gas, the metallic items release protons, which dope the CP and induce an increase in conductivity. For example, in the PANI-AuNP system, the gas reacts with gold to form AuS and H+, leading to an enhancement in the doping level of PANI (Shirsat et al., 2009; Liu et al., 2012). Secondly, the presence of metallic particles in p-type CPs induces the formation of nano-Schottky junctions. At the interface between the two components of the material, the difference in work function between the metal and the organic semiconductor prevents the transfer of electron, resulting in an excess of negative charge at the surface of the particles and the formation of a depletion region (Joshi et al., 2014; Kaushik et al., 2015). Changes in the width of the depletion region modulate the conductivity of the sensing material by changing the resistance but also the capacitance of the material (Joshi et al., 2014; Mekki et al., 2014). The properties of the Schottky junctions are highly sensitive to adsorption phenomena, and their presence amplifies the response toward gas adsorbed, resulting in enhanced sensitivity.
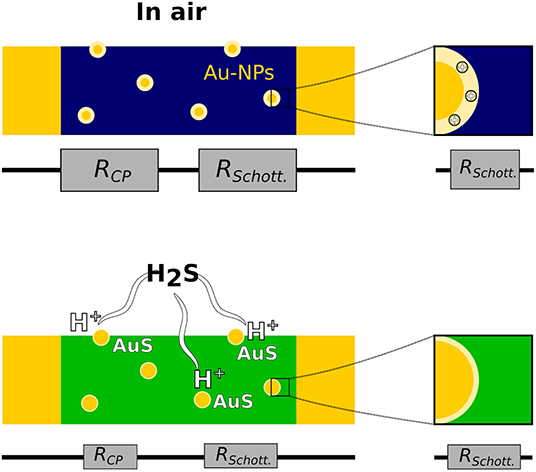
Figure 2. Mechanisms of H2S detection by CPs hybridized with metallic nanoparticles (Au-NPs). RCP and RSchott represent the resistance contribution of the polymer and depletion layer (in pale yellow) at the interface with the metal (Schottky junction), respectively. Those resistances tend to decrease in contact with H2S gas.
Given the sensing mechanism, the efficiency of the metallic fillers is highly dependent on their nature, geometry, and distribution. The role of sensitizing agents endorsed by metallic nanoparticles has been demonstrated on PANI nanowires decorated by Au-NPs (Shirsat et al., 2009). Electropolymerization followed by electrofunctionalization of the material leads to the formation of polymeric fibers with a diameter of 250–320 nm and well-dispersed NPs (70–120 nm). This system shows an extraordinarily high response at sub-ppb level (−20% at 0.1 ppb) when the unfunctionalized nanofibers have a limit of detection four orders of magnitude higher (>50 ppm). The high performance of the system is mainly attributed to its morphology (i.e., nanofibers decorated by nanoparticles) and the good dispersion of the metallic particles.
Moreover, the addition of metal can enhance the selectivity toward H2S. Compared to the pristine polymer, electropolymerized polycarbazole covered by a 1-nm layer of gold shows a response toward 40 ppm H2S doubled when its response toward 40 ppm NH3 is divided by a factor of 7.5 (Joshi et al., 2014). The slower response and recovery of the functionalized surface endorse the authors' assumption, i.e., this specific behavior is due to the enhanced interaction between H2S and the material.
Briefly, metal/CP composites have shown their ability to detect H2S from 0.1 ppb to 800 ppm. PANI nanofibers decorated with gold particles provide the lowest detection limit from the literature dedicated to organic chemiresistive sensors for H2S detection (Shirsat et al., 2009). The origin of this good performance can be attributed to three factors: (i) the morphology insures the accessibility to a high number of active sites, (ii) the presence of gold leads to the release of protons by reacting with H2S, and (iii) the formation of Schottky junction in the bulk of material leads to the amplification of the response toward H2S. In order to optimize the sensitive materials, the exact role of the metallic items in hybridized CPs needs to be clarified and, most importantly, the effects of environmental parameters such as humidity need to be considered. Indeed, those parameters have never been investigated on the hybridized metal/CPs when they are crucial for the end-use of gas sensors.
Metal Oxide and Sulfide Composites
Metal oxides (MOx) are the most commonly employed material in chemiresistive gas sensors in the academic and industrial field (Korotcenkov and Cho, 2017; Llobet et al., 2017; Joshi et al., 2018). The hybridization of such material with CPs often aims to overcome some of their drawbacks, e.g., their low selectivity and high operating temperature, and to optimize their sensing performances (Hangarter et al., 2013). For H2S detection, mechanical mixing and in situ polymerization have been used to prepare MOx/CPs hybrids based on PPy, PANI, or polythiophene and WO3, SnO2, or the metal sulfide CdS (Supplementary Table 3).
The presence of H2S can induce a decrease in the resistance of both CPs and n-type semiconductors. This fact tends to support the assumption that the conjoint effect of gas on the different moieties of the material is at the origin of the increased sensitivity of hybrids (Geng et al., 2006). However, it is not the lonely mechanism involved.
In composites based on organic and inorganic semiconductors, the inorganic moieties are often the main sensitizing agents when the polymer plays the role of amplifier (Hangarter et al., 2013). Indeed, the adsorption of reducing/oxidizing gas modulates easily the resistance of inorganic semiconductors by affecting the properties of the depletion layer at the interface gas/solid. In air, adsorbed oxygen traps electrons from n-type MOx (which are classically used in H2S sensing), resulting in a lower concentration of electron at the surface than in the bulk of the material (i.e., appearance of a depletion layer). Upon exposure to H2S, the gas reacts with adsorbed oxygen ions (), leading to the release of the trapped electrons and the decrease in the resistance, as shown in the following equations (Moseley et al., 1991; Mirzaei et al., 2018):
This mechanism of sensing often insures toward MOx a higher sensitivity than CPs. For example, polythiophene shows a response four times lower than WO3 and nine times lower than the composite loading WO3 and 10% of polythiophene toward 100 ppm H2S at 70°C (Bai et al., 2014).
As illustrated in Figure 3, the mechanism of amplification is based on the appearance of p–n junctions at the interface of p-type CPs and n-type MOx. The inclusion of CPs increases the width of the depletion layer of MOx (Su and Peng, 2014). As seen for noble metal/CP hybrids, the presence of heterojunctions produces a new potential barrier in material, which are highly sensitive toward gas absorption (Bärtsch and Niederberger, 2017; Walker et al., 2019). When H2S is adsorbed on the composite, more electrons migrate from the polymer to MOx, decreasing the potential barrier at the interface CPs/MOx. The induced resistance change is extreme and makes easier the detection of the gas.
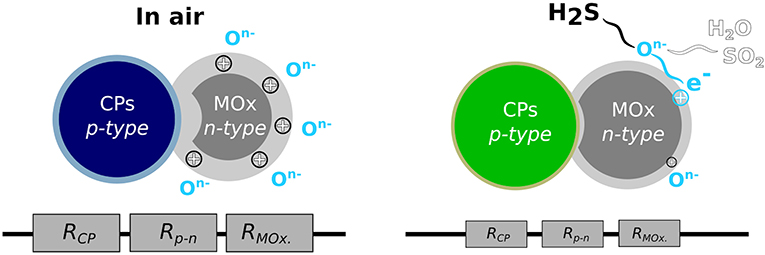
Figure 3. Mechanisms of H2S detection by CPs hybridized with metal oxide (MOx). RCP, RMOx, and Rp−n represent the resistance contribution of the polymer, the MOx, and the depletion layer at the interface between the two materials (p–n junction), respectively. The depletion layer is represented by lighter colors. The size of the resistance represents their tendency to decrease in contact with H2S.
Thereby, the presence of a heterojunction in the material leads to an increase in the sensitivity and decrease in the limit of detection. For example, WO3 NPs decorated with PPy by in situ photopolymerization show a response around −80% toward 1 ppm H2S when pure WO3 and PPy present a response of −10 and −25%, respectively. Noticeable changes in resistance (−8.9%) are still visible on the hybrid sensor at 100 ppb in contrast to the sensors based on pure material (Su and Peng, 2014). Moreover, hybridizing CPs with MOx can also shorten the response and recovery times. As illustration, in comparison to pure inorganic material, the oxidative polymerization of PPy around SnO2 nanograins results in a response time and a recovery time at 50 ppm divided by more than six and three, respectively (Shu et al., 2017). Additionally, among the enhancements allowed by hybridization is the decrease in the operating temperature. Indeed, the hybrid composites dedicated to H2S present a generally lower operating temperature than the pure MOx sensor does. As shown in the Supplementary Table 3, most of the systems work at room temperature when the others present an optimal response at 70 or 90°C.
However, the improvement in the performances of hybrids is not always easy to capture given the high sensitivity of this strategy to the quality of CPs, metal oxide, and interfaces as well as the ratio of CPs/MOx and the resultant morphology (Hangarter et al., 2013). As shown on polythiophene/WO3, the composite with 10% of polymer shows a response 2.3 times higher than that of pure WO3. However, above this content, the sensing performance decreases to reach a lower performance than pure WO3 at 30% (Bai et al., 2014). With a high amount of polymer, the organic layer is too thick to allow the gas to contact the inorganic sensitizing agent, affecting the sensing properties of the system. A compromise is necessary between the extent of the heterojunction (i.e., the extent of the interface CPs/MOx) and the accessibility to MOx.
Finally, a promising approach to optimizing the properties of hybrid CPs/MOx sensors is the inclusion of the doping agent in the MOx lattice in order to create new active sites. In the PPy/SnO2 composite, substituting Cu for Sn atoms by doping SnO2 with Cu2+ ions promotes the generation of oxygen vacancies acting as reaction sites (i.e., where adsorbed gas easily traps electrons from SnO2). Thereby, the response (defined as S = (Ig – Ia)/Ia, where Ig and Ia correspond, respectively to the steady-state current of the sensor in H2S and air) of the doped-hybrid material is about 4 at 50 ppb when the response of undoped PPy/SnO2 is not appreciable at 10 ppm (Shu et al., 2017). The synergetic effect from the doping providing an extra active site and the polymer creating a heterojunction gives rise to sensors having high sensitivity, fast response and recovery rate, satisfactory selectivity, and long-term stability over 1 month.
Concisely, MOx/CP composites have shown their ability to detect H2S from 0.05 to 1,000 ppm. The hybridization with MOx can enhance drastically the performance of the H2S sensor by improving the quality of the response (i.e., the sensitivity, limit of detection, response and recovery time) and the operating condition of the sensor by decreasing the temperature. Given the entanglement of the sensing mechanisms in the material (i.e., sensing from CP, MOx and p–n heterojunction), the synergetic effect between MOx and CPs can be found delicate and the parameters controlling their efficiency are numerous (e.g., synthesis, process of deposition, morphology). Further investigation is needed to understand better the impact of each sensing mechanism and their controlling parameter. Moreover, the impact of environmental conditions and long-term stability (>1 month) are too rarely investigated once they become crucial for sensor application.
Metal-Chloride Composites
The addition of metal chlorides to CPs has been done directly in solution or by alternate deposition (i.e., deposition of a polymeric layer covered by a metallic layer, etc.). In the literature dedicated to H2S sensing, only PANI has been hybridized with metallic chlorides (Supplementary Table 4). Commonly, CuCl2 is used for this purpose given its higher sensing performances (Virji et al., 2005).
Metal chlorides are used to overcome the fact that H2S is a weak acid, which cannot directly induce high changes in the conductivity of CPs. Indeed, hydrogen sulfide is known to react with metal salts (MCl2) in solution to form a metal sulfide (MS) and a strong acid (HCl) (Skoog and West, 1963), as illustrated in the following Equation:
n air, metal and chloride ions are strongly bound, making the reaction with H2S unfavorable. The association with PANI by coordination of metal ions facilitates the reaction with gas. Therefore, the presence of the two components is necessary for the detection of H2S gas. In contact with metal salts lightly coordinated to nitrogen atoms from the polymer, H2S releases a strong acid, which dopes PANI resulting in a drastic increase in polymer conductivity (Virji et al., 2005).
Given the mechanisms of action, the hybrids based on metal chloride present two specificities. Showing a variation of resistance of a few orders of magnitude at 10 ppm, they have the highest response from literature but their responses are quasi-systematically irreversible. For example, screen-printed PANI–CuCl2 from an aqueous solution shows a resistance divided by 105 toward 15 ppm H2S at room temperature but the signal presents no recovery during the ventilation of the sample even after 20 h (Sarfraz et al., 2013). The enhanced response is attributed to the doping effect from HCl released, associated with a favorable morphology, facilitating the diffusion of the analyte in the bulk of the material. Unlike the doping of PANI, the reaction of metal chloride with H2S is irreversible, resulting in the formation of stable copper sulfides (CuS and/or Cu2S), which are good conductors at room temperature (Silvester et al., 1991).
The unique hybrid material based on metal chloride showing recovery are surface-printed layer-by-layer alternating PANI NPs and CuCl2 deposited on silver electrodes (Crowley et al., 2010). The reversibility of the signal depends on the nature of the electrode. Indeed, the signal is irreversible on carbon electrodes and completely reversible on silver for several exposures at a high concentration (100 ppm). The presence of silver postpones the end of the stock of reactants able to form strong acid. Silver chlorides (AgCl) can be formed in the presence of copper chlorides (CuCl2), leading to the formation of copper (Cu) and/or copper(I) chloride (CuCl). Under exposition to H2S, both chlorides and metal can react, resulting in the release of proton, electron, and HCl according to the following equations:
The release of electrons is assumed to be at the origin of the reversibility of the signal. However, due to the finite reservoir of free copper, the sensor response results to be irreversible over time.
Shortly, CPs hybridized with metal chloride have shown their ability to detect H2S from 2.5 to 15 ppm. The use of metal chloride insures a high response toward H2S. However, due to the irreversible reaction at the origin of the enhanced response, those hybrid materials can be used only for short-time applications.
Graphitic Composites (CNT/Graphene)
Due to their good transducing properties, efficient adsorption of gas molecules, and high aspect ratio (Joshi et al., 2018), carbon nanotubes (CNT) and graphene are the most explored additives for the hybridization of CPs in gas sensing (Hangarter et al., 2013; Varghese et al., 2015). However, only few carbon-based composites are dedicated to H2S sensing (Supplementary Table 5). Graphitic hybrid CPs are exclusively composed of PANI decorated by single-wall nanotubes (SWNT), graphene oxide (GO), or reduced graphene oxide (rGO).
In the literature dedicated to H2S, carbon fillers have never been employed as sensitizing agent. Firstly, they are used to enhance analyte and/or charge transport. For example, in PSS-doped PANI hybridized with GO, H2S is not detected by pristine graphene sheets when the response of the composites is −20% toward 1 ppm of gas (Cho et al., 2014). Moreover, the conductivity is changing from 11.2 to 168.4 S/cm with the addition of 30 wt% of graphene.
Secondly, carbon fillers can be used as an amplifier in a ternary system based on the p–n junction by adding new p–n interfaces and thus increasing the effect of the depletion region on the signal. In the SnO2/RGO/PANI nanocomposite, the heterojunction is the main contributor to sensing enhancement (Zhang et al., 2019). The band gaps of SnO2, RGO, and PANI are 3.5, 4.39, and 4.49 eV, respectively, resulting in the formation of a p–n junction between the two p-type materials (i.e., RGO and PANI) and the metal oxide (SnO2).
Finally, carbon-based hybrids have shown their ability to detect H2S from 50 ppb to 30 000 ppm. However, the graphitic composites often result in low selectivity toward H2S with a higher response toward NH3, as shown for PANI hybridized with SWNT (Lim et al., 2010) or GO (Gaikwad et al., 2017).
Sensing Performances
The first characteristics of sensing performance are the amplitude and rate of the response. Figure 4 summarizes the relative response of the materials discussed previously as a function of the lowest concentration tested and the response time at different concentrations (the values and references are detailed in Supplementary Tables 1–5).
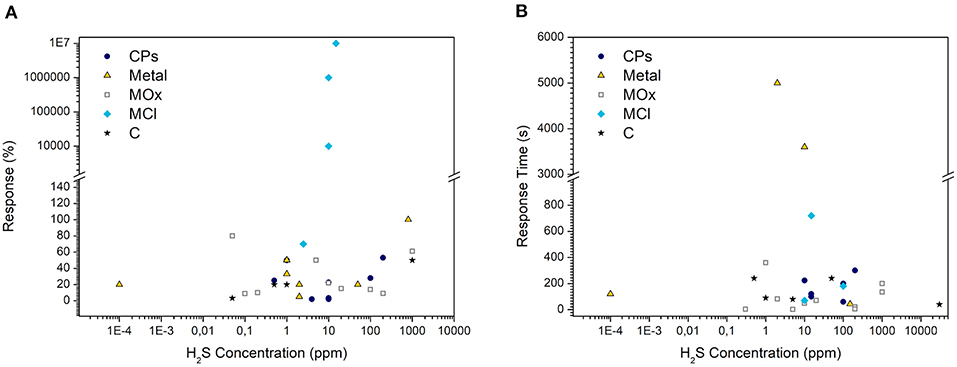
Figure 4. (A) Relative responses of different materials at the lowest H2S concentration tested. The relative response is defined as (Rg-R0)*100/R0, where Rg and R0 correspond to the resistance of the sensor in H2S and in the reference medium (air or nitrogen). It has been directly extracted or calculated from the papers presented in Supplementary Tables 1–4. (B) Response times of different materials directly extracted from the literature. Metal, MOx, MCl, and C refer to hybrid composites based on conductive polymers (CPs) mixed with noble metal particles and metal-oxide, metal-chloride, and carbon-based items, respectively.
Considering the relative response (Figure 4A), the hybrids filled with metal chloride present an extraordinarily high amplitude of response about several orders of magnitude. This performance can be explained by the sensing mechanism, resulting in the release of a strong acid in the presence of H2S jointly to the increase in filler conductivity. Tested between 2.5 and 15 ppm, those sensors show the highest relative response, but the contact with the target gas induces irreversible changes in the material giving rise to devices with a short lifetime.
In contrast, odor disturbance is felt below 1.5 ppm (Malone Rubright et al., 2017), and the guideline value likely to protect the public from H2S odor nuisance established by the World Health Organization is 7 μg/m3 (i.e., 4 ppb for an averaging time of 30 min) (World Health Organization, 2000); only few studies characterize the sensors at the sub-ppm level. The lack of characterization at lower concentrations is not specific to CP-based sensors. Indeed, a similar trend is observed on MOx sensors (Mirzaei et al., 2018). According to Figure 4A, PANI-nanofibers decorated with gold nanoparticles are the lonely CP-based material characterized below 50 ppb (Shirsat et al., 2009). Presenting a good response even at a lower concentration (−20% at 0.1 ppb), this nanostructured hybrid material highlights the high potential of CP-based sensors. Indeed, it largely outperforms chemiresistive sensors based on metal oxide nanostructures, whose best combination (i.e., highest response at the lowest concentration) is −33% at 10 ppb for CuO nanowires (Mirzaei et al., 2018).
Given the necessity of real-time measurement, the rapidity of the sensor is also an important parameter to consider. This parameter is often characterized by the response time and less systematically by the recovery time, which are the times to reach 90% of the resistance change upon injection of the target gas and reference gas (air or nitrogen), respectively. As shown in Figure 4B, the response time of pure CPs comprised the range 100–300 s. In general, the addition of MOx allows reducing the response time, to values lower than 120 s. The lowest response time (2.5 s at 5 ppm) is observed on nanostructured WO3 functionalized with polythiophene. Despite the increase in the response time with the concentration of H2S, it is still lower than 35 s even at 200 ppm (Bai et al., 2014). In addition, this material shows a fast recovery (<15 s). The hierarchical structure of the material can be one reason for this high rapidity. Here, the hybridization of CPs and the fabrication of an open hierarchical structure appear as functional approaches to enhancing the velocity of CP-based devices.
Beyond the sensitivity and velocity, selectivity needs to be considered in order to evaluate the performance of a device. Ammonia is the interfering species most frequently investigated on CP-based chemiresistive sensors dedicated to H2S sensing (Supplementary Tables 1–5). Commonly inducing an opposite change of resistance than H2S, ammonia can induce high response, which can disturb the ability of the sensor to quantify the target gas as seen on carbon-based hybrids and some of pure CPs. The hybridization with metal, MOx, or metal chloride seems to enhance the selectivity toward H2S. However, the exact benefit of the fillers on the selectivity is difficult to prove given the fact that the interfering studies are most of the time only conducted on the best composite and not on its different components. Further investigations are necessary to clarify the origin of increased selectivity in order to rationalize the research on sensitive material.
Then, the stability of the material under different environmental conditions is crucial to turning those CP-based sensors effective on the field. This pre-requisite encompasses two main capacities of the devices: (i) the preservation of its behavior under different humidities and temperatures and (ii) the conservation of its performance over time. Those two parameters undergo a lack of consideration in the literature. Indeed, <25% of the studies address the impact of humidity or time. When the increase in humidity can induce an increase or a decrease in response depending on the material, the mechanisms at the origin of this changing behavior are rarely explicated. Moreover, only short-term aging is considered. CP-based sensors seem stable for a few weeks. For example, WO3 nanoparticles decorated by PPy maintain 83% of its response toward 1 ppm of H2S after 54 days (Su and Peng, 2014), which corresponds to the longest aging test.
Conclusion And Perspectives
Finally, working at room temperature, easily tuned by changing monomers or dopants, easily processed, and integrated into low-cost and miniaturized devices, CPs present a large potential in H2S sensing applications. The common approach to enhancing their sensing performance while retaining the advantages from their polymeric nature is to hybridize them with inorganic fillers (e.g., noble metal particles, metal oxide or chloride items, and carbon-based nano-objects). The addition of those fillers allows decreasing the limit of detection and the response time, leading to composites competing with MOx sensors. However, more investigation is needed to be able to establish a clear link between the material properties (chemical nature and morphology) and its sensing performance. Moreover, none of the sensors developed have been employed in realistic situations. Indeed, the impact of interfering species (other reducing/oxidizing gas), environmental conditions (humidity and temperature), and time should be examined more systematically. While field measurement can be complicated to implement, experiments with continuous changes in H2S concentration, using gas mixture as reference and controlled humidity/temperature, can easily be done in laboratory in order to validate the applicative potential of the materials under investigation.
Author Contributions
CD made the illustrations and the main part of the bibliographic, analytic, and writing work. M-LB participated in the bibliographic research. J-LW and NR participated in the reflection and correction. All authors contributed to the article and approved the submitted version.
Funding
The study has been funded by Institut Mines-Télécom Lille Douai (IMT Lille Douai).
Conflict of Interest
The authors declare that the research was conducted in the absence of any commercial or financial relationships that could be construed as a potential conflict of interest.
Acknowledgments
The authors gratefully acknowledge Alexis Vlandas (CNRS, IEMN) and Sabine Crunaire (IMT Lille Douai) for their corrections and fruitful discussions and Patricia Krawczak (IMT Lille Douai) for the opportunity to participate in the article collection Women in Science: Materials.
Supplementary Material
The Supplementary Material for this article can be found online at: https://www.frontiersin.org/articles/10.3389/fmats.2020.00215/full#supplementary-material
References
Agbor, N. E., Petty, M. C., and Monkman, A. P. (1995). Polyaniline thin films for gas sensing. Sensors Actuators B. Chem. 28, 173–179. doi: 10.1016/0925-4005(95)01725-9
Anderson, T., and Roth, S. (1994). Conducting polymers: electrical transport and current applications. Braz. J. Phys. 24, 746–754.
Bai, H., and Shi, G. (2007). Gas sensors based on conducting polymers. Sensors 7, 267–307. doi: 10.3390/s7030267
Bai, S., Zhang, K., Sun, J., Zhang, D., Luo, R., Li, D., et al. (2014). Polythiophene-WO3 hybrid architectures for low-temperature H2S detection. Sensors Actuators B. Chem. 197, 142–148. doi: 10.1016/j.snb.2014.02.038
Bärtsch, M., and Niederberger, M. (2017). The role of interfaces in heterostructures. ChemPlusChem 82, 42–59. doi: 10.1002/cplu.201600519
Beauchamp, R. O., Bus, J. S., Popp, J. A., Boreiko, C. J., Andjelkovich, D. A., and Leber, P. (1984). A critical review of the literature on hydrogen sulfide toxicity. Crit. Rev. Toxicol. 13, 25–97. doi: 10.3109/10408448409029321
Chabukswar, V. V., Bhavsar, S. V., Horne, A. S., Handore, K., Gaikwad, V. B., and Mohite, K. C. (2013). Conducting poly(N-propylaniline) nanoparticles for hydrogen sulfide gas detection. Macromol. Symp. 327, 39–44. doi: 10.1002/masy.201350504
Cho, S., Lee, J. S., Jun, J., Kim, S. G., and Jang, J. (2014). Fabrication of water-dispersible and highly conductive PSS-doped PANI/graphene nanocomposites using a high-molecular weight PSS dopant and their application in H2S detection. Nanoscale 6, 15181–15195. doi: 10.1039/C4NR04413D
Crowley, K., Morrin, A., Shepherd, R. L., In Het Panhuis, M., Wallace, G. G., Smyth, M. R., et al. (2010). Fabrication of polyaniline-based gas sensors using piezoelectric inkjet and screen printing for the detection of hydrogen sulfide. IEEE Sens. J. 10, 1419–1426. doi: 10.1109/JSEN.2010.2044996
Dong, X., Zhang, X., Wu, X., Cui, H., and Chen, D. (2016). Investigation of gas-sensing property of acid-deposited polyaniline thin-film sensors for detecting H2S and SO2. Sensors 16:1889. doi: 10.3390/s16111889
Dongmin Kang, S., and Jeffrey Snyder, G. (2017). Charge-transport model for conducting polymers. Nat. Mater. 16, 252–257. doi: 10.1038/nmat4784
Fratoddi, I., Venditti, I., Cametti, C., and Russo, M. V. (2015). Chemiresistive polyaniline-based gas sensors: a mini review. Sensors Actuators B. Chem. 220, 534–548. doi: 10.1016/j.snb.2015.05.107
Gaikwad, G., Patil, P., Patil, D., and Naik, J. (2017). Synthesis and evaluation of gas sensing properties of PANI based graphene oxide nanocomposites. Mater. Sci. Eng. B Solid State Mater. Adv. Technol. 218, 14–22. doi: 10.1016/j.mseb.2017.01.008
Garg, R., Kumar, V., Kumar, D., and Chakarvarti, S. K. (2015). Polypyrrole microwires as toxic gas sensors for ammonia and hydrogen sulphide. J. Sensors Instrum. 3, 1–13. doi: 10.7726/jsi.2015.1001
Geng, L., Huang, X., Zhao, Y., Li, P., Wang, S., Zhang, S., et al. (2006). H2S sensitivity study of polypyrrole/WO3 materials. Solid. State. Electron. 50, 723–726. doi: 10.1016/j.sse.2006.04.024
Guo, Z., Chen, G., Zeng, G., Liu, L., and Zhang, C. (2015). Metal oxides and metal salt nanostructures for hydrogen sulfide sensing: mechanism and sensing performance. RSC Adv. 5, 54793–54805. doi: 10.1039/C5RA10394K
Habeeb, O. A., Kanthasamy, R., Ali, G. A. M., Sethupathi, S., and Yunus, R. B. M. (2018). Hydrogen sulfide emission sources, regulations, and removal techniques: a review. Rev. Chem. Eng. 34, 837–854. doi: 10.1515/revce-2017-0004
Hangarter, C. M., Chartuprayoon, N., Hernández, S. C., Choa, Y., and Myung, N. V. (2013). Hybridized conducting polymer chemiresistive nano-sensors. Nano Today 8, 39–55. doi: 10.1016/j.nantod.2012.12.005
Iqbal, S., and Ahmad, S. (2018). Recent development in hybrid conducting polymers: synthesis, applications and future prospects. J. Ind. Eng. Chem. 60, 53–84. doi: 10.1016/j.jiec.2017.09.038
Joshi, N., Hayasaka, T., Liu, Y., Liu, H., Oliveira, O. N., and Lin, L. (2018). A review on chemiresistive room temperature gas sensors based on metal oxide nanostructures, graphene and 2D transition metal dichalcogenides. Microchim. Acta 185:213. doi: 10.1007/s00604-018-2750-5
Joshi, N., Saxena, V., Singh, A., Koiry, S. P., Debnath, A. K., Chehimi, M. M., et al. (2014). Flexible H2S sensor based on gold modified polycarbazole films. Sensors Actuators B. Chem. 200, 227–234. doi: 10.1016/j.snb.2014.04.041
Kaushik, A., Kumar, R., Arya, S. K., Nair, M., Malhotra, B. D., and Bhansali, S. (2015). Organic-inorganic hybrid nanocomposite-based gas sensors for environmental monitoring. Chem. Rev. 115, 4571–4606. doi: 10.1021/cr400659h
Kivelson, S., and Heeger, A. J. (1988). Intrinsic conductivity of conducting polymers. Synth. Met. 22, 371–384. doi: 10.1016/0379-6779(88)90108-7
Korotcenkov, G., and Cho, B. K. (2017). Metal oxide composites in conductometric gas sensors: achievements and challenges. Sensors Actuators B. Chem. 244, 182–210. doi: 10.1016/j.snb.2016.12.117
Kriván, E., Visy, C., Dobay, R., Harsányi, G., and Berkesi, O. (2000). Irregular response of the polypyrrole films to H2S. Electroanalysis 12, 1195–1200. doi: 10.1002/1521-4109(200010)12:15andlt;1195::AID-ELAN1195andgt;3.0.CO;2-1
Krukiewicz, K., and Katunin, A. (2016). The effect of reaction medium on the conductivity and morphology of polyaniline doped with camphorsulfonic acid. Synth. Met. 214, 45–49. doi: 10.1016/j.synthmet.2016.01.017
Le, T.-H., Kim, Y., and Yoon, H. (2017). Electrical and electrochemical properties of conducting polymers. Polymers. 9:150. doi: 10.3390/polym9040150
Li, W., and Wan, M. (1999). Stability of polyaniline synthesized by a doping-dedoping-redoping method. J. Appl. Polym. Sci. 71, 615–621. doi: 10.1002/(SICI)1097-4628(19990124)71:4andlt;615::AID-APP13andgt;3.0.CO;2-O
Lim, J. H., Phiboolsirichit, N., Mubeen, S., Deshusses, M. A., Mulchandani, A., and Myung, N. V. (2010). Electrical and gas sensing properties of polyaniline functionalized single-walled carbon nanotubes. Nanotechnology 21:75502. doi: 10.1088/0957-4484/21/7/075502
Liu, C., Hayashi, K., and Toko, K. (2012). Au nanoparticles decorated polyaniline nanofiber sensor for detecting volatile sulfur compounds in expired breath. Sensors Actuators B. Chem. 161, 504–509. doi: 10.1016/j.snb.2011.10.068
Llobet, E., Brunet, J., Pauly, A., Ndiaye, A., and Varenne, C. (2017). Nanomaterials for the selective detection of hydrogen sulfide in air. Sensors 17, 1–19. doi: 10.3390/s17020391
Malone Rubright, S. L., Pearce, L. L., and Peterson, J. (2017). Environmental toxicology of hydrogen sulfide. Nitric Oxide. 71, 1–13. doi: 10.1016/j.niox.2017.09.011
Mekki, A., Joshi, N., Singh, A., Salmi, Z., Jha, P., Decorse, P., et al. (2014). H2S sensing using in situ photo-polymerized polyaniline-silver nanocomposite films on flexible substrates. Org. Electron. 15, 71–81. doi: 10.1016/j.orgel.2013.10.012
Mirzaei, A., Kim, S. S., and Kim, H. W. (2018). Resistance-based H2S gas sensors using metal oxide nanostructures: a review of recent advances. J. Hazard. Mater. 357, 314–331. doi: 10.1016/j.jhazmat.2018.06.015
Moseley, P. T., Norris, J. O. W., and Williams, D. E. (1991). Technique and Mechanism in Gas Sensing, ed A. Hilger. Bristol: Taylor & Francis.
National Research Council (US) Committee on Acute Exposure Guideline Levels (2010). “4 hydrogen sulfide acute exposure guideline levels,” in Acute Exposure Guideline Levels for Selected Airborne Chemicals (Washington, DC: National Academies Press), 173–218.
Nylabder, C., Armgrath, M., and Lundstrom, I. (1983). “An ammonia detector based on a conducting polymer,” in International Meeting on Chemical Sensors (Fukuoka), 203–207.
Occupational Safety Health Administration USA. (2020). OSHA Standards: Hydrogen Sulfide Exposure. OSHA. Available online at: https://www.osha.gov/SLTC/hydrogensulfide/hazards.html (accessed Sepetember 17, 2020).
Palaniappan, S., and Saravanan, C. (2010). Polyaniline–maleicacid–dodecylhydrogensulfate salt as sensor material for toxic gases. J. Appl. Polym. Sci. 118, 518–524. doi: 10.1002/app.32347
Pandey, S. K., Kim, K. H., and Tang, K. T. (2012). A review of sensor-based methods for monitoring hydrogen sulfide. TrAC Trends Anal. Chem. 32, 87–99. doi: 10.1016/j.trac.2011.08.008
Park, S. J., Park, C. S., and Yoon, H. (2017). Chemo-electrical gas sensors based on conducting polymer hybrids. Polymers. 9:155. doi: 10.3390/polym9050155
Patil, Y. S., Salunkhe, P. H., Navale, Y. H., Patil, V. B., Ubale, V. P., and Ghanwat, A. A. (2019). Tetraphenylthiophene–thiazole-based π-conjugated polyazomethines: synthesis, characterization and gas sensing application. Polym. Bull. 77, 2205–2226. doi: 10.1007/s00289-019-02856-2
Phillips, P., and Wu, H. L. (1991). Localization and its absence: a new metallic state for conducting polymers. Science. 252, 1805–1812. doi: 10.1126/science.252.5014.1805
Ramgir, N. S., Sharma, P. K., Datta, N., Kaur, M., Debnath, A. K., Aswal, D. K., et al. (2013). Room temperature H2S sensor based on Au modified ZnO nanowires. Sensors Actuators B. Chem. 186, 718–726. doi: 10.1016/j.snb.2013.06.070
Sarfraz, J., Ihalainen, P., Määttänen, A., Peltonen, J., and Lindén, M. (2013). Printed hydrogen sulfide gas sensor on paper substrate based on polyaniline composite. Thin Solid Films 534, 621–628. doi: 10.1016/j.tsf.2013.02.055
Serban, B., Cobianu, C., and Brezeanu, M. (2014). Hydrogen sulphide sensing review. Ann. Acad. Romanian Sci. Ser. Sci. Technol. Inf. 7, 55–67.
Shirsat, M. D., Bangar, M. A., Deshusses, M. A., Myung, N. V., and Mulchandani, A. (2009). Polyaniline nanowires-gold nanoparticles hybrid network based chemiresistive hydrogen sulfide sensor. Appl. Phys. Lett. 94, 2012–2015. doi: 10.1063/1.3070237
Shu, J., Qiu, Z., Lv, S., Zhang, K., and Tang, D. (2017). Cu2+-doped SnO2 nanograin/polypyrrole nanospheres with synergic enhanced properties for ultrasensitive room-temperature H2S gas sensing. Anal. Chem. 89, 11135–11142. doi: 10.1021/acs.analchem.7b03491
Silvester, E. J., Grieser, F., Sexton, B. A., and Healy, T. W. (1991). Spectroscopic studies on copper sulfide sols. Langmuir 7, 2917–2922. doi: 10.1021/la00060a009
Skoog, D. A., and West, D. M. (Eds.) (1963). Fundamentals of Analytical Chemistry, 3rd Edn. New York, NY: Holt, Rinehart, and Winston.
Skotheim, T. A., and Reynolds, J. R. (Eds.). (2007). Handbook of Conducting Polymers - Conjugated Polymers Processing and Applications, 3rd Edn. Boca Raton, FL: CRC Press Taylor and Francis Group.
Su, P. G., and Peng, Y. T. (2014). Fabrication of a room-temperature H2S gas sensor based on PPy/WO3 nanocomposite films by in-situ photopolymerization. Sensors Actuators B. Chem. 193, 637–643. doi: 10.1016/j.snb.2013.12.027
Tranchida, P. Q., Franchina, F. A., Dugo, P., and Mondello, L. (2016). Comprehensive two-dimensional gas chromatography- mass spectrometry: recent evolution and current trends. Mass Spectrom. Rev. 35, 524–534. doi: 10.1002/mas.21443
Varghese, S. S., Lonkar, S., Singh, K. K., Swaminathan, S., and Abdala, A. (2015). Recent advances in graphene based gas sensors. Sensors Actuators B. Chem. 218, 160–183. doi: 10.1016/j.snb.2015.04.062
Virji, S., Fowler, J. D., Baker, C. O., Huang, J., Kaner, R. B., and Weiller, B. H. (2005). Polyaniline nanofiber composites with metal salts: chemical sensors for hydrogen sulfide. Small 1, 624–627. doi: 10.1002/smll.200400155
Walker, J. M., Akbar, S. A., and Morris, P. A. (2019). Synergistic effects in gas sensing semiconducting oxide nano-heterostructures: a review. Sensors Actuators B. Chem. 286, 624–640. doi: 10.1016/j.snb.2019.01.049
Wong, Y. C. Y. H., Ang, B. C., Haseeb, A. S. M. A., Baharuddin, A. A., and Wong, Y. C. Y. H. (2020). Review—conducting polymers as chemiresistive gas sensing materials: a review. J. Electrochem. Soc. 167:037503. doi: 10.1149/2.0032003JES
Zhang, D., Wu, Z., and Zong, X. (2019). Flexible and highly sensitive H2S gas sensor based on in-situ polymerized SnO2/rGO/PANI ternary nanocomposite with application in halitosis diagnosis. Sensors Actuators B. Chem. 289, 32–41. doi: 10.1016/j.snb.2019.03.055
Keywords: hybrid polymer, conductive polymer, chemiresistive sensor, hydrogen sulfide detection, H2S sensors, gas sensing
Citation: Duc C, Boukhenane M-L, Wojkiewicz J-L and Redon N (2020) Hydrogen Sulfide Detection by Sensors Based on Conductive Polymers: A Review. Front. Mater. 7:215. doi: 10.3389/fmats.2020.00215
Received: 27 April 2020; Accepted: 12 June 2020;
Published: 30 September 2020.
Edited by:
Emilia Morallon, University of Alicante, SpainReviewed by:
Peter Kruse, McMaster University, CanadaHaibao Lu, Harbin Institute of Technology, China
Copyright © 2020 Duc, Boukhenane, Wojkiewicz and Redon. This is an open-access article distributed under the terms of the Creative Commons Attribution License (CC BY). The use, distribution or reproduction in other forums is permitted, provided the original author(s) and the copyright owner(s) are credited and that the original publication in this journal is cited, in accordance with accepted academic practice. No use, distribution or reproduction is permitted which does not comply with these terms.
*Correspondence: Caroline Duc, Y2Fyb2xpbmUuZHVjQGltdC1saWxsZS1kb3VhaS5mcg==