- 1Department of Bioengineering, School of Engineering, The University of Tokyo, Tokyo, Japan
- 2Fuji Systems Corporation, Tokyo, Japan
- 3Department of Biomedical Engineering, Graduate School of Medicine, The University of Tokyo, Tokyo, Japan
- 4Department of Thoracic Surgery, Graduate School of Medicine, The University of Tokyo, Tokyo, Japan
Surface modification of hemocompatible copolymers on silicone elastomers (SEs) is crucial for the long-term use of medical devices. Both physical adsorption and chemical conjugation are important for modification of SE. Oxygen plasma treatment is widely used to produce silanol groups on SE for silane coupling. However, the plasma reaction is difficult to apply to the surface modification of three-dimensional complex devices. This study demonstrated an appropriate and efficient method with alkaline solution for producing silanol groups on SE for modifying phosphorylcholine-based copolymer with organosilane (cross-MPC copolymer). A 2.5 wt% aqueous solution of potassium hydroxide (KOH) was effective in producing silanol groups and for coating the cross-MPC copolymer. Additionally, we successfully modified the cross-MPC copolymer on the inner surface of SE tubes after pretreatment with the 2.5 wt% KOH aqueous solution, and the copolymer film was coated homogeneously. The cross-MPC copolymer film on SE was stable for one month under fluidic condition with a shear stress of 3.2 Pa. The hollow fiber membrane with the polymer coating inhibited blood coagulation after one week implantation with extracorporeal circulation device using a goat. Therefore, pretreatment of SE using an alkaline solution is an appropriate method for producing silanol groups for coating the cross-MPC copolymer by silane-coupling reaction.
Introduction
Artificial organs that are used in contact with blood should be hemocompatible. Specifically, artificial lungs (ALs) with hollow fibers, which have gas exchange functions for removing carbon dioxide and oxygenating blood, are used for treating acute respiratory failure or as a bridge for transplantation in the future (Federspiel et al., 2004; Iwahashi et al., 2004; Betit, 2018). Materials for hollow fibers in membrane-type oxygenators are classified into microporous materials such as polypropylene (PP) (Suma et al., 1981) and polymethyl pentene (PMP) (Huang et al., 2016) as well as homogeneous materials such as silicone elastomers (SEs), which have excellent oxygen transparency (Motomura et al., 2003). The prevention of blood coagulation on the surface of hollow fiber materials is an important strategy to improve their hemocompatibility. There are many reports on the modified surfaces of PP and PMP for clinical oxygenators (Maul et al., 2016; Ontaneda and Annich, 2018). Biological molecules such as polypeptides (Zimmermann et al., 2007; Reser et al., 2012), heparin (Larm et al., 1983; Wendel and Ziemer, 1999; Zimmermann et al., 2007; Reser et al., 2012), and albumin (Mulvihill et al., 1990) have been used as surface modification materials. Given that synthetic polymers are more stable than biological molecule coatings, surface modifications using synthetic polymers such as polyethylene oxide (Eynden et al., 2008), poly(2-methoxyethyl acrylate) (Suhara et al., 2001), and phosphorylcholine(PC)-based copolymers (De Somer et al., 2000; Reser et al., 2012) have been applied to commercial products (Maul et al., 2016).
Currently, the usage time of ALs with membrane-type oxygenators is limited to several hours in clinical use due to the poor durability; however, long-term durability is required to apply as a bridge for the transplantation of lungs (Duy Nguyen et al., 2021). Furthermore, there has been an increasing demand for the continuous use of oxygenators because of the Covid-19 pandemic (Sanford et al., 2020; Raasveld et al., 2021), recently.
SE could be a suitable material for long-term use of oxygenators in ALs because of the rejection of plasma leakage caused by homogeneous materials; however, durable surface modification of hemocompatible materials for SE is difficult to obtain. This is mainly because of the hydrophobic recovery of SE (Bausch et al., 1998). We developed a surface modification method using a PC-based copolymer with organosilane for SE (Nagahashi et al., 2015). The chemical structure of PC is similar to that of a hydrophilic head group of phospholipids in the outer cell membrane (Zwaal and Hemker, 1982), and it has non-thrombogenic properties (Pieri et al., 2013). This PC-based copolymer composed of 2-methacryloyloxyethyl phosphorylcholine (MPC), 3-(methacryloyloxy) propyl-tris(trimethylsilyloxy)silane (MPTSSi), and 3-methacryloxypropyl trimethoxysilane (MPTMSi), denoted as cross-MPC copolymer, exhibited excellent coating stability on SE. The methoxy silane moiety of the MPTMSi unit in the copolymer successfully reacted with the silanol group produced on the silicone surface by oxygen plasma. In addition, the MPTSSi unit, which had good affinity with silicone elastomer, helped the polymer film formation by the hydrophobic interaction with silicone elastomer surface (Seo et al., 2011; Nii et al., 2013). The coated cross-MPC copolymer on SE inhibited the adsorption of plasma proteins for three months.
Potassium hydroxide (KOH) solution is widely used as an anisotropic etching reagent for single-crystal silicon (Yun, 2000), etching of silica glass (Kouassi et al., 2010; Pfiffer et al., 2017), and production of a silanol group on the surface (Bruin et al., 1989). It was reported that SE could be depolymerized by a KOH solution and by cleaving the Si–O bond in the main chain (Brook et al., 2012). Moreover, KOH solution was also used to produce hydroxyl groups on the polymer surfaces, such as silicone-based materials (Zhang et al., 2018), and poly(vinylidene fluoride) (Al- Gharabli et al., 2017). In this study, we have applied the liquid-phase process with an alkaline solution for the surface functionalization of silicone elastomer, and evaluated the functions such as hemocompatibility and stability of coated film of the phosphorylcholine-based copolymer containing organosilane. This liquid-phase process has various advantages such as mass production and low cost compared with vacuum-phase processes, such as plasma treatment. Moreover, the durability of the cross-MPC copolymer film on SE was evaluated in vitro under dynamic flow condition for one month. The hemocompatibility of the cross-MPC copolymer film-coated hollow fiber membrane as a model of medical devices was tested using a goat blood circuit for a one-week in vivo experiment.
Materials and Methods
Materials
The cross-MPC copolymer was provided by NOF Corporation, Japan, and it was synthesized via free radical copolymerization of MPC, MPTSSi, and MPTMSi. The composition ratio of MPC, MPTSSi, and MPTMSi in the cross-MPC copolymer was 54, 27, and 19 mol%, respectively (Nagahashi et al., 2015). This ratio in the cross-MPC copolymer had been optimized to coat polymer film well with good inhibition for protein adsorption, and stability on silicone. Sheets (1.0 × 1.0 × 0.1 cm) and tubes (outer diameter = 0.9 cm; inner diameter = 0.6 cm, length = 10.0 cm) made of SEs were fabricated by Fuji Systems Co. (Tokyo, Japan). The membrane (1.0 × 1.0 cm) was fabricated from hollow fibers with an outer diameter of 0.4 mm, by holding hollow fibers at a constant spacing of 0.4 mm using a string.
Rhodamine 6G (R6G), 3-aminopropyltrimethoxysilane (APTES), KOH, sodium hydroxide (NaOH), and sodium dodecyl sulfate (SDS) were purchased from FUJIFILM Wako Pure Chemical Corporation Ltd. (Osaka, Japan). Methanol, ethanol, acetic acid, and phosphate-buffered saline (PBS) were purchased from Kanto Chemical Co., Inc. (Tokyo, Japan). Human serum albumin (HSA), human plasma fibrinogen (HPF), fluorescein isothiocyanate-labeled BSA (FITC-BSA), and glutaraldehyde (GA) were purchased from Sigma–Aldrich (St. Louis, MO, United States). The µ-BCA protein assay kit was purchased from Thermo Fisher Scientific Inc. (Waltham, MA, United States). The blood collection tube with 0.2 ml 3.2% citric acid for preparing platelet-rich plasma was purchased from Nipro Corporation (Osaka, Japan).
Alkaline Pretreatment and Oxygen Plasma Pretreatment on SE
The coating process of the crosslinking-type MPC-copolymer via pretreatment of its chemical structure are shown in Figure 1. The SE substrates were ultrasonically washed in ethanol for 15 min, followed by drying through air blowing. Subsequently, the substrates were immersed in an aqueous solution of NaOH or KOH for 1 h with gently shaking on shaker under different conditions, such as the type, concentration, and temperature (Table 1). For oxygen plasma treatment, substrates were treated under the condition of 20 W at 600 mTorr for 2 min (PDC-001, Harrick Plasma, NY, United States). Thereafter, the substrates were washed sufficiently with pure water.
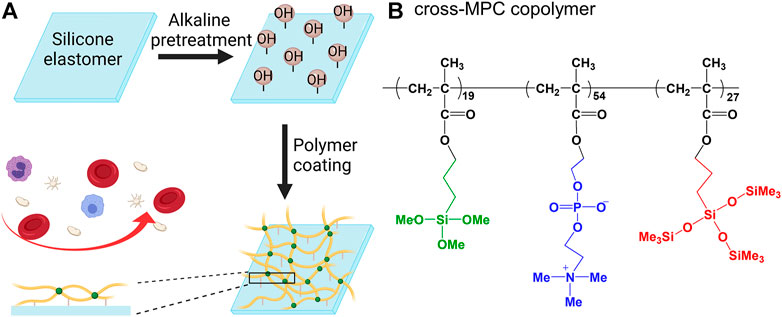
FIGURE 1. Schematic coating procedure for the cross-MPC copolymer film on silicone elastomer (SE) surface (A); Chemical structure of the cross-MPC-copolymer (B).
Modification of the Cross-MPC Copolymer by Dip Coating
A methanol solution containing 0.1 wt% polymer was mixed with an aqueous solution of 0.1 M acetic acid (acetic acid/polymer solution: 10/90, v/v) to prepare a coating solution. The sheets and hollow fibers were coated with the cross-MPC copolymer film by dipping in the coating solution for 2 h. To coat the inner surface of the tube with the cross-MPC copolymer, the tubes were filled with the polymer and were sealed with forceps. Thereafter, the sheets, hollow fibers, and tubes were dried in a vacuum chamber for 1 h, and then heated at 70°C for 3 h to dehydrate.
Evaluating the Formation of the Cross-MPC Copolymer Film on SE
To evaluate the uniformity and thickness of the cross-MPC copolymer film via fluorescence microscopy, R6G was stained on the polymer film (Wang et al., 2005). First, the cross-MPC copolymer-coated SE sheets were immersed in water for 1 h, and then they were soaked in an aqueous solution of 0.01 wt% R6G at room temperature for 30 s. Finally, they were immersed in water for 30 min to wash the extra R6G. The sheets were observed via fluorescence microscopy (Axioscope 2 Plus, Carl Zeiss AG, Oberkochen, Germany, 10X objective lens, exposure time: 1/30 s). The fluorescence images were captured using a charge-coupled device (CCD) camera (VB-7010, Keyence Co., Osaka, Japan), and fluorescence intensity was analyzed using an imaging software (ImageJ, Wayne Rasband). The surface morphology of the cross-MPC copolymer-coated sheets was observed via scanning electron microscopy (SEM) at an acceleration voltage of 5 kV (JSM-7000Fm, JEOL Ltd. Japan). The cross-MPC copolymer film was coated with osmium (Neoc-ST, MEIWAFOSIS Co. Japan) before the SEM observation.
Surface Characterization via X-Ray Photoelectron Spectroscopy
The elemental composition for evaluating the film stability and silanol groups was characterized via XPS (JPS-9010MC, JEOL, Tokyo, Japan). Elemental analysis of poly-SE surfaces was conducted via XPS equipped with a 10 kV magnesium Kα radiation source at an electron take-off angle of 90° from the surface.
Evaluation of Protein Adsorption on the Cross-MPC Copolymer-Coated SE
The amount of adsorbed protein on the cross-MPC copolymer-coated SE surfaces was evaluated via the analysis of relative fluorescence intensity (RFI) of the adsorbed FITC-BSA and quantification analysis of the adsorbed BSA using the micro-BCA assay kit. The SE sheets (1.0 × 1.0 × 0.1 cm) were incubated in 4.5 mg/ml BSA solution (FITC-BSA: BSA = 1 : 9) in PBS at 37°C for 1 h. Thereafter, the sheets were washed with PBS, and the fluorescence intensity was measured using a fluorescence microscope (Axioscope 2 Plus, Carl Zeiss AG, Oberkochen, Germany, 10X objective lens, exposure time: 1/2 s) and analyzed using ImageJ. RFI was calculated according to Eq. 1, where FIpoly-SE is the fluorescence intensity of the cross-MPC copolymer-coated SE and FISE is the fluorescence intensity of SE without polymer coating.
To quantify the adsorbed HSA and HPF, the SE sheets (1.0 × 4.0 × 0.1 cm) were incubated in 0.3 mg/ml HSA and 0.3 mg/ml HPF at 37°C for 1 h. The HSA and HPF adsorbed sheets were washed with PBS under magnetic stirring to remove the unabsorbed protein. Then the 3 pieces of protein adsorbed sheets were immersed in 6 ml of PBS containing 1 wt% SDS. Thereafter, ultrasonic waves were applied at room temperature for 5 min to detach the adsorbed HSA and HPF. The concentration of HSA and HPF in the SDS solution was determined using the µ-BCA protein assay.
Evaluating the Stability of the Cross-MPC Copolymer Film Under Fluidic Condition
The circulation system for evaluating the stability of the cross-MPC copolymer film under fluidic conditions is illustrated in Figure 2. Figure 2A shows the details of the test module used for the SE sheets. The wall shear stress (WSS) on the sheet was calculated using computational fluid dynamics (CFD) analysis software (ANSYS CFX, Ansys Inc., United States). First, a 3D model of the fluid-contacting surface was established using a computer-aided design (CAD) software (Creo 5.0, Parametric Technology Corporation, United States), as shown in Supplementary Figure S1. Prior to running the CFD analysis, the 3D model was segmented into tetrahedrons. The grid/mesh was fabricated using 1.3 × 107 elements (surface of tetrahedral structure) and 2.3 × 106 nodes (grid points of the element corner). As the parameters of the circulating fluid, the density and dynamic viscosity of PBS were set to 1723 kg/m3 and 0.8882 mPa s, respectively. The pressure of the outlet was 0 mmHg and the flow rate of the inlet was set to 8 L/min. The steady state flow was assumed with no-slip condition of the surface and non-gravity effects, and it was set as laminar flow because the Reynolds number was 3659 lower than 4000.
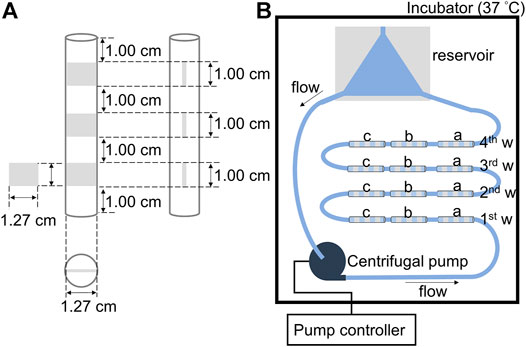
FIGURE 2. Schematic circulating system for evaluating the stability of the polymer film: (A) The tubing with three pieces of silicone elastomer (SE) sheets of 1.27 × 1.00 cm located inside at a distance of 1.00 cm. Cross-sectional view of the tubing. (B) Illustration of the liquid flow circulation circuit for evaluating the stability of the cross-MPC copolymer film on different pretreated SE sheets, a) oxygen plasma treated-silicone elastomer (OPT-SE), b) 2.5 wt% KOH treated silicone elastomer (2.5K-SE), and c) without pretreated silicone elastomer (SE).
The SE sheets (1.27 × 1.0 × 0.1 cm) pretreated with 2.5 wt% KOH (2.5K-), oxygen plasma treatment (OPT-), as well as those without pretreatment were used, and were coated with cross-MPC copolymer. The SE sheets were fixed into a Tygon® R3603 laboratory tubing (inner diameter = 1/2 in.; outer diameter = 5/8 in.; length = 9.0 cm). Thereafter, 12 pieces of the tubing with the SE sheets were connected in series, and a reservoir made of polyvinyl chloride and centrifugal pump (CAPIOX® SP, Terumo, Japan) were joined to form a liquid flow circulation circuit. The reservoir was used to remove air in tubes and centrifugal pumps (Brook et al., 2012). The liquid circulation circuit was filled with PBS and placed in an incubator at 37°C. The flow rate was controlled at 8 L/min (2500 rpm) using a centrifugal pump. PBS was allowed to flow for 7, 14, 21, and 28 days, then the SE sheets were rinsed with water and vacuum dried in a desiccator overnight. The atomic ratio of N/C (N in the MPC unit/C in the polymer film and SE) of the surface of the sheets was evaluated via XPS.
Evaluating the Silanol Group on SE
APTES was used for the reaction of the silanol group and the reaction between the cross-MPC-copolymer and the SE pretreated with alkaline was investigated. Additionally, the OPT-SE sheets were modified with APTES as a control. The pretreated SE sheets were dipped into a 0.5% APTES solution (in ethanol) for 1 h at room temperature. Thereafter, the APTES-modified sheets were rinsed with ethanol and dried in vacuum overnight in a desiccator. The spectra of C 1s, N 1s, O 1s, and Si 2p states were analyzed via XPS, and the atomic ratio of N/Si was calculated from the elemental composition of the SE after APTES modification.
Evaluation of Platelet Adsorption on the Cross-MPC Copolymer Film Coated on SE
Platelet-rich plasma (PRP) was freshly prepared from the whole blood of a goat (Japan Saanen goat, female, adult). The whole blood (1.8 ml) was collected using a syringe and injected into a blood collection tube. Thereafter, it was centrifuged at 184.5 × g (1000 rpm) for 30 min in a refrigerated compact centrifuge (himac-CF7D2, Hitachi Ltd. Japan) at 4°C. The mixture of plasma supernatant containing platelets and a small amount of red blood cells was transferred into another sterile tube and centrifuged at 1660.2 × g (3000 rpm) for 5 min at 4°C. The supernatant was used as PRP. The PRP concentration was 1.1×108 ± 1.2×107 cell/mL.
SE were immersed in PBS overnight. Thereafter, SE, and the cross-MPC copolymer-coated SE (poly-2.5K-SE) sheets were incubated in PRP at 37°C for 1 h. The incubated poly-2.5K-SE, and SE sheets were rinsed with PBS to remove unadhered platelets. The PRP incubation for stability evaluation was same as polymer coated SE. The adhered platelets were fixed with 2.5 wt% GA in PBS for 30 min at room temperature. All the sheets were immersed in 50, 60, 70, 80, 90, 95% ethanol/water solution, and 100% ethanol for 30 min in order. Thereafter, the sheets were dried in a vacuum overnight and stored in a desiccator. The platelets that adhered on the SEs were observed via SEM at an acceleration voltage of 5 kV (JSM-7000Fm, JEOL Ltd. Japan) after coating with osmium using a plasma coater.
Evaluation of Hemocompatibility via In-Vivo Experiment Using a Goat
The long-term blood compatibility of the hollow fibers made of SE in contact with blood was evaluated via an in-vivo experiment. Figure 3 illustrates the blood circulation system for the in vivo experiment using a goat (healthy Japanese Saanen goat, wild type, female, 44.5 kg). The blood tests were analyzed before surgery and the results are summarized in Supplementary Table S1. The in vivo experiment was performed according to the guidelines of the Animal Experiment Committee at the Graduate School of Medicine, the University of Tokyo. We fabricated a chamber that was pasted on the hollow fiber membrane (HFM) with a size of 1.0 × 1.0 cm. The membrane was pre-treated at 2.5 K (Table 1) and coated with the cross-MPC copolymer by dipping it in a of 0.1 wt% polymer solution for 1 h. Thereafter, it was dried in vacuum for 1 h and heated for 3 h. Subsequently, the cross-MPC copolymer-coated membrane (poly-HFM) and that not coated (HFM) were fixed on a rectangular acrylic plate. Acrylic plates with 16 pieces of poly-HFM and HFM were then fabricated as chambers, as shown in Figure 3A.
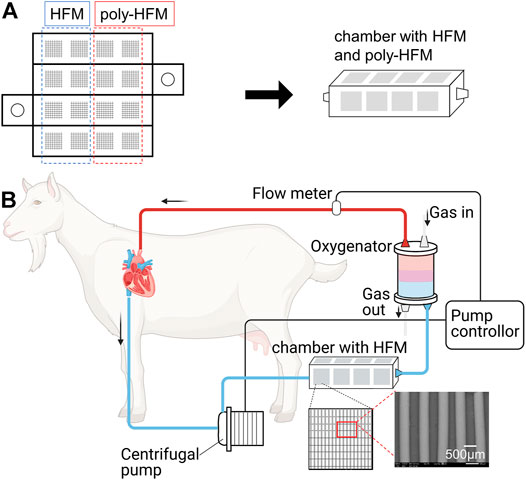
FIGURE 3. (A) Development of a chamber of acrylic plates pasted with membranes made of hollow fibers (HFM) of 1.0 × 1.0 cm. Eight pieces of HFM were coated with the cross-MPC copolymer (poly-HFM). (B) Schematic illustration of in-vivo experiment for evaluating the blood compatibility. The chamber was connected to the ECMO system in series. The centrifugal pump in ECMO system was controlled from 1.0 to 2.0 L/min.
Anesthesia was induced using isoflurane. The pulmonary artery was connected to sutured cannulas of inflow and outflow to the left atrial appendage. An extracorporeal membrane oxygenation (ECMO) system (EMERSAVE, Terumo Corporation) was attached to the sutured cannula for extracorporeal circulation. The chamber was attached by cutting the tube in the circulation system between the centrifugal pump and AL. The blood flow was measured using a flow meter and controlled using a pump controller between 1.0 and 2.0 L/min. The activated clotting time (ACT) in the blood was adjusted to less than 250 using heparin. After 1 week of circulation, the chamber was removed from the ECMO system. After washing the chamber with PBS, the poly-HFMs and HFMs were fixed with 2.5% GA and observed via SEM.
Results and Discussion
Alkaline Pretreatment Conditions for the Cross-MPC Copolymer Coating
OPT is a method for the pretreatment of the surface modification of SE to produce silanol groups. However, the travel length of the active plasma species produced under vacuum is limited by pressure and flow rate. In addition, the active species are eliminated by collision with the surface of the materials. Therefore, OPT is not appropriate for the inner wall surfaces of long tubes and textile hollow fibers (Pfiffer et al., 2017). Therefore, we focused on a pretreatment method using an alkaline solution without vacuuming and complex operation, which is suitable for the three-dimensional complex-shaped medical devices. Alkaline solutions have reportedly been used for etching (Yun, 2000) as well as the functionalization of silicon and silicon oxide (Bruin et al., 1989). In this study, aqueous solutions of KOH and NaOH were used to provide hydroxide ions for alkaline treatment. After pretreatment using the alkaline aqueous solutions under various conditions, the SE sheets were coated with the cross-MPC copolymer (Table 1). In the code of treatments, “K” and “N” indicate KOH and NaOH, respectively. “2.5K” indicates that 2.5 wt% KOH aqueous solution was used. An SE sheet pretreated with oxygen plasma for 2 min was used to compare the effect of oxygen plasma treatment (OPT). Here, “poly-” indicates that the samples were coated with a polymer. The formation of a polymer film and protein adsorption were evaluated by treatment with R6G and FITC-BSA, respectively. First, we have confirmed the fluorescence intensity of the adsorbed R6G on the cross-MPC copolymer film prepared with different concentrations of polymer solutions (Supplementary Figure S2) and understood that the fluorescence intensity of adsorbed R6G is a good indicator to be determined the film formation. Figure 4A shows the fluorescence intensity of R6G for the polymer film on SE pretreated with different alkaline solution conditions and O2 plasma. The fluorescence images of R6G for the polymer film on SE pretreated with different alkaline solution conditions and O2 plasma are shown in Supplementary Figure S3. The results of the fluorescence intensity of adsorbed R6G show that every pretreatment for the polymer coating on SE could form polymer film well. Next, protein adsorption was evaluated by use of FITC-BSA to optimize the parameters for pretreatment, which is one of the factors of blood coagulation. We evaluated the RFI of FITC-BSA absorbed on the coated polymer films under different pretreatment conditions (Table 1). The results are shown in Figure 4B. The amount of the protein on the polymer coated with 2.5K-treated SE (poly-2.5K) was lower than those on the polymer coated with 0.5K-treated SE (poly-0.5K), and with 1.0K-treated SE (poly-1.0K). When the temperature was increased to 45°C, the adsorbed FITC-BSA significantly increased; therefore, we investigated the influence of pretreatment using an alkaline solution by evaluating the surface morphology. Figure 4C shows the optical microscopic images of SE without treatment (SE), as well as with the 2.5K and 2.5K-45 pretreatments. There were many pits on the surface of the 2.5K-45 pretreatment because the SE was etched by an alkaline solution (Brook et al., 2012). It has been reported that rough surfaces have more adsorption of proteins (Maeda et al., 2000); therefore, the etched surface with many pits induced protein adsorption. Moreover, the protein adsorption on the poly-5K was much larger than that on the poly-2.5K. Looking at the polymer-coated with 2.5N-treated SE (poly-2.5N), and polymer-coated with O2 plasma-treated SE (poly-OPT), the fluorescence intensity of adsorbed FITC-BSA on poly-2.5N was the same of that on poly-2.5K, and that on poly-OPT was smaller than on poly-2.5K. The surface morphology of 2.5N-SE is rough than 2.5K-SE by SEM images shown in Supplementary Figure S4, because NaOH is a stronger etchant than KOH (Jaffer and Weitz, 2019). Compared with the surface of OPT-SE and 2.5K-SE, the OPT-SE is smoother than 2.5K-SE (Supplementary Figure S4), but the etching by O2 plasma has occurred more severe than 2.5K. We confirmed that the etching thickness of O2 plasma was around three times higher than that of KOH solution, although the etching occurred in less than several nanometers. In summary of the alkaline solution pretreatment, the increase in surface roughness was caused by an increase in the concentration of the alkaline solution and the reaction temperature. Hence, the 2.5K alkaline treatment was the most appropriate condition for alkaline treatment and was applied for various experiments.
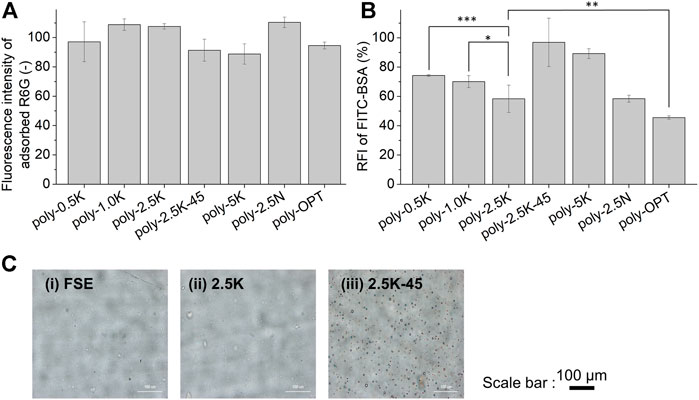
FIGURE 4. Characterizations of the polymer film coated under different conditions (Table 1). Fluorescence intensity of the adsorbed R6G on the polymer film (A); Relative fluorescence intensity of adsorbed FITC-BSA on the polymer-coated surface. (B) Results are presented as mean ± SD (n = 9). (C) Optical microscope images for surface morphology of SEs (i) without treatment, (ii) treated with 2.5 K, (iii) treated with 2.5K–45. *, **, and *** indicate p < 0.05, p < 0.01, and p < 0.001, respectively.
Evaluation of the Formation of a Polymer Film on the Surface in an SE Tube
Here, the applicability of alkaline solution pretreatment on the inner surface of tubular-shaped materials was evaluated. The inner surfaces of the SE tubes (inner diameter: 0.6 cm) were pretreated with 2.5 wt% alkaline solution. Thereafter, the pretreated tubes were coated with the polymer. The polymer-coated tubes were cut into small pieces to evaluate the polymer film coating and protein adsorption (Figure 5A). As shown in Figure 5B, the fluorescence intensities of adsorbed R6G at the different parts of the inner tubes were not significantly different, indicating that the polymer-coated film on the alkaline-treated tube was homogeneous.
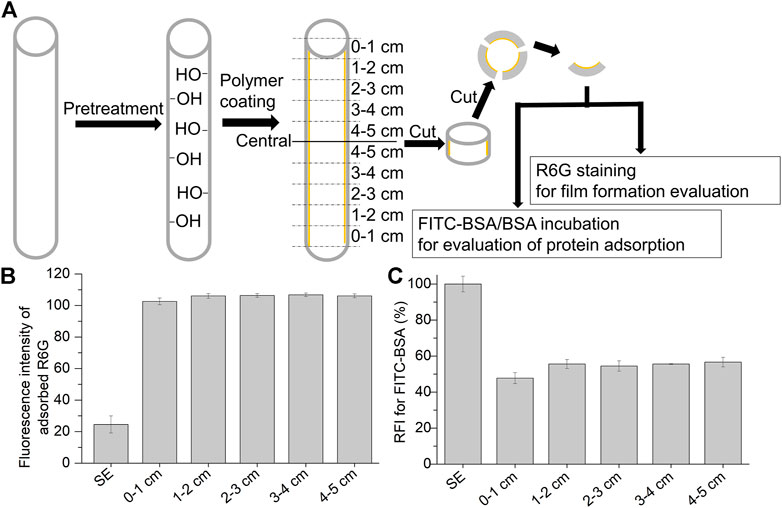
FIGURE 5. Characterization of the polymer coated in silicone elastomer tube (inner diameter: 0.6 cm). (A) Procedure for the characterization of the polymer-coated tubes with an alkaline solution (2.5K). The tubes were cut into small pieces before staining them with Rhodamine 6G (R6G) and FITC-BSA/BSA incubation. Fluorescence intensity of the adsorbed R6G (B) and relative fluorescence intensity of the adsorbed FITC-BSA (C) on the polymer-coated tube with alkaline pretreatment (2.5 K). Results are presented as mean ± SD (n = 3).
Additionally, protein adsorption was evaluated by measuring the RFI with FITC-BSA/BSA incubation. As shown in Figure 5C, after polymer coating on the alkaline pretreated tube, the entire area in the SE tube prevented protein adsorption with a homogeneous polymer film. These results indicate that pretreatment with an alkaline solution is appropriate for the coating of cross-linked copolymers on tubular-shaped materials or medical devices with complex structures.
Evaluating the Long-Term Stability of the Cross-MPC Copolymer Film Under Fluidic Conditions
The stability of the coated copolymer film on the SE surface under fluidic conditions is crucial for applications in medical devices, specifically ALs. Thus, we fabricated a circulating system for evaluating the long-term stability of the polymer films on SE, 2.5K-SE, and OPT-SE. Maeda et. al. established that the upper limit of the blood flow rate in a silicone oxygenator was 5 L/min (Maeda et al., 2000). Therefore, we set the flow rate for the circulating system to 8 L/min, which is high but provides more severe conditions for evaluating the stability of the polymer film. In the circulation system, the sheets were stuck in the tube in series at an interval of 1.0 cm (Figure 2). Because it is necessary for each SE sheet in the tube to be tuned under the same WSS, we performed a CFD simulation to confirm the fluidic condition of the evaluation system. As an important parameter that can affect the results, the WSS forced on the sheets was evaluated, and it is shown in Figure 6A. The color map in Figure 6Ai shows that the fluid jet occurred at the corners of the sheets when the fluid hit the sheet owing to the Coandâ effect. These fluidic jets exhibited high WSS, represented in green-yellow on the sheet. However, Figure 6Aii shows that WSS near the sheet did not have a significant difference and that on the sheets was not affected by the fluidic jets. Additionally, the average value of WSS was 3.2 Pa.
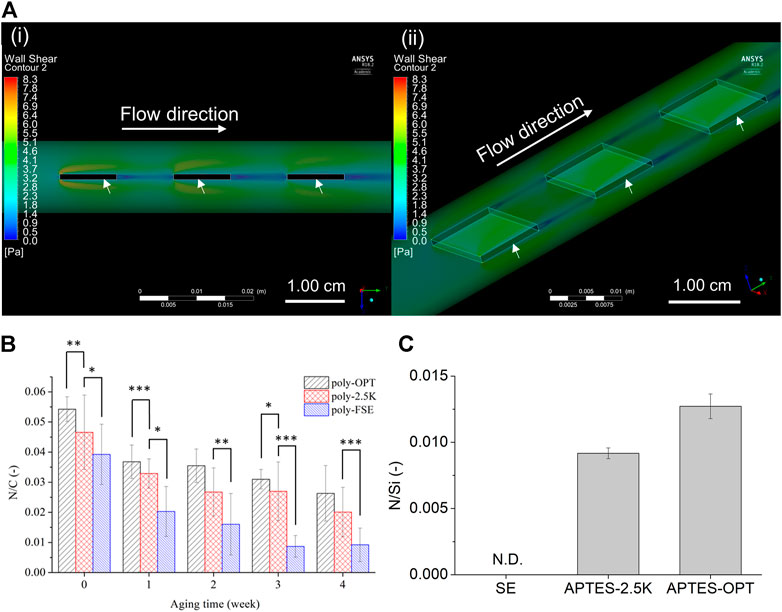
FIGURE 6. Stability evaluation of the coated polymer film on the silicone elastomer (SE) sheet in a fluidic circulating system. (A) Color maps for cross section view of side (i) and top view (ii) of wall shear stress (WSS) by computational fluid dynamics (CFD) model in the tubing (inner diameter = 1.27 cm; outer diameter = 1.59 cm). The arrows indicate the SE sheets. The inlet flow rate was set as 8 L/min without outlet pressure. (B) The N/C (nitrogen comes from the polymer/carbon) ratio of the coated-polymer film on oxygen plasma treated (poly-OPT), 2.5 K alkaline treated (poly-2.5K), and untreated (poly-SE sheets. The data was measured every week for one month. Results are presented as mean ± SD (n = 6). *, **, and *** denote p < 0.05, p < 0.01, and p < 0.001, respectively. (C) Evaluation of the silanol group on the surface of SE. The silanol group was reacted with APTES after treatment with 2.5 K (APTES-2.5K) and oxygen plasma (APTES-OPT), and then N/Si (nitrogen comes from APTES/silicon) was measured via XPS. Results are presented as mean ± SD (n = 3).
Thus, the results of the CFD analysis indicated that the evaluation setup composed of the fluidic circulation system shown in Figure 2B was reliable for evaluating the stability of the coated polymer film.
After aging under fluidic condition, the atomic composition of N in the MPC component, C in the SE, and cross-MPC copolymers were analyzed via XPS. Figure 6B shows the relationship between the atomic ratio of N/C and the aging time. The remaining polymer films on OPT SE (poly-OPT) and 2.5 wt% KOH solution-treated SE (poly-2.5K) at each aging time exhibited a similar decreasing trend. Conversely, the remaining polymer film on the non-treated FSE (poly-SE) significantly decreased after aging for 1 month, and had significant difference from the poly-2.5K. Although the continuous flow was circulated for 4 weeks, the polymer film coated after pretreatment with the alkaline solution and oxygen plasma remained on the SE. Moreover, the N/C ratio for indicating the remaining polymer of poly-OPT and poly-2.5K was slightly decreased owing to the degradation of the polymer.
Because the pretreatment conditions affect the stability of the cross-MPC copolymer coatings, the amount of activated silanol groups on the surface are crucial for maintaining the stability of the coatings. Thus, the modified APTES on the SE surface was analyzed to identify the silanol group that affects the stability of the polymer film on the pretreated SE. In the code of treatments, “APTES-OPT” and “APTES-2.5K″ indicate APTES-conjugated OPT-SE and APTES-conjugated 2.5 K-SE, respectively. The XPS spectrums of Si2p, C1s, O1s, and N1s of APTES-2.5K, APTES-OPT, and SE are shown in Supplementary Figure S5. The N/Si ratio of APTES-2.5K and APTES-OPT increased, as shown in Figure 6C. The treatment using KOH solution and OPT produced silanol groups, which were reacted with APTES. Considering the relationship between the stability of the polymer film and the density of the silanol group, the stability was successfully enhanced by increasing the density of the silanol group. Generally, the 2.5K alkaline treatment obtained sufficient silanol groups on the SE surface to provide conjugatable sites for coating the cross-MPC copolymer.
Evaluating the Hemocompatibility of the Cross-MPC Copolymer Film Before and After Aging by Fluidic Circulating System
Evaluating hemocompatibility is necessary for the clinical application of biomaterials. Plasma protein adsorption is crucial during the first stage of blood coagulation (Jaffer and Weitz, 2019). To discuss the optimizing parameters of alkaline treatment, the protein adsorption has been evaluated by RFI of FITC-BSA. Here, the relative amount of adsorption of human serum albumin (HSA) and human plasma fibrinogen (HPF) was used to know the relationship to platelet adhesion, which correlates the hemocompatibility. The adsorbed protein was evaluated by a quantitation method via micro-BCA method, and the results were shown in Figure 7A. It indicated that relative HSA adsorption on poly-2.5K and poly-OPT were 71 and 69% of that on SE, and the relative HPF adsorption on poly-2.5K and poly-OPT were 47 and 45% of that on SE. Both poly-2.5K and poly-OPT had an ability to reduce the adsorption of HSA and HPF, respectively. In addition, platelet adhesion and activation affect blood coagulation (Jung et al., 2013; Gremmel et al., 2016). The morphology of the adhered platelets on PRP statically-incubated SE, and poly-2.5K-SE surfaces was observed via SEM. SEM micrographs of Figure 7Bi at low magnification showed that were many adhered platelets (262 ± 48 cells/mm2) on the surface of the PRP-incubated SE. As shown in Figure 7Biii, the platelets on the SE surface were adhered and activated. Conversely, only a few inactivated platelets were found on the poly-2.5K-SE surface, as shown in Figures 7Bii,iv. These results indicate that the poly-2.5K-SE inhibited protein adsorption and platelet adhesion.
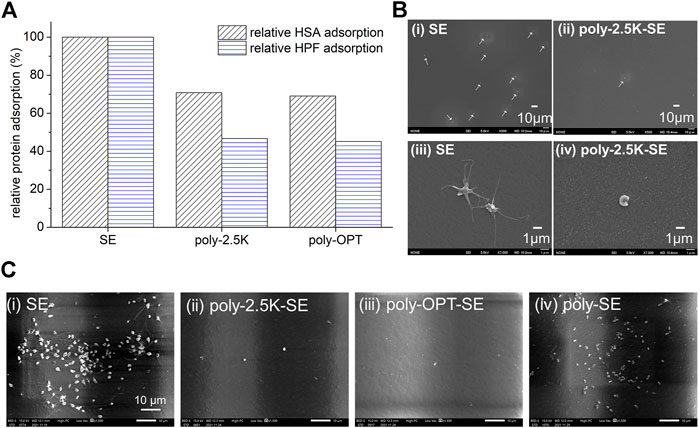
FIGURE 7. (A) Relative HSA and HPF adsorption on the uncoated SE, poly-OPT-SE, as well as the poly-2.5K-SE via µ-BCA assay. (B) Hemocompatibility evaluation under static condition. SEM micrographs of SE and poly-2.5K-SE after incubation in PRP for 1 h at 37°C, (i)-(ii) magnified image (500X), (iii)-(iv) magnified image (7,000X). (C) Hemocompatibility evaluation after aging under fluidic circulation for 4 weeks. SEM micrographs of (i) SE, (ii) poly-2.5K-SE, (iii) poly-OPT-SE, and (iv) poly-SE after incubation in PRP for 1 h at 37°C.
To confirm the hemocompatibility of the coating film of cross-MPC copolymer after aging under fluidic circulating for 4 weeks which are the same condition shown in Figure 6, the SE, poly-OPT-SE, poly-2.5K-SE and poly-SE substrates were incubated in PRP for 1 h at 37°C, and then observed by SEM. The PRP incubated SE was conducted as control. The SEM images of adhered platelet are shown in Figure 7C. The results indicated that the poly-2.5K and poly-OPT still had good inhibition of platelet adhesion after aging by fluidic circulating with PBS for 4 weeks. By contrast, the poly-SE had many adhered platelets on the surface. These results were suggested that high-density of silanol group produced by KOH solution on silicone elastomer improved the stability of polymer film and keep hemocompatibility for long time. Thus, this surface modification can be applied to medical devices for long-term use.
Evaluation of the Hemocompatibility of Hollow Fiber Membrane via One Week In-Vivo Implantation
The hemocompatibility of the polymer-coated membrane made of hollow fiber with KOH pretreatment (denoted poly-HFM) was evaluated via in-vivo implantation in a goat for one week. Thrombus was observed using the naked eye and via SEM. Figure 8A shows a photograph of the chamber, which is burnt red owing to the thrombus on all membranes of HFM without polymer coating. For the polymer-coated HFM, thrombus was observed in some parts. As shown in Figure 8Bi, various blood cells adhered to the poly-HFM surface. However, as shown in Figure 8Bii, a high magnification image showed that the platelets were not activated because the polymer film inhibited the platelet activation. Unlike the polymer-coated hollow fiber, severe blood coagulation was observed on HFM, as shown in Figures 8Biii,iv. Thicker thrombi are induced by the activation of platelets, leading to the formation of the thrombus on the HFM.
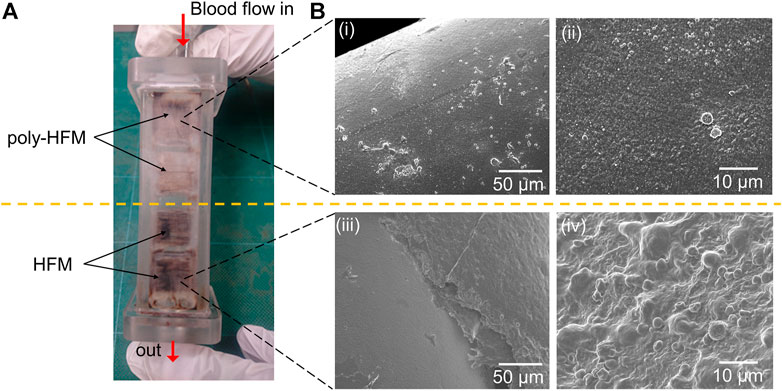
FIGURE 8. (A) Photograph of the chamber with 16 pieces of membranes made of the hollow fiber (HFMs) after one week of implantation in goat. The upper part of the membranes was coated with the polymer film (poly-HFM), and the lower part was left uncoated. (B) The SEM images of the hollow fiber coated with the polymer film (i, ii) and those without polymer coating (iii, iv).
This result was consistent with the results of protein adsorption and platelet adhesion in the in-vitro evaluation, as shown in Figure 7. The activated platelets on SE contributed to blood coagulation and led to burnt red thrombus, as shown in Figure 8A. In addition, the thrombus filled the space between the hollow fibers (Figure 8Biii) because they formed easily under a slow flow rate. These results implied that the blood did not coagulate on the poly-HFM. The poly-HFM treated with KOH solution exhibited good hemocompatibility for in-vivo implantation for one week under fluidic conditions.
Conclusion
In this study, the cross-MPC copolymer was coated via a silane coupling reaction on functionalized SE, which was treated by alkaline solution. The type and concentration of the alkaline solution as well as the reaction temperature were optimized to achieve good polymer film formation and effective inhibition of protein adsorption on the coated polymer films. The increased protein adsorption on the polymer-coated SE was induced by the stronger etching resulting from high concentration and high temperature of KOH and NaOH solutions, which increased the roughness of SE. The 2.5 wt% KOH solution at room temperature performed better compared to other pretreatment conditions, as evidenced by less protein adsorption on polymer-coated surface and good polymer film formation. The optimum conditions for alkaline treatment were applied to the coating on the inner surface of the SE tube. There was no difference in the fluorescence intensity in different areas, which indicated excellent homogeneity of the coated polymer film on the SE surface in tube. Although the polymer films were removed gradually under the shear stress (3.2 Pa) of the fluid condition, the stability of the cross-MPC copolymer film on the SE pretreatment with 2.5K alkaline solution for one month was comparable to that of the polymer film on SE pretreated with OPT. It was established that the increase in the stability of the cross-MPC copolymer film on SE depended on the amount of the silanol groups. The results of platelet adhesion on 4-weeks aging under fluidic condition shows that the stability of cross-MPC copolymer film on the SE pretreated with 2.5K alkaline solution was good enough to inhibit platelet adhesion. For in vivo experiments using a goat with ECMO, the cross-MPC copolymer-coated HFM effectively inhibited blood coagulation for one week under blood flow. In summary, coating film of the cross-MPC copolymer via silane coupling reaction on functionalized silicone elastomer by KOH solution could keep good hemocompatibility for long time. This is beneficial for developing improved interfaces for medical devices.
Data Availability Statement
The raw data supporting the conclusion of this article will be made available by the authors, without undue reservation.
Ethics Statement
The animal study was reviewed and approved by the University of Tokyo Animal Experiment Committee. Written informed consent was obtained from the owners for the participation of their animals in this study.
Author Contributions
F-YC: conceptualization, methodology, data curation, investigation, visualization, writing—original draft; SH: investigation, visualization, writing—review & editing; KU: investigation, validation; YM: investigation, validation; TM: visualization, writing-review & editing; RY: methodology for chamber with hollow fiber membranes, resources for silicone elastomer substrates; TO: investigation for in vivo experiment; MA: supervision, funding acquisition, investigation for surgery of in vivo experiment, writing—review & editing; TI: supervision, writing—review & editing; MT: conceptualization, supervision, funding acquisition, writing—review & editing.
Funding
This work was supported by Japan Agency for Medical Research and Development (AMED) (grant number 18hm0102048h0002) (MT, MA, and RY), and Grants-in-Aid for Scientific Research of Japan Society for the Promotion of Science (grant number 20K17577) (SH). The funds received for open access publication fees from our other grants.
Conflict of Interest
RY was employed by the company Fuji Systems Corporation.
The remaining authors declare that the research was conducted in the absence of any commercial or financial relationships that could be construed as a potential conflict of interest.
Publisher’s Note
All claims expressed in this article are solely those of the authors and do not necessarily represent those of their affiliated organizations, or those of the publisher, the editors and the reviewers. Any product that may be evaluated in this article, or claim that may be made by its manufacturer, is not guaranteed or endorsed by the publisher.
Acknowledgments
A part of this work was conducted at Advanced Characterization Nanotechnology Platform of the University of Tokyo, supported by “Nanotechnology Platform” of the Ministry of Education, Culture, Sports, Science and Technology (MEXT), Japan. We would like to thank Editage (www.editage.com) for English language editing.
Supplementary Material
The Supplementary Material for this article can be found online at: https://www.frontiersin.org/articles/10.3389/fmats.2022.877755/full#supplementary-material
References
Al-Gharabli, S., Mavukkandy, M. O., Kujawa, J., Nunes, S. P., and Arafat, H. A. (2017). Activation of PVDF Membranes through Facile Hydroxylation of the Polymeric Dope. J. Mater. Res. 32 (22), 4219–4231. doi:10.1557/jmr.2017.403
Bausch, G. G., Stasser, J. L., Tonge, J. S., and Owen, M. J. (1998). Behavior of Plasma-Treated Elastomeric Polydimethylsiloxane Coatings in Aqueous Environment. Plasmas Polym. 3 (1), 23–34. doi:10.1023/a:1022577816591
Betit, P. (2018). Technical Advances in the Field of ECMO. Respir. Care 63 (9), 1162–1173. doi:10.4187/respcare.06320
Brook, M. A., Zhao, S., Liu, L., and Chen, Y. (2012). Surface Etching of Silicone Elastomers by Depolymerization. Can. J. Chem. 90 (1), 153–160. doi:10.1139/v11-145
Bruin, G. J. M., Huisden, R., Kraak, J. C., and Poppe, H. (1989). Performance of Carbohydrate-Modified Fused-Silica Capillaries for the Separation of Proteins by Zone Electrophoresis. J. Chromatogr. A 480, 339–349. doi:10.1016/s0021-9673(01)84303-x
De Somer, F., François, K., van Oeveren, W., Poelaert, J., De Wolf, D., Ebels, T., et al. (2000). Phosphorylcholine Coating of Extracorporeal Circuits Provides Natural protection against Blood Activation by the Material Surface. Eur. J. Cardiothorac. Surg. 18 (5), 602–606. doi:10.1016/s1010-7940(00)00508-x
Duy Nguyen, B. T., Nguyen Thi, H. Y., Nguyen Thi, B. P., Kang, D.-K., and Kim, J. F. (2021). The Roles of Membrane Technology in Artificial Organs: Current Challenges and Perspectives. Membranes 11 (4), 239–272. doi:10.3390/membranes11040239
Eynden, F. V., Carrier, M., Ouellet, S., Demers, P., Forcillo, J., Perrault, L. P., et al. (2008). Avecor Trillium Oxygenator versus Noncoated Monolyth Oxygenator: A Prospective Randomized Controlled Study. J. Card. Surg. 23 (4), 288–293. doi:10.1111/j.1540-8191.2008.00682.x
Federspiel, W. J., and Henchir, K. A. (2004). “Lung, Artificial: Basic Principles and Current Applications,” in Encyclopedia of Biomaterials and Biomedical Engineering. Editor G. Wnek (Boca Raton, FL: CRC), 910–921.
Gremmel, T., Frelinger, A. L., and Michelson, A. D. (2016). Platelet Physiology. Semin. Thromb. Hemost. 42 (3), 191–204. doi:10.1055/s-0035-1564835
Huang, X., Wang, W., Zheng, Z., Fan, W., Mao, C., Shi, J., et al. (2016). Surface Monofunctionalized Polymethyl Pentene Hollow Fiber Membranes by Plasma Treatment and Hemocompatibility Modification for Membrane Oxygenators. Appl. Surf. Sci. 362, 355–363. doi:10.1016/j.apsusc.2015.11.236
Iwahashi, H., Yuri, K., and Nosé, Y. (2004). Development of the Oxygenator: Past, Present, and Future. J. Artif. Organs 7 (3), 111–120. doi:10.1007/s10047-004-0268-6
Jaffer, I. H., and Weitz, J. I. (2019). The Blood Compatibility Challenge. Part 1: Blood-Contacting Medical Devices: The Scope of the Problem. Acta Biomater. 94, 2–10. doi:10.1016/j.actbio.2019.06.021
Jung, F., Braune, S., and Lendlein, A. (2013). Haemocompatibility Testing of Biomaterials Using Human Platelets. Clin. Hemorheol. Microcirc. 53 (1–2), 97–115. doi:10.3233/CH-2012-1579
Kouassi, S., Andji, J., Bonnet, J., and Rossignol, S. (2010). Dissolution of Waste Glasses in High Alkaline Solutions. J. Ceram.-Silikáty 54 (3), 235–240.
Larm, O., Larsson, R., and Olsson, P. (1983). A New Non-thrombogenic Surface Prepared by Selective Covalent Binding of Heparin via a Modified Reducing Terminal Residue. Biomater. Med. Dev. Artif. Organs 11 (2–3), 161–173. doi:10.3109/10731198309118804
Maeda, T., Iwasaki, A., Kawahito, S., Nakata, K.-i., Nonaka, K., Linneweber, J., et al. (2000). Preclinical Evaluation of a Hollow Fiber Silicone Membrane Oxygenator for Extracorporeal Membrane Oxygenator Application. ASAIO J. 46 (4), 426–430. doi:10.1097/00002480-200007000-00011
Maul, T. M., Massicotte, M. P., and Wearden, P. D. (2016). “ECMO Biocompatibility: Surface Coatings, Anticoagulation, and Coagulation Monitoring,” in Extracorporeal Membrane Oxygenation. Editor M.S. Firstenberg (IntechOpen), 27–61. doi:10.5772/63888
Motomura, T., Maeda, T., Kawahito, S., Matsui, T., Ichikawa, S., Ishitoya, H., et al. (2003). Development of Silicone Rubber Hollow Fiber Membrane Oxygenator for ECMO. Artif. Organs 27 (11), 1050–1053. doi:10.1046/j.1525-1594.2003.07077.x
Mulvihill, J. N., Faradji, A., Oberling, F., and Cazenave, J.-P. (1990). Surface Passivation by Human Albumin of Plasmaperesis Circuits Reduces Platelet Accumulation and Thrombus Formation. Experimental and Clinical Studies. J. Biomed. Mater. Res. 24 (2), 155–163. doi:10.1002/jbm.820240203
Nagahashi, K., Teramura, Y., and Takai, M. (2015). Stable Surface Coating of Silicone Elastomer with Phosphorylcholine and Organosilane Copolymer with Cross-Linking for Repelling Proteins. Colloids Surf. B: Biointerfaces 134 (1), 384–391. doi:10.1016/j.colsurfb.2015.07.040
Nii, K., Sueyoshi, K., Otsuka, K., and Takai, M. (2013). Zone Electrophoresis of Proteins in Poly(dimethylsiloxane) (PDMS) Microchip Coated with Physically Adsorbed Amphiphilic Phospholipid Polymer. Microfluid. Nanofluid. 14, 951–959. doi:10.1007/s10404-012-1102-8
Ontaneda, A., and Annich, G. M. (2018). Novel Surfaces in Extracorporeal Membrane Oxygenation Circuits. Front. Med. 5, 321. doi:10.3389/fmed.2018.00321
Pfiffer, M., Cormont, P., Fargin, E., Bousquet, B., Dussauze, M., Lambert, S., et al. (2017). Effects of Deep Wet Etching in HF/HNO3 and KOH Solutions on the Laser Damage Resistance and Surface Quality of Fused Silica Optics at 351 Nm. Opt. Express 25 (5), 4607–4620. doi:10.1364/oe.25.004607
Pieri, M., Turla, O., Calabrò, M., Ruggeri, L., Agracheva, N., Zangrillo, A., et al. (2013). A New Phosphorylcholine-Coated Polymethylpentene Oxygenator for Extracorporeal Membrane Oxygenation: A Preliminary Experience. Perfusion 28 (2), 132–137. doi:10.1177/0267659112469642
Raasveld, S. J., Delnoij, T. S. R., Broman, L. M., Lansink-Hartgring, A. O., Hermans, G., De Troy, E., et al. (2021). Extracorporeal Membrane Oxygenation in Patients with COVID-19: An International Multicenter Cohort Study. J. Intensive Care Med. 36 (8), 910–917. doi:10.1177/08850666211007063
Reser, D., Seifert, B., Klein, M., Dreizler, T., Hasenclever, P., Falk, V., et al. (2012). Retrospective Analysis of Outcome Data with Regards to the Use of Phisio®-, Bioline®- or Softline®-Coated Cardiopulmonary Bypass Circuits in Cardiac Surgery. Perfusion 27 (6), 530–534. doi:10.1177/0267659112454558
Sanford, Z., Madathil, R. J., Deatrick, K. B., Tabatabai, A., Menaker, J., Galvagno, S. M., et al. (2020). Extracorporeal Membrane Oxygenation for COVID-19. Innovations (Phila) 15 (4), 306–313. doi:10.1177/1556984520937821
Seo, J.-H., Shibayama, T., Takai, M., and Ishihara, K. (2011). Quick and Simple Modification of a Poly(dimethylsiloxane) Surface by Optimized Molecular Design of the Anti-biofouling Phospholipid Copolymer. Soft Matter 7 (6), 2968–2976. doi:10.1039/c0sm01292k
Suhara, H., Sawa, Y., Nishimura, M., Oshiyama, H., Yokoyama, K., Saito, N., et al. (2001). Efficacy of a New Coating Material, PMEA, for Cardiopulmonary Bypass Circuits in a Porcine Model. Ann. Thorac. Surg. 71 (5), 1603–1608. doi:10.1016/s0003-4975(01)02466-3
Suma, K., Tsuji, T., Takeuchi, Y., Inoue, K., Shiroma, K., Yoshikawa, T., et al. (1981). Clinical Performance of Microporous Polypropylene Hollow-Fiber Oxygenator. Ann. Thorac. Surg. 32 (6), 558–562. doi:10.1016/s0003-4975(10)61798-5
Wang, J.-H., Bartlett, J. D., Dunn, A. C., Small, S., Willis, S. L., Driver, M. J., et al. (2005). The Use of Rhodamine 6G and Fluorescence Microscopy in the Evaluation of Phospholipid-Based Polymeric Biomaterials. J. Microsc. 217 (3), 216–224. doi:10.1111/j.1365-2818.2005.01453.x
Wendel, H., and Ziemer, G. (1999). Coating-techniques to Improve the Hemocompatibility of Artificial Devices Used for Extracorporeal Circulation. Eur. J. Cardiothorac. Surg. 16 (3), 342–350. doi:10.1016/s1010-7940(99)00210-9
Yun, M. (2000). Investigation of KOH Anisotropic Etching for the Fabrication of Sharp Tips in Silicon-On-Insulator (SOI) Material. J. Korean Phy. Soc. 37 (5), 605–610. doi:10.3938/jkps.37.605
Zhang, W., Kalulu, M., Wang, X. H., Xia, X. K., Han, X. L., and Jiang, Y. (2018). Reverse Hydrophobic PDMS Surface to Hydrophilic by 1-Step Hydrolysis Reaction. Polym. Adv. Technol. 29, 1–7. doi:10.1002/pat.4319
Zimmermann, A. K., Weber, N., Aebert, H., Ziemer, G., and Wendel, H. P. (2007). Effect of Biopassive and Bioactive Surface-Coatings on the Hemocompatibility of Membrane Oxygenators. J. Biomed. Mater. Res. 80B (2), 433–439. doi:10.1002/jbm.b.30614
Zwaal, R. F. A., and Hemker, H. C. (1982). Blood Cell Membranes and Haemostasis. Pathophysiol. Haemos Thromb. 11 (1), 12–39. doi:10.1159/000214638
Gloassary
ACT activated clotting time
AL artificial lungs
APTES 3-aminopropyltrimethoxysilane
CAD computer-aided design
CCD charge-coupled device
CFD computational fluid dynamics
cross-MPC copolymer phosphorylcholine-based copolymer with an organosilane
ECMO extracorporeal membrane oxygenation
FITC-BSA fluorescein isothiocyanate-labeled BSA
GA glutaraldehyde
HSA human serum albumin
HFM hollow fiber membrane
HPF human plasma fibrinogen
KOH potassium hydroxide
MPC 2-methacryloyloxyethyl phosphorylcholine
MPTMSi 3-methacryloxypropyl trimethoxysilane
MPTSSi 3-(methacryloyloxy) propyl-tris(trimethylsilyloxy)silane
NaOH sodium hydroxide
OPT oxygen plasma treatment
PC phosphorylcholine
PBS phosphate-buffered saline
PRP platelet-rich plasma
PP polypropylene
PMP polymethyl pentene
R6G Rhodamine 6G
RFI relative fluorescence intensity
SDS sodium dodecyl sulfate
SE silicone elastomers
SEM scanning electron microscopy
WSS wall shear stress
XPS X-ray photoelectron spectroscopy
Keywords: silicone elastomer, phosphorylcholine-based copolymer, surface modification, alkaline solution, artificial lung
Citation: Chou F-Y, Hara S, Uchida K, Matsuo Y, Masuda T, Yokoi R, Ono T, Anraku M, Isoyama T and Takai M (2022) Functionalized Silicone Elastomer via Alkaline Solution to Coat Phosphorylcholine-Based Copolymer Containing Organosilane to Improve Hemocompatibility for Medical Devices. Front. Mater. 9:877755. doi: 10.3389/fmats.2022.877755
Received: 17 February 2022; Accepted: 11 April 2022;
Published: 29 April 2022.
Edited by:
Sofia Rangou, Helmhlotz-Zentrum hereon, GermanyCopyright © 2022 Chou, Hara, Uchida, Matsuo, Masuda, Yokoi, Ono, Anraku, Isoyama and Takai. This is an open-access article distributed under the terms of the Creative Commons Attribution License (CC BY). The use, distribution or reproduction in other forums is permitted, provided the original author(s) and the copyright owner(s) are credited and that the original publication in this journal is cited, in accordance with accepted academic practice. No use, distribution or reproduction is permitted which does not comply with these terms.
*Correspondence: Madoka Takai, dGFrYWlAYmlzLnQudS10b2t5by5hYy5qcA==