- 1School of Stomatology, Zhejiang Chinese Medical University, Hangzhou, Zhejiang, China
- 2Center for Plastic and Reconstructive Surgery, Department of Stomatology, Zhejiang Provincial People’s Hospital, Hangzhou, Zhejiang, China
Regeneration of soft tissues, especially those requiring complex vascularization, is a major challenge in the field of tissue engineering. The current types of tissue engineering scaffolds include sponges, electric spinning silk, hydrogels, and 3D printed biomaterials. Among them, hydrogels have the unique property of mimicking extracellular matrix (ECM), which can provide a relatively stable microenvironment for cellular activities and facilitate cell adhesion, proliferation, and differentiation; thus, have become a promising scaffold. In this paper, we present a review of the commonly used types of natural hydrogels and their applications as scaffolds in tissue vascularization. First, we enumerate the importance and advantages of several types of commonly used hydrogels of natural origin in terms of fabricating vascularized tissues or organs. Second, we discuss two different formation modalities of blood vessels, as well as natural hydrogel-based vascularization strategies, including carrying growth factors, stem cell delivery, special scaffold structures and pharmaceutical-enhanced strategy. In addition, we describe the crosslinking strategies of hydrogels as scaffolds for regeneration of vascularized soft tissues, as well as the factors affecting it. Finally, new insights are provided for the development of natural hydrogel-based vascularized soft tissue regeneration research.
1 Introduction
The treatment of soft tissue defects of varying degrees of severity caused by trauma, surgery, burns, cancer and infection has been a hot topic (Wang et al., 2021). Clinically, larger soft tissue defects often cause serious tissue structure damage and organ dysfunction in the oral and maxillofacial regions, which bring great disturbance to patients’ daily life, work, and mental health (Cheng et al., 2016).
Soft tissues are one of the more important types of tissues in the human body, including skin, muscles, ligaments, synovium, articular cartilage, tendons, and so on Patel et al. (2018). During the process of soft tissue repair, vascularization is an extremely important event. The presence of an appropriate vascular system is vital for the influx of cells, growth factors, signaling molecules, nutrients, oxygen, and drugs to the site of injury or implanted tissue to support the healing process (Chandra and Atala, 2019). Without proper vascularization, tissue growth is limited by the diffusion of oxygen through the tissue (approximately 200 μm) (Novosel et al., 2011). Therefore, successful vascularization is a prerequisite for soft tissue repair.
The commonly used methods for soft tissue defect repair are divided into three categories: autologous tissue transplantation, allogeneic tissue transplantation and xenotransplantation (Berthiaume et al., 2011). While, autologous tissue transplantation is the most used and effective method of soft tissue reconstruction, as it has fewer complications and no risk of immune rejection; however, it does involve the potential for a second surgical site (Emerick and Teknos, 2007; Schrementi et al., 2008). Allogeneic tissue transplantation carries the risk of immune reaction, and the availability of tissue is limited. Xenogeneic tissue transplantation is associated with risks such as an unstable donor source and potential postoperative infection (Bonferoni et al., 2021).
In recent years, rapidly developing tissue engineering techniques have provided new approaches for soft tissue repair and reconstruction. The three basic elements of tissue engineering are: the seed cells, the signaling factors and scaffold materials (Matai et al., 2020). The choice of scaffold materials is crucial for the success of tissue regeneration. Suitable scaffold materials not only accurately mimic the structure of the ECM, but also promote the adhesion and proliferation of seed cells around the defective area (Zhao et al., 2013). Currently, several scaffold materials of natural, synthetic, and biological origins have been widely used in tissue engineering research, including sponges, electrostatic spinning, hydrogels, and other 3D-printed biomaterials (Zhang et al., 2023; Yadav et al., 2024).
Hydrogel is a non-water-soluble three-dimensional (3D) polymer network structure made by crosslinking physically or chemically, which has the unique property of mimicking ECM and can provide a relatively stable microenvironment for cellular activities (Lin et al., 2021). Hydrogels can be classified into natural and synthetic hydrogels based on their origin. Natural hydrogels generally originate from within living organisms and have good biocompatibility and bioactivity (Cheung et al., 2014). Synthetic hydrogels are made of various macromolecular monomers crosslinked by chemical reactions and have excellent mechanical properties. However, they have the disadvantages of poor degradation performance and lack of bioactivity, which restrict the application of this type of material in soft tissue (Delaittre et al., 2015; Zhu et al., 2018a). As mentioned before, the establishment of a vascular network remains a priority in soft tissue repair. Using appropriate biomaterials as scaffolds to promote vascularization is the core of these efforts. In addition, ensuring the growth of the vascular network at the center of the scaffold, good microcirculation in the scaffold material, and the maturation of the vascular network are crucial for achieving long-term vascularization (Fleischer et al., 2020).
The unique internal structure of hydrogel allow it to absorb and retain a large amount of water. Due to its good biocompatibility and biodegradability, natural hydrogel can be directly used as a carrier material for cells, cytokines, and small molecules (Yue et al., 2020). These biologically active substances can remain active within the hydrogel and slowly released at the scaffold implantation site. Moreover, the interconnected pores within hydrogel networks facilitate the diffusion of molecules, thereby meeting the vascular system’s requirement to transport molecules from blood vessels to tissues (Vedadghavami et al., 2017; Gutierrez et al., 2022). Thus, using natural hydrogel as a scaffold can achieve drug delivery, cell encapsulation, and local vascularization. As a soft material, hydrogel can also be prepared in any shape according to the specific application, precisely matching the defect site and reducing inflammatory reactions with surrounding cells and soft tissues (Buwalda et al., 2017). As a scaffold material, hydrogel can not only provide space for cell infiltration and vascular growth through its own structure, but also modify the inflammatory response by delivering different substances, promoting angiogenesis and regeneration, and facilitating tissue remodeling, thereby enabling effective soft tissue repair (Li et al., 2019; Shi et al., 2023).
In summary, this study aims to summarize commonly used natural hydrogels in soft tissue engineering, outline those that have been studied for inducing vascularization strategies and explore current limitations along with potential strategies for future optimization. We first introduce five common types of natural hydrogels and their unique properties. We then briefly summarize vascularization and angiogenesis as the two primary mechanisms of vascular regeneration. Furthermore, we discuss the main vascularization strategies for soft tissue repair, emphasizing the importance of natural hydrogels as biomaterial scaffolds in the vascularization process. Finally, we explore different crosslinking strategies for hydrogels and briefly discuss how these crosslinking modes influence vascularization (Figure 1).
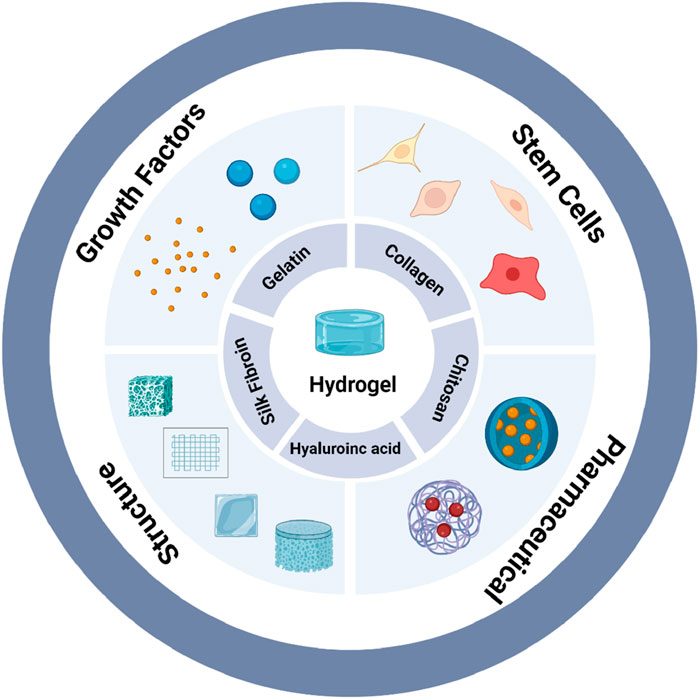
Figure 1. Natural hydrogels with multiple functions for vascularization (created with BioRender.com).
2 Common types of natural hydrogels
Natural polymers encompass a range of polysaccharides and proteins predominantly sourced from animals, microorganisms, and plants. They are widely used due to their superior biocompatibility, biodegradability, and mechanical properties compared to synthetic polymers. Natural hydrogel is a type of hydrogel obtained by modifying and cross-linking natural polymers. To date, various natural hydrogels have been utilized in vascularization of soft tissue repair. We provide a concise overview of the advantages and disadvantages associated with the suitability of different hydrogels for vascularization in soft tissues (Table 1).
2.1 Gelatin
Gelatin is a collection of peptides and proteins produced through the partial hydrolysis of collagen. It possesses adjustable biodegradability, excellent biocompatibility, and notable viscoelasticity. Because its degradation products are non-toxic, it is commonly used clinically as a hemostatic sponge and pharmaceutical excipient (Pennacchio et al., 2018). Gelatin retains many natural properties of collagen, including matrix metallopeptidase (MMP)-sensitive sites and Arg-Gly-Asp (RGD) adhesion motifs, which regulate cell-mediated matrix degradation and cell attachment, respectively (Yang et al., 2019b). Moreover, gelatin contains matrix metalloproteinase (MMP) sequences, which are endopeptidases that facilitate enzymatic degradation, playing a critical role in tissue rehabilitation and wound closure (Klotz et al., 2016).
The use of gelatin as a scaffold material dates back to the 1990s, when Scherzer et al. developed gelatin methacrylate (GelMA) by modifying the reactive side groups of gelatin using glyceryl methacrylate (Scherzer et al., 1997), and in 2000, Van Den Bulcke et al. found that free radical polymerization crosslinking induced by UV photoinitiation in the presence of methacrylate transformed it from a liquid to a solid state, endowing it with new physical properties (Van Den Bulcke et al., 2000). A series of GelMA hydrogels with varying physical properties can also be obtained by adjusting fabrication parameters such as polymer concentration, initiator concentration and UV irradiation conditions. This discovery has made GelMA an attractive and cost-effective material for biological applications.
2.2 Collagen
Collagen is an important component of the dermis of the skin, accounting for 25%–30% of the total dry weight of mammals (Collagen in Health and Disease, 1978). There are at least 28 types of collagens (Shoulders and Raines, 2009), and common types include type I, type II, type III, and type V collagen (Wakitani et al., 1998). Among these, type I collagen is the most abundant in human skin. Type I collagen is widely sourced, and it is often obtained from animal tissues such as pig skin, bovine Achilles tendon, fish skin and swim bladder, etc. The main methods of collagen preparation are extraction from animal connective tissues by purification, acid or alkaline hydrolysis, and enzymatic extraction (Antoine et al., 2014). Collagen is also a major component of ECM. It can self-assemble into a triple-helical fibrous structure under physiological conditions, giving collagen great tensile strength and durability (Gajbhiye and Wairkar, 2022). It can also provide structural support for human skin, joints, and bones (Müller et al., 2005). At the microscopic level, collagen can provide support to cells by interacting with different receptors on the cell surface promoting cell adhesion and growth (Zeltz and Gullberg, 2016).
2.3 Chitosan
Chitosan is an aminated, linear, naturally occurring polysaccharide consisting of a deacetylation unit (D-glucosamine) and an acetylation unit (N-acetyl-D-glucosamine). It is obtained from the chemical or enzymatic hydrolysis of chitosan deacetylation, either under highly alkaline conditions or with specific enzymes like chitin deacetylase (Xu et al., 2012). Chitosan is the second most abundant natural polymer in the world after cellulose and is found in the exoskeletons of insects (e.g., beetle cocoons, cuticles, and egg cases), the cell walls of fungi (e.g., Aspergillus cloudyi and Trichoderma reesei), the shells of crustaceans (e.g., crabs and shrimps), and in centric diatoms (e.g., algae and riverine seaweeds) (Soares et al., 2016). Chitosan-based hydrogels exhibit favorable properties for a variety of biomedical applications, such as drug delivery, cell therapy and single-cell analysis (Ul-Islam et al., 2019). The abundant hydroxyl and amine functional groups in the chitosan structure can be used to crosslink polymer chains to form robust hydrogels (Xiao et al., 2016).
2.4 Silk fibroin
Silk is a high-quality natural fiber obtained from spinning silkworms. It is mainly composed of sericin and fibroin. Among these, fibroin is the main component of silk, accounting for about 75%. The fibroin molecular chain consists of three parts: the heavy chain (H chain), the light chain (L chain) and P25 protein, with a molar ratio of 6:6:1. The molecular chain of the fibroin contains 18 amino acids with glycine (Gly), alanine (Ala) and serine (Ser) accounting for approximately 80% or more of the total composition. Fibroin is composed of repeating units of six amino acids (Gly-Ala-Gly-Ala-Gly-Ser), which form large hydrophobic proteins capable of creating antiparallel β-sheet structures connected by hydrogen bonding, van der Waals forces, and hydrophobic interactions (Koh et al., 2015). Fibroin is a Food and Drug Administration (FDA)-approved polymer for biomedical applications such as drug delivery, surgical sutures, and regenerative tissues, and it is more beneficial to the in vivo environment compared to synthetic polymer fibers. Among various proteins, fibroin shows particular promise for drug delivery and provides synergistic effects in enhancing therapeutic outcomes for diseases (Zhu et al., 2018b).
2.5 Hyaluronic acid
Hyaluronic acid (HA) is a natural, non-sulphated, linear polyanionic glycosaminoglycan consisting of N-acetyl-D-glucosamine and D-glucuronic acid as alternating linkages of disaccharide units (Kogan et al., 2006). Hyaluronic acid was first discovered in 1934 by K. Meyer and John W. Palmer, who succeeded in isolating this substance from the vitreous body of bovine eyes (Meyer et al., 1940). Hyaluronic acid is also a major component of ECM, interacting with the cell surface receptor CD44 to promote the proliferation, migration, and differentiation of stem cells during tissue development. It is also an important component of soft tissue regeneration and wound healing (Voigt and Driver, 2012; Yee et al., 2011).
Different molecular weights of hyaluronic acid have different biological activities, Ke et al. found that low molecular weight hyaluronic acid can recruit endothelial cells to the site of vascular injury, participate in their proliferation and migration, and acid in tissue adaptation to vascularization (Ke et al., 2013). Hyaluronic acid-based hydrogels can be used as suitable biomaterials for tissue engineering scaffolds due to its good biocompatibility, degradability, softness, and viscoelasticity.
3 Regeneration mechanism of vascularization approach
The formation of blood vessels consists of two main modalities: Vascularization and Angiogenesis. Vascularization is defined as the differentiation of precursor cells (angiogenic cells) into endothelial cells and the reformation of the primitive vascular network. It is also the process by which blood vessels are created from scratch. Angiogenesis refers to the maturation and enlargement of the original blood vessels stimulated by certain factors, such as endothelial growth factor, hypoxia, or nitric oxide. From a microscopic perspective, angiogenesis is more like hemodynamically mediated vascular remodeling. This process mainly involves the proliferation and migration of endothelial cells, which gradually form vascular rings. Then the vascular rings interconnected with each other to form a neointimal lumen (Potente et al., 2011) (Figure 2). Migration of vascular endothelial cells is an important part of homeostasis and angiogenesis in vivo (Hirose et al., 2021). It is influenced by a variety of stimulator factors, including Vascular Endothelial Growth Factor (VEGF), basic Fibroblast Growth Factor (bFGF), Platelet-Derived Growth Factor (PDGF) and Fibroblast Growth Factor-2 (FGF-2) (Bai et al., 2018). These factors stimulate the proliferation and migration of vascular endothelial cells in a direct or indirect manner, thus promoting the stability and maturation of vascularization. Table 2 illustrates the common applications of the natural-origin hydrogel as tissue engineering scaffolds.
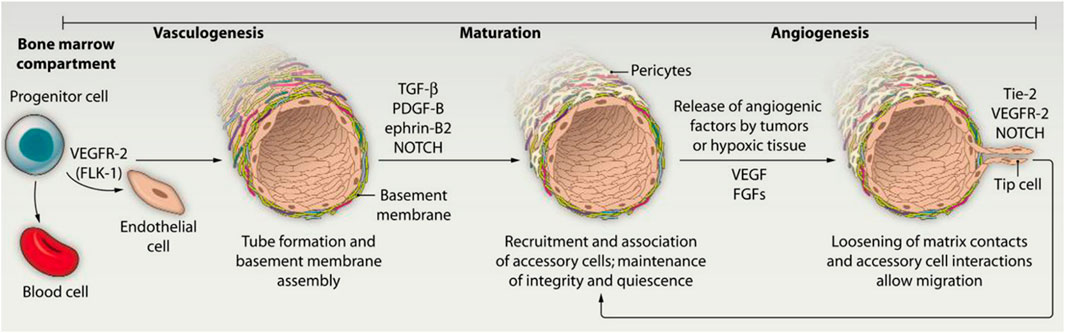
Figure 2. Two distinct mechanisms of blood vessel formation (Bae et al., 2012).
4 Vascularization strategies for soft tissue repair
4.1 Hydrogel as a carrier for the release of growth factors during vascularization
Angiogenic factors play a crucial regulatory role in the formation of a mature vascular network during the repair process of soft tissue defects (Laschke and Menger, 2012; Cheng et al., 2020). Growth factors are peptides that can regulate cell growth and activate certain cell-specific functions by binding to specific receptors on the cell membrane (Barrientos et al., 2008). Among different types of growth factors, VEGF is the most important regulator of vascularization. The level of VEGF is closely related to angiogenesis (Tateishi, 2005). By binding with the receptors for VEGF on the cell membrane, VEGF can control endothelial cell growth, differentiation, and migration during vascularization and promote the formation of tubular structures (Rahimi, 2006).
In conventional growth factor delivery systems, it is difficult to integrate the dynamic and heterogeneous nature of the biochemical microenvironment of neoplastic tissues. In order to response this inability, Rana et al. used aptamer complementary sequence (CS) hybridization to trigger VEGF165 release. They chose GelMA hydrogels as a growth factor delivery platform. Co-culture of aptamers with HUVECs and Mesenchymal Stem Cells (MSCs) 3D in GelMA hydrogels was found to selectively release VEGF through spatiotemporal control, with no non-specific leakage throughout the release process. Thereby directing cellular responses and manipulating lumen-like micro vessel network formation (Rana et al., 2022).
In situ bioprinting has been a new trend in directly printing scaffolding materials into the defect site. It can minimize the preparation time of scaffolds and promote rapid wound closure (Singh et al., 2020). With the convenience of in-situ crosslinking and the strong adhesive force of GelMA hydrogel, Nuutila et al. (2022) used a custom-made handheld printer is implemented to finely print gelatin-methacryloyl (GelMA) hydrogel containing vascular endothelial growth factor (VEGF) into the wounds (Figure 3). The result of their research suggested that the in situ GelMA crosslinking induces a strong scaffold adhesion and enables printing on curved surfaces of wet tissues without the need for any sutures. The scaffold is further shown to offer a sustained release of VEGF, enhancing the migration of endothelial cells in vitro.
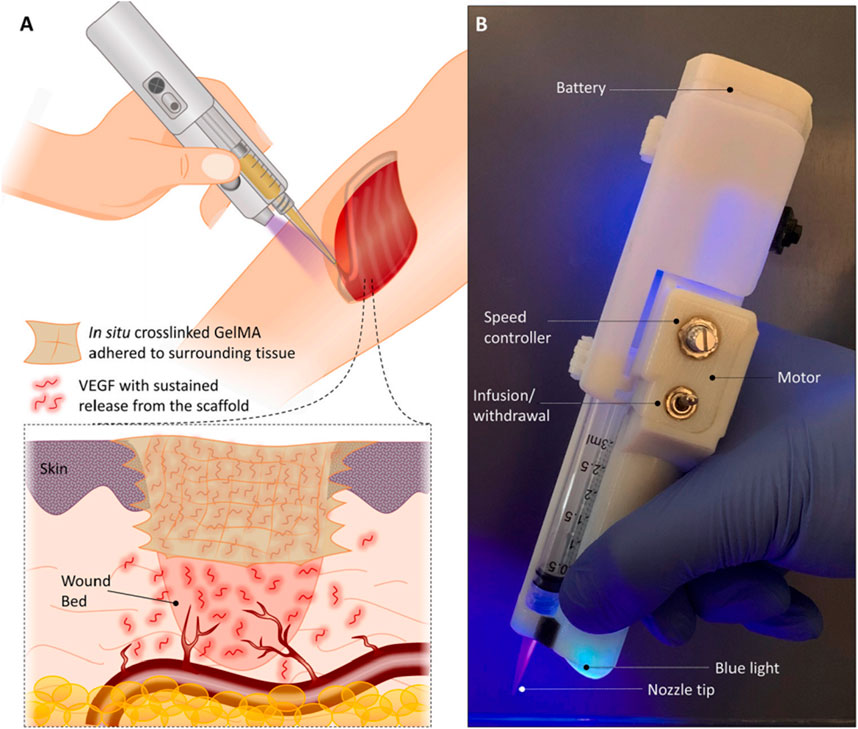
Figure 3. In vivo printing of growth factor-eluting hydrogel for wound healing applications. (A) Schematic representation of the in vivo printing strategy. GelMA precursor supplemented with VEGF is extruded using a handheld printer and photo-crosslinked in situ. This approach enables the treatment of a wound with irregular shapes and on curved surfaces. (B) The portable handheld printer used in this study. The device allows the adjustment of deposition rate and in situ photo-crosslinking with an integrated UV light (Nuutila et al., 2022).
Owing to their unique performance and adjustable characteristics, natural hydrogels are increasingly used in vascularized soft tissue repair. By engineering hydrogels into various scaffold types and incorporating VEGF, it is possible to achieve a controllable and long-term release of VEGF in the defect area. This facilitates the internal vascularization of the scaffold and promotes host tissue grow into the scaffold, thereby completing the repair of soft tissue. Taken together, the synergistic effect of VEGF offers great promise for soft tissue repair scaffolds and vascularized soft tissue regeneration.
4.2 Hydrogel provides microenvironment for stem cells during vascularization
In addition to the direct application of growth factors, stem cell-based vascularization strategies have gradually been emphasized by researchers (Melchiorri et al., 2014). Stem cells play an important role in vascularization by being able to transform into other cell types and proliferate to accelerate wound healing, as well as displaying superior paracrine capacity to initiate the healing process by releasing factors such as cytokines, chemokines, and growth factors (Chamberlain et al., 2007).
However, stem cells affect the function, state and signaling of the cells themselves during and after transplantation due to alterations in the microenvironment (Guvendiren and Burdick, 2010). Hydrogel can precisely provide a stable and reliable microenvironment for stem cells (Burdick et al., 2016). In addition to acting as a barrier, hydrogel can provide water at the defect site to form a moist microenvironment, while hydrogel with appropriate viscoelasticity can maximally mimic the composition and structure of the ECM to provide a good, confined environment for soft tissue defect sites (Jeon et al., 2013).
In the current study, it is essential to find a dermal substitute containing mesenchymal stem cells that is easy to use and can stimulate angiogenesis. Eke et al. (2017) integrated Adipose-derived Stem Cells (ADSC) into photocrosslinked gelatin/hyaluronic acid hydrogels, which provided a suitable microenvironment for ADSC proliferation. The results of in vivo experiments showed that hydrogels loaded with stem cells increased vascularization up to 3 times compared to hydrogels without stem cells. The pro-angiogenic effect of ADSC was confirmed, which is essential for promoting wound healing and improving the survival of tissue-engineered cells. The proposed approach in this study would be most clinically relevant in chronic nonhealing wounds to stimulate wound healing. Previous studies have shown that the GMSCs can enhance wound healing (Jiang et al., 2015). However, the GMSC-derived exosomes have not yet been isolated and characterized. Shi et al. isolated exosomes derived from GMSCs and used freeze-dried Chitosan/Silk fibroin protein hydrogel sponges as carriers for exosomes derived from gingival mesenchymal stem cells (GMSCs), and the combination of exosomes and hydrogel could effectively promote healing of skin wounds in a diabetic rat model by facilitating the re-epithelialization, deposition, and remodeling of collagen, as well as angiogenesis and neurite growth (Shi et al., 2017). Recent tissue engineering approaches have been directed toward initiating and controlling cell autonomous modes of organogenesis at the microscopic and macroscopic scales while integrating a vascular system to sustain cell viability and function (Shao and Fu, 2022). Escudero et al. developed a method that promotes the three-dimensional self-assembly of hydrogel spheres into pre-vascularized human beige fat-like organs. The method was based on GelMA hydrogels and stromal vascular fraction (SVF) cells from human white adipose tissue as a source of endothelial progenitor cells and pluripotent adipose-derived mesenchymal stem cells (ASC). By building a controlled biochemical and mechanical environment that enabled the development of ASC towards a beige adipocyte lineage, as well as modulating GelMA porosity and mechanical properties through tumor growth factor (TGF-β) pathway inhibition and embedding of individual spheroids by varying the percentage of gelatin, vasculature development was facilitated at the microscale, and vascularized centimeter sized beige adipose microtissues were able to be generated at the macroscopic scale (Escudero et al., 2023).
4.3 Different pore structure design of vascularization hydrogel scaffold
The ECM is a complex heterogeneous spatial structure consisting of multiple macromolecules around the cell, spanning the macro-, micro- and nano scales. Natural hydrogels can mimic the structure of ECM, based on which the modification of hydrogels can enable cells to sense the spatial structural changes of the surrounding microenvironment and make feedback to adjust their own gene transcription and protein expression (Naleway et al., 2015). Therefore, spatial structural features are important considerations for the preparation of tissue repair hydrogels (Wegst et al., 2015). Hydrogel can be processed into different types of multi control scaffolds, such as membrane, sponge, foam, and can also be made into scaffolds with controllable pore size through the latest 3D printing technology (Chevalier et al., 2008). Porosity and an appropriate surface-to-volume ratio provides a suitable environment for ECM secretion and nutrient supplies to the cells (Ouriemchi and Vergnaud, 2000).
Appropriate pore structure of soft tissue repair scaffold species can enhance the permeability of the matrix and facilitate the diffusion of nutrients and wastes, which is critical for cell adhesion, growth infiltration, and the formation of new tissues (Vepari and Kaplan, 2007; Yang et al., 2009). Yang et al. prepared a novel filipin protein (SF)/hyaluronic acid (HA)/sodium alginate (SA) composite scaffold by using the freeze-drying method. SF/HA/SA scaffolds were soft and elastic with an average pore size of 93 μm and a porosity of 92%. The ability to promote soft tissue repair was verified using a rat whole-layer skin burn model, and the results indicated that the SF/HA/SA group had the fastest healing rate, and on the 21st day, the trauma area was only 3% Fluorescence staining results indicated that on the 14th day, the SF/HA/SA group was rich in collagen deposition compared to the control group, and obvious new tissue formation was visible (Yang et al., 2009).
As mentioned before, the ECM structure spans the macroscale, microscale and nanoscale, and the current tissue engineering scaffolds mostly work on the macro- and microscale. Few studies have been reported on the nanoscale microporous structure of the scaffolds. Therefore, Sun et al. established a kind of membrane by non-solvent-induced phase separation to achieve the direct and controllable construction of porous regenerated sericin (RSF) membranes. The prepared RSF film had good stretchability and excellent biocompatibility, and its tunable nanopore structure not only improved air permeability, but also enhanced the wound healing speed. The results of animal experiments showed that the wound was almost completely healed by the 21st day, and a large amount of regenerated epidermis and hairs covered the wound. The HE staining showed greater epidermal remodeling and significantly more vascularization in the RSF membrane-treated wounds compared to the control group (Sun et al., 2023).
Porous scaffolds have shown great potential for directing the spatial organization and proliferation of fibroblasts and dermal follicular cells in vitro, indicating good biocompatibility (Dong et al., 2021). However, drawbacks such as poor mechanical properties, rapid fluid loss, and progression of postoperative infections limit the efficiency and clinical application of scaffolds. Therefore, scaffolds need to be optimized for better all-layer wound regeneration. Shen et al. prepared a non-porous membrane (SASFm) by casting to protect the wound as an epidermal layer, then prepared an underlying porous material (PM) scaffold by freeze-drying to mimic the structure of ECM. The results showed that at day 14, the trabecular granulation tissue in the PM@SASFm group was thickened, re-epithelialization was more complete, the dermis and epidermis were thinner, and the collagen matrix was organized and had stronger tensile strengths, which were more like those of normal skin tissues. Immunohistochemical staining showed that at day 7, many small blood vessels were formed in the PM@SASFm group, compared with very few blood vessels that could be found in the control group (Shen et al., 2022).
4.4 Pharmaceutical-enhanced vascular growth
Using hydrogels as drug delivery platforms is a hot topic among researchers. It offers several advantages (Abd Ellah et al., 2018), including prolonged drug release, reduced drug dosage, enhanced bioavailability, and minimized side effects. Additionally, the entangled polymer network within hydrogels can absorb significant quantities of water as well as hydrophilic drugs without solubilization, thus shielding the drug molecules from adverse conditions (Zhang et al., 2015).
The integration of hydrogels with antibiotic eluting nanofibers can be used as temporary framework for pulp tissue regeneration. It erodes infection and supports new tissue formation while migration of host cells from the root apex, and then promotes the complete and effective regeneration of the pulp-dentin complex. Dubey et al. developed a hydrogel system to obtain antimicrobial and angiogenic properties while minimizing cytotoxicity by incorporating antibiotic-eluting fibrous microparticles into gelatin methacryloyl (GelMA) hydrogels. Dimethylaminotetracycline (MINO) or clindamycin (CLIN) was introduced into the polymer solution. The polymer solution was then processed into fibers using electrospinning technology, and further milled at low temperatures to obtain MINO- or CLIN-eluted fibrous microparticles. The eluted microparticles were suspended in GelMA at different concentrations to prepare the novel heterogeneous hydrogels. Compared to MINO, CLIN hydrogel enhanced the formation of capillary-like networks in endothelial cells in vitro and induced an increase in microvessel density in vivo (Dubey et al., 2023).
Existing research on wound dressings focuses more on the design of biochemical functions, combining biochemical functionality and mechanical stimuli will provide a comprehensive perspective when designing wound dressings (Hu et al., 2021). Huang et al. prepared thermos-responsive and photocrosslinked multifunctional hydrogels by grafting l-arginine onto the main chain of Methacrylated Hydroxybutyl Chitosan (HBC), aiming at inducing cellular differentiation. They applied mechanical stress through temperature-responsive deformation and relying on the synergistic effect of arginine (Arg) and deferoxamine (DFO) released from hydrogel to promote angiogenesis (Figure 4). In the rat skin wound healing assay, CD31 expression increased 3.3 times in the HBCm_Arg/DFO group than in the control group on day 5, while it increased 2.7 times in the HBCm_Arg/DFO group than in the control group on day 10, and the relative expression of HIF1-α was 10.4 times higher than that in the control group. The significant improvement in revascularization was attributed to the synergistic effect of arginine and deferoxamine (DFO) released from the hydrogel. In addition, the hydrogel’s temperature responsiveness postphotocrosslinking enables self-shrinking, leading to systolic deformation. This deformation mildly stretches the tissue, providing a stimulus for cellular growth and tissue regeneration. The contractile force of the hydrogel promotes skin wound closure (Huang et al., 2024).
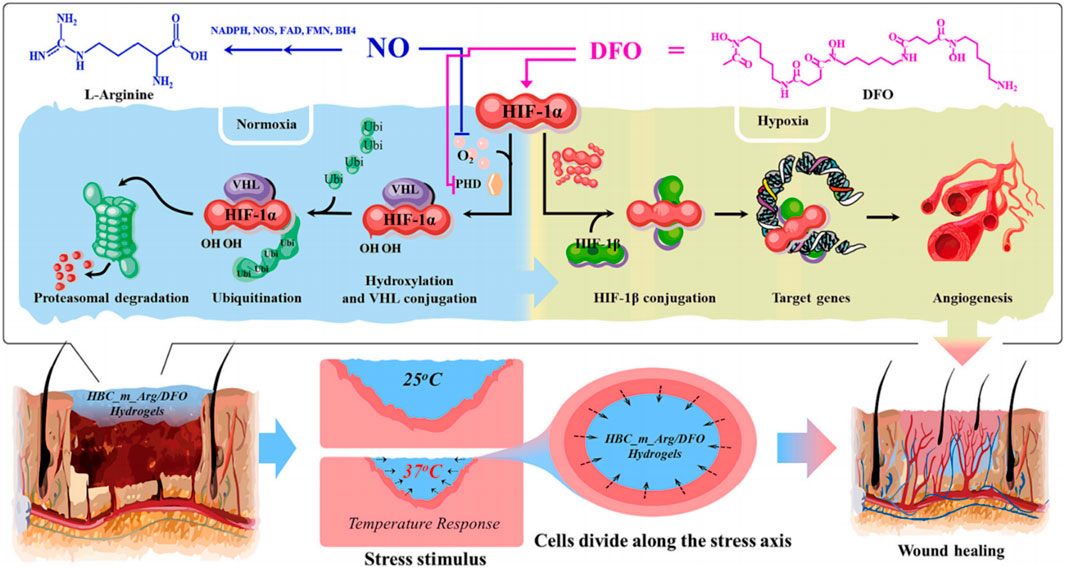
Figure 4. Schematic diagram of HBC_m_Arg/DFO hydrogel to enhance wound healing. On one hand, HBC_m_Arg/DFO hydrogel releases arginine and DFO to synergistically enhance vascular regeneration, on the other hand, temperature-responsive shrinkage provides a favorable mechanical stimulation to contract the wound (Huang et al., 2024).
5 Crosslinking strategies in natural hydrogels
In most cases, cells and factors are encapsulated into the hydrogels to develop tissues or organs, creating a complex 3D microenvironment (Sultan et al., 2021). Various polymers, including natural polymers and synthetic polymers, can form liquid monomer phase and be processed into hydrogels (Zhang and Khademhosseini, 2017).
Physical and chemical crosslinks are two basic strategies (Liu et al., 2017). Physical crosslinks typically include ionic/electrostatic interaction, hydrogen bonding, and ultrasonication mediated sol-to-gel phase transition (Zhang et al., 2011). The physical crosslinking reaction is mild and avoids the cytotoxic reactions caused by chemical crosslinking agents, but it also has the disadvantages of easy reversal of the crosslinking process and limited adjustable properties (Berger et al., 2004). Chemical crosslinked hydrogels are usually created by comprise photo-polymerization (Mironi-Harpaz et al., 2012), enzyme-induced crosslinking (Jin et al., 2010), Schiff base formation and “click” chemistry (Jia and Li, 2015). Compared with physical crosslinking, chemical crosslinking provides the hydrogel with superior mechanical properties and stability. With different chemical reactions, the time of gel process can be flexibly controlled, which is more advantageous for tissue repair with irregular defect areas or complex defect site environments (Xue et al., 2022).
In recent years, Photo-activated crosslinking has been widely used for hydrogel formation in the field of therapeutics or cytokines encapsulation (Sun et al., 2017). The advantage of this method is the rapid formation of hydrogel networks at ambient temperature under mild conditions. Additionally, the mechanical properties of the hydrogels can be tuned by controlling the crosslinking reactions (Nguyen and West, 2002). In addition, exposure to UV light can influence crosslinking density, which may in turn affect cell behavior. Lin et al. (2013) injected the GelMA solution containing human ECFCs and MSCs into mice to explore the effect of the degree of GelMA crosslinking to vascularization in vivo. Notably, they examined the application of UV light to modulate vascular morphogenesis in mice. The results showed that cell spreading was progressively diminished by increasing the UV exposure time from 15 to 45 s. In addition, the cell viability was negatively affected beyond 300s under UV exposure. The vascularization process can be directly modulated by adjusting the initial exposure time to UV light 15–45s, with constructs displaying progressively less vascular density and smaller average lumen size as the degree of GelMA crosslinking was increased. Controlling the degree of GelMA polymerization by adjusting UV light exposure time can regulate both the mechanical properties of hydrogels and the formation of vascular networks. The lack of proper scaffolding material that can both support rapid vascularization and direct the differentiation of transplanted MSCs into a specific lineage remains a major challenge. Considering the above situation, Kuo et al. prepared autologous extracellular matrix scaffolds using murine collagen-Ph hydrogels. They demonstrated the suitability of injectable, enzymatically cross-linkable collagen-Ph hydrogels for supporting the formation of 3D vascular networks from human progenitor cells, both in vitro and in vivo. They also showed that the biodegradability, swelling properties, and stiffness of collagen-Ph hydrogels, controlled by adjusting the degree of crosslinking-can be used to tune the final vascular density within the collagen-Ph hydrogel constructs (Kuo et al., 2015). Therefore, we speculate that the mode and degree of crosslinking in the hydrogel will influence its microstructure and mechanical properties, which may, in turn, impact the angiogenesis process and, consequently, affect soft tissue repair.
6 Conclusion and perspective
Vascularization plays a crucial role in soft tissue repair, directly determining and influencing the repair process and outcome. Effective vascularization in tissue-engineered scaffolds facilitates nutrient exchange and waste removal between the surrounding tissues and the scaffold interior. Due to the excellent biocompatibility and biodegradability of natural hydrogels, they are increasingly used-either individually or in combination-to construct vascular networks by creating specialized scaffold structures or serving as carriers for growth factors or stem cells. This approach has shown promising results in facilitating the repair of soft tissue defects.
However, the influence of hydrogels’ physical and chemical properties on cell activities such as spreading, migration, and differentiation remains unclear. Further research is needed to understand how these properties affect cell behavior. Additionally, the impact of reduced mechanical strength and swollen volume on vascularization and tissue formation during long-term culture periods is underexplored.
Moreover, while natural hydrogel-based vascularization strategies for soft tissue repair have shown promise in animal experiments, further in vivo studies are necessary to validate their application and support clinical translation. Although angiogenesis or vascularization within tissue-engineering scaffolds is commonly observed, the compatibility of newly formed or regenerated blood vessels with the surrounding host tissue-and the dynamic processes of scaffold degradation and local vascularized tissue repair-still require substantial research investigation.
In conclusion, the natural hydrogel-based vascularization strategies for soft tissue defect repair hold significant potential. However, ongoing research is needed to address current technical challenges and advance their clinical application and development.
Author contributions
ZX: Conceptualization, Investigation, Writing–original draft. BG: Investigation, Writing–original draft. DW: Visualization, Writing–review and editing. FY: Supervision, Writing–review and editing. YD: Supervision, Writing–review and editing.
Funding
The author(s) declare that financial support was received for the research, authorship, and/or publication of this article. This work was supported by Zhejiang Provincial Medical and Health Science and Technology Program grant (2023KY035) and Zhejiang Traditional Chinese Medicine Administration Program grant (2023ZL242).
Conflict of interest
The authors declare that the research was conducted in the absence of any commercial or financial relationships that could be construed as a potential conflict of interest.
Publisher’s note
All claims expressed in this article are solely those of the authors and do not necessarily represent those of their affiliated organizations, or those of the publisher, the editors and the reviewers. Any product that may be evaluated in this article, or claim that may be made by its manufacturer, is not guaranteed or endorsed by the publisher.
References
Abd Ellah, N. H., Abouelmagd, S. A., Abbas, A. M., Shaaban, O. M., and Hassanein, K. M. A. (2018). Dual-responsive lidocaine in situ gel reduces pain of intrauterine device insertion. Int. J. Pharm. 538, 279–286. doi:10.1016/j.ijpharm.2018.01.033
Antoine, E. E., Vlachos, P. P., and Rylander, M. N. (2014). Review of collagen I hydrogels for bioengineered tissue microenvironments: characterization of mechanics, structure, and transport. Tissue Eng. Part B Rev. 20, 683–696. doi:10.1089/ten.teb.2014.0086
Bae, H., Puranik, A. S., Gauvin, R., Edalat, F., Carrillo-Conde, B., Peppas, N. A., et al. (2012). Building vascular networks. Sci. Transl. Med. 4, 160ps23. doi:10.1126/scitranslmed.3003688
Bai, Y., Bai, L., Zhou, J., Chen, H., and Zhang, L. (2018). Sequential delivery of VEGF, FGF-2 and PDGF from the polymeric system enhance HUVECs angiogenesis in vitro and CAM angiogenesis. Cell. Immunol. 323, 19–32. doi:10.1016/j.cellimm.2017.10.008
Barrientos, S., Stojadinovic, O., Golinko, M. S., Brem, H., and Tomic-Canic, M. (2008). PERSPECTIVE ARTICLE: growth factors and cytokines in wound healing. Wound Repair Regen. 16, 585–601. doi:10.1111/j.1524-475X.2008.00410.x
Berger, J., Reist, M., Mayer, J. M., Felt, O., and Gurny, R. (2004). Structure and interactions in chitosan hydrogels formed by complexation or aggregation for biomedical applications. Eur. J. Pharm. Biopharm. 57, 35–52. doi:10.1016/S0939-6411(03)00160-7
Berthiaume, F., Maguire, T. J., and Yarmush, M. L. (2011). Tissue engineering and regenerative medicine: history, progress, and challenges. Annu. Rev. Chem. Biomol. Eng. 2, 403–430. doi:10.1146/annurev-chembioeng-061010-114257
Bonferoni, M. C., Caramella, C., Catenacci, L., Conti, B., Dorati, R., Ferrari, F., et al. (2021). Biomaterials for soft tissue repair and regeneration: a focus on Italian research in the field. Pharmaceutics 13, 1341. doi:10.3390/pharmaceutics13091341
Burdick, J. A., Mauck, R. L., and Gerecht, S. (2016). To serve and protect: hydrogels to improve stem cell-based therapies. Cell. Stem Cell. 18, 13–15. doi:10.1016/j.stem.2015.12.004
Buwalda, S. J., Vermonden, T., and Hennink, W. E. (2017). Hydrogels for therapeutic delivery: current developments and future directions. Biomacromolecules 18, 316–330. doi:10.1021/acs.biomac.6b01604
Chamberlain, G., Fox, J., Ashton, B., and Middleton, J. (2007). Concise review: mesenchymal stem cells: their phenotype, differentiation capacity, immunological features, and potential for homing. Stem Cells 25, 2739–2749. doi:10.1634/stemcells.2007-0197
Chandra, P., and Atala, A. (2019). Engineering blood vessels and vascularized tissues: technology trends and potential clinical applications. Clin. Sci. 133, 1115–1135. doi:10.1042/CS20180155
Cheng, B., Lu, S., and Fu, X. (2016). Regenerative medicine in China: demands, capacity, and regulation. Burns and Trauma 4, 24–28. doi:10.1186/s41038-016-0046-8
Cheng, Y., Li, Y., Huang, S., Yu, F., Bei, Y., Zhang, Y., et al. (2020). Hybrid freeze-dried dressings composed of epidermal growth factor and recombinant human-like collagen enhance cutaneous wound healing in rats. Front. Bioeng. Biotechnol. 8, 742. doi:10.3389/fbioe.2020.00742
Cheung, H. K., Han, T. T. Y., Marecak, D. M., Watkins, J. F., Amsden, B. G., and Flynn, L. E. (2014). Composite hydrogel scaffolds incorporating decellularized adipose tissue for soft tissue engineering with adipose-derived stem cells. Biomaterials 35, 1914–1923. doi:10.1016/j.biomaterials.2013.11.067
Chevalier, E., Chulia, D., Pouget, C., and Viana, M. (2008). Fabrication of porous substrates: a review of processes using pore forming agents in the biomaterial field. J. Pharm. Sci. 97, 1135–1154. doi:10.1002/jps.21059
Delaittre, G., Greiner, A. M., Pauloehrl, T., Bastmeyer, M., and Barner-Kowollik, C. (2015). Correction: chemical approaches to synthetic polymer surface biofunctionalization for targeted cell adhesion using small binding motifs. Soft Matter 11, 2314. doi:10.1039/C5SM90037A
Dong, K., Wang, X., Shen, Y., Wang, Y., Li, B., Cai, C., et al. (2021). Maintaining inducibility of dermal follicle cells on silk fibroin/sodium alginate scaffold for enhanced hair follicle regeneration. Biology 10, 269. doi:10.3390/biology10040269
Dubey, N., Ribeiro, J. S., Zhang, Z., Xu, J., Ferreira, J. A., Qu, L., et al. (2023). Gelatin methacryloyl hydrogel as an injectable scaffold with multi-therapeutic effects to promote antimicrobial disinfection and angiogenesis for regenerative endodontics. J. Mat. Chem. B 11, 3823–3835. doi:10.1039/D2TB02788G
Eke, G., Mangir, N., Hasirci, N., MacNeil, S., and Hasirci, V. (2017). Development of a UV crosslinked biodegradable hydrogel containing adipose derived stem cells to promote vascularization for skin wounds and tissue engineering. Biomaterials 129, 188–198. doi:10.1016/j.biomaterials.2017.03.021
Emerick, K. S., and Teknos, T. N. (2007). State-of-the-art mandible reconstruction using revascularized free-tissue transfer. Expert Rev. Anticancer Ther. 7, 1781–1788. doi:10.1586/14737140.7.12.1781
Escudero, M., Vaysse, L., Eke, G., Peyrou, M., Villarroya, F., Bonnel, S., et al. (2023). Scalable generation of pre-vascularized and functional human beige adipose organoids. Adv. Sci. 10, 2301499. doi:10.1002/advs.202301499
Fleischer, S., Tavakol, D. N., and Vunjak-Novakovic, G. (2020). From Arteries to Capillaries: Approaches to Engineering Human Vasculature. Adv Funct Materials 30, 1910811. doi:10.1002/adfm.201910811
Gajbhiye, S., and Wairkar, S. (2022). Collagen fabricated delivery systems for wound healing: a new roadmap. Biomater. Adv. 142, 213152. doi:10.1016/j.bioadv.2022.213152
Gutierrez, A. M., Frazar, E. M., X. Klaus, M. V., Paul, P., and Hilt, J. Z. (2022). Hydrogels and hydrogel nanocomposites: enhancing healthcare through human and environmental treatment. Adv. Healthc. Mater. 11, 2101820. doi:10.1002/adhm.202101820
Guvendiren, M., and Burdick, J. A. (2010). The control of stem cell morphology and differentiation by hydrogel surface wrinkles. Biomaterials 31, 6511–6518. doi:10.1016/j.biomaterials.2010.05.037
He, G., Yan, X., Miao, Z., Qian, H., Ma, Y., Xu, Y., et al. (2020). Anti-inflammatory catecholic chitosan hydrogel for rapid surgical trauma healing and subsequent prevention of tumor recurrence. Chin. Chem. Lett. 31, 1807–1811. doi:10.1016/j.cclet.2020.02.032
Hemshekhar, M., Thushara, R. M., Chandranayaka, S., Sherman, L. S., Kemparaju, K., and Girish, K. S. (2016). Emerging roles of hyaluronic acid bioscaffolds in tissue engineering and regenerative medicine. Int. J. Biol. Macromol. 86, 917–928. doi:10.1016/j.ijbiomac.2016.02.032
Hirose, S., Tabata, Y., Sone, K., Takahashi, N., Yoshino, D., and Funamoto, K. (2021). P21-activated kinase regulates oxygen-dependent migration of vascular endothelial cells in monolayers. Cell. Adhesion and Migr. 15, 272–284. doi:10.1080/19336918.2021.1978368
Hu, J., Wei, T., Zhao, H., Chen, M., Tan, Y., Ji, Z., et al. (2021). Mechanically active adhesive and immune regulative dressings for wound closure. Matter 4, 2985–3000. doi:10.1016/j.matt.2021.06.044
Huang, J., Lu, D., Wu, C., Pei, D., Guo, C., Guo, H., et al. (2024). Guanidinylated bioactive chitosan-based injectable hydrogels with pro-angiogenic and mechanical properties for accelerated wound closure. Int. J. Biol. Macromol. 258, 128943. doi:10.1016/j.ijbiomac.2023.128943
Jeon, O., Alt, D. S., Linderman, S. W., and Alsberg, E. (2013). Biochemical and physical signal gradients in hydrogels to control stem cell behavior. Adv. Mater. 25, 6366–6372. doi:10.1002/adma.201302364
Jia, Y., and Li, J. (2015). Molecular assembly of Schiff base interactions: construction and application. Chem. Rev. 115, 1597–1621. doi:10.1021/cr400559g
Jiang, C. M., Liu, J., Zhao, J. Y., Xiao, L., An, S., Gou, Y. C., et al. (2015). Effects of hypoxia on the immunomodulatory properties of human gingiva–derived mesenchymal stem cells. J. Dent. Res. 94, 69–77. doi:10.1177/0022034514557671
Jin, R., Moreira Teixeira, L. S., Dijkstra, P. J., Van Blitterswijk, C. A., Karperien, M., and Feijen, J. (2010). Enzymatically-crosslinked injectable hydrogels based on biomimetic dextran–hyaluronic acid conjugates for cartilage tissue engineering. Biomaterials 31, 3103–3113. doi:10.1016/j.biomaterials.2010.01.013
Ke, C., Wang, D., Sun, Y., Qiao, D., Ye, H., and Zeng, X. (2013). Immunostimulatory and antiangiogenic activities of low molecular weight hyaluronic acid. Food Chem. Toxicol. 58, 401–407. doi:10.1016/j.fct.2013.05.032
Ke, X., Li, M., Wang, X., Liang, J., Wang, X., Wu, S., et al. (2020). An injectable chitosan/dextran/β -glycerophosphate hydrogel as cell delivery carrier for therapy of myocardial infarction. Carbohydr. Polym. 229, 115516. doi:10.1016/j.carbpol.2019.115516
Klotz, B. J., Gawlitta, D., Rosenberg, A. J. W. P., Malda, J., and Melchels, F. P. W. (2016). Gelatin-methacryloyl hydrogels: towards biofabrication-based tissue repair. Trends Biotechnol. 34, 394–407. doi:10.1016/j.tibtech.2016.01.002
Kogan, G., Šoltés, L., Stern, R., and Gemeiner, P. (2006). Hyaluronic acid: a natural biopolymer with a broad range of biomedical and industrial applications. Biotechnol. Lett. 29, 17–25. doi:10.1007/s10529-006-9219-z
Koh, L.-D., Cheng, Y., Teng, C.-P., Khin, Y.-W., Loh, X.-J., Tee, S.-Y., et al. (2015). Structures, mechanical properties and applications of silk fibroin materials. Prog. Polym. Sci. 46, 86–110. doi:10.1016/j.progpolymsci.2015.02.001
Kuo, K.-C., Lin, R.-Z., Tien, H.-W., Wu, P.-Y., Li, Y.-C., Melero-Martin, J. M., et al. (2015). Bioengineering vascularized tissue constructs using an injectable cell-laden enzymatically crosslinked collagen hydrogel derived from dermal extracellular matrix. Acta Biomater. 27, 151–166. doi:10.1016/j.actbio.2015.09.002
Laschke, M. W., and Menger, M. D. (2012). Vascularization in tissue engineering: angiogenesis versus inosculation. Eur. Surg. Res. 48, 85–92. doi:10.1159/000336876
Li, X., Cho, B., Martin, R., Seu, M., Zhang, C., Zhou, Z., et al. (2019). Nanofiber-hydrogel composite–mediated angiogenesis for soft tissue reconstruction. Sci. Transl. Med. 11, eaau6210. doi:10.1126/scitranslmed.aau6210
Lin, H., Yin, C., Mo, A., and Hong, G. (2021). Applications of hydrogel with special physical properties in bone and cartilage regeneration. Materials 14, 235. doi:10.3390/ma14010235
Lin, R.-Z., Chen, Y.-C., Moreno-Luna, R., Khademhosseini, A., and Melero-Martin, J. M. (2013). Transdermal regulation of vascular network bioengineering using a photopolymerizable methacrylated gelatin hydrogel. Biomaterials 34, 6785–6796. doi:10.1016/j.biomaterials.2013.05.060
Liu, M., Zeng, X., Ma, C., Yi, H., Ali, Z., Mou, X., et al. (2017). Injectable hydrogels for cartilage and bone tissue engineering. Bone Res. 5, 17014. doi:10.1038/boneres.2017.14
Matai, I., Kaur, G., Seyedsalehi, A., McClinton, A., and Laurencin, C. T. (2020). Progress in 3D bioprinting technology for tissue/organ regenerative engineering. Biomaterials 226, 119536. doi:10.1016/j.biomaterials.2019.119536
Melchiorri, A. J., Nguyen, B.-N. B., and Fisher, J. P. (2014). Mesenchymal stem cells: roles and relationships in vascularization. Tissue Eng. Part B Rev. 20, 218–228. doi:10.1089/ten.teb.2013.0541
Meyer, K., Hobby, G. L., Chaffee, E., and Dawson, M. H. (1940). The hydrolysis of hyaluronic acid by bacterial enzymes. J. Exp. Med. 71, 137–146. doi:10.1084/jem.71.2.137
Mironi-Harpaz, I., Wang, D. Y., Venkatraman, S., and Seliktar, D. (2012). Photopolymerization of cell-encapsulating hydrogels: crosslinking efficiency versus cytotoxicity. Acta Biomater. 8, 1838–1848. doi:10.1016/j.actbio.2011.12.034
Müller, R., Abke, J., Schnell, E., Macionczyk, F., Gbureck, U., Mehrl, R., et al. (2005). Surface engineering of stainless steel materials by covalent collagen immobilization to improve implant biocompatibility. Biomaterials 26, 6962–6972. doi:10.1016/j.biomaterials.2005.05.013
Naleway, S. E., Porter, M. M., McKittrick, J., and Meyers, M. A. (2015). Structural design elements in biological materials: application to bioinspiration. Adv. Mater. 27, 5455–5476. doi:10.1002/adma.201502403
Nguyen, K. T., and West, J. L. (2002). Photopolymerizable hydrogels for tissue engineering applications. Biomaterials 23, 4307–4314. doi:10.1016/S0142-9612(02)00175-8
Novosel, E. C., Kleinhans, C., and Kluger, P. J. (2011). Vascularization is the key challenge in tissue engineering. Adv. Drug Deliv. Rev. 63, 300–311. doi:10.1016/j.addr.2011.03.004
Nuutila, K., Samandari, M., Endo, Y., Zhang, Y., Quint, J., Schmidt, T. A., et al. (2022). In vivo printing of growth factor-eluting adhesive scaffolds improves wound healing. Bioact. Mater. 8, 296–308. doi:10.1016/j.bioactmat.2021.06.030
Ouriemchi, E. M., and Vergnaud, J. M. (2000). Processes of drug transfer with three different polymeric systems with transdermal drug delivery. Comput. Theor. Polym. Sci. 10, 391–401. doi:10.1016/S1089-3156(00)00003-9
Paladini, F., and Pollini, M. (2022). Novel approaches and biomaterials for bone tissue engineering: a focus on silk fibroin. Materials 15, 6952. doi:10.3390/ma15196952
Park, J., Choe, G., Oh, S., and Lee, J. Y. (2020). In situ formation of proangiogenic mesenchymal stem cell spheroids in hyaluronic acid/alginate core–shell microcapsules. ACS Biomater. Sci. Eng. 6, 6938–6948. doi:10.1021/acsbiomaterials.0c01489
Patel, S., Caldwell, J., Doty, S. B., Levine, W. N., Rodeo, S., Soslowsky, L. J., et al. (2018). Integrating soft and hard tissues via interface tissue engineering. J. Orthop. Res. 36, 1069–1077. doi:10.1002/jor.23810
Pennacchio, F. A., Casale, C., Urciuolo, F., Imparato, G., Vecchione, R., and Netti, P. A. (2018). Controlling the orientation of a cell-synthesized extracellular matrix by using engineered gelatin-based building blocks. Biomater. Sci. 6, 2084–2091. doi:10.1039/C7BM01093A
Potente, M., Gerhardt, H., and Carmeliet, P. (2011). Basic and therapeutic aspects of angiogenesis. Cell. 146, 873–887. doi:10.1016/j.cell.2011.08.039
Prakash Parthiban, S., Rana, D., Jabbari, E., Benkirane-Jessel, N., and Ramalingam, M. (2017). Covalently immobilized VEGF-mimicking peptide with gelatin methacrylate enhances microvascularization of endothelial cells. Acta Biomater. 51, 330–340. doi:10.1016/j.actbio.2017.01.046
Prè, E. D., Conti, G., and Sbarbati, A. (2016). Hyaluronic acid (HA) scaffolds and multipotent stromal cells (MSCs) in regenerative medicine. Stem Cell. Rev Rep 12, 664–681. doi:10.1007/s12015-016-9684-2
Rahimi, N. (2006). VEGFR-1 and VEGFR-2: two non-identical twins with a unique physiognomy. Front. Biosci. 11, 11. doi:10.2741/1839
Rana, D., Kandar, A., Salehi-Nik, N., Inci, I., Koopman, B., and Rouwkema, J. (2022). Spatiotemporally controlled, aptamers-mediated growth factor release locally manipulates microvasculature formation within engineered tissues. Bioact. Mater. 12, 71–84. doi:10.1016/j.bioactmat.2021.10.024
Scherzer, T., Beckert, A., Langguth, H., Rummel, S., and Mehnert, R. (1997). Electron beam curing of methacrylated gelatin. I. Dependence of the degree of crosslinking on the irradiation dose. J. Appl. Polym. Sci. 63, 1303–1312. doi:10.1002/(SICI)1097-4628(19970307)63:10<1303::AID-APP9>3.0.CO;2-O
Schrementi, M. E., Ferreira, A. M., Zender, C., and DiPietro, L. A. (2008). Site-specific production of TGF-β in oral mucosal and cutaneous wounds. Wound Repair Regen. 16, 80–86. doi:10.1111/j.1524-475X.2007.00320.x
Shao, Y., and Fu, J. (2022). Engineering multiscale structural orders for high-fidelity embryoids and organoids. Cell. Stem Cell. 29, 722–743. doi:10.1016/j.stem.2022.04.003
Shen, Y., Wang, X., Wang, Y., Guo, X., Yu, K., Dong, K., et al. (2022). Bilayer silk fibroin/sodium alginate scaffold promotes vascularization and advances inflammation stage in full-thickness wound. Biofabrication 14, 035016. doi:10.1088/1758-5090/ac73b7
Shi, Q., Qian, Z., Liu, D., Sun, J., Wang, X., Liu, H., et al. (2017). GMSC-derived exosomes combined with a chitosan/silk hydrogel sponge accelerates wound healing in a diabetic rat skin defect model. Front. Physiol. 8, 904. doi:10.3389/fphys.2017.00904
Shi, X., Wang, X., Shen, W., and Yue, W. (2023). Biocompatibility of silk methacrylate/gelatin-methacryloyl composite hydrogel and its feasibility as a vascular tissue engineering scaffold. Biochem. Biophysical Res. Commun. 650, 62–72. doi:10.1016/j.bbrc.2023.01.097
Shoulders, M. D., and Raines, R. T. (2009). Collagen structure and stability. Annu. Rev. Biochem. 78, 929–958. doi:10.1146/annurev.biochem.77.032207.120833
Singh, S., Choudhury, D., Yu, F., Mironov, V., and Naing, M. W. (2020). In situ bioprinting – bioprinting from benchside to bedside? Acta Biomater. 101, 14–25. doi:10.1016/j.actbio.2019.08.045
Soares, P. I. P., Sousa, A. I., Silva, J. C., Ferreira, I. M. M., Novo, C. M. M., and Borges, J. P. (2016). Chitosan-based nanoparticles as drug delivery systems for doxorubicin: optimization and modelling. Carbohydr. Polym. 147, 304–312. doi:10.1016/j.carbpol.2016.03.028
Sultan, Md. T., Choi, B. Y., Ajiteru, O., Hong, D. K., Lee, S. M., Kim, H.-J., et al. (2021). Reinforced-hydrogel encapsulated hMSCs towards brain injury treatment by trans-septal approach. Biomaterials 266, 120413. doi:10.1016/j.biomaterials.2020.120413
Sun, F., Xiao, D., Su, H., Chen, Z., Wang, B., Feng, X., et al. (2023). Highly stretchable porous regenerated silk fibroin film for enhanced wound healing. J. Mat. Chem. B 11, 1486–1494. doi:10.1039/D2TB01896A
Sun, X., Lang, Q., Zhang, H., Cheng, L., Zhang, Y., Pan, G., et al. (2017). Electrospun photocrosslinkable hydrogel fibrous scaffolds for rapid in vivo vascularized skin flap regeneration. Adv. Funct. Mater. 27, 1604617. doi:10.1002/adfm.201604617
Tateishi, U. (2005). Vascular endothelial growth factor–related angiogenesis. Radiology 235, 1084–1085. doi:10.1148/radiol.2353042072
Ul-Islam, M., Subhan, F., Islam, S. U., Khan, S., Shah, N., Manan, S., et al. (2019). Development of three-dimensional bacterial cellulose/chitosan scaffolds: analysis of cell-scaffold interaction for potential application in the diagnosis of ovarian cancer. Int. J. Biol. Macromol. 137, 1050–1059. doi:10.1016/j.ijbiomac.2019.07.050
Van Den Bulcke, A. I., Bogdanov, B., De Rooze, N., Schacht, E. H., Cornelissen, M., and Berghmans, H. (2000). Structural and rheological properties of methacrylamide modified gelatin hydrogels. Biomacromolecules 1, 31–38. doi:10.1021/bm990017d
Vedadghavami, A., Minooei, F., Mohammadi, M. H., Khetani, S., Rezaei Kolahchi, A., Mashayekhan, S., et al. (2017). Manufacturing of hydrogel biomaterials with controlled mechanical properties for tissue engineering applications. Acta Biomater. 62, 42–63. doi:10.1016/j.actbio.2017.07.028
Vepari, C., and Kaplan, D. L. (2007). Silk as a biomaterial. Prog. Polym. Sci. 32, 991–1007. doi:10.1016/j.progpolymsci.2007.05.013
Voigt, J., and Driver, V. R. (2012). Hyaluronic acid derivatives and their healing effect on burns, epithelial surgical wounds, and chronic wounds: a systematic review and meta-analysis of randomized controlled trials. Wound Repair Regen. 20, 317–331. doi:10.1111/j.1524-475X.2012.00777.x
Wakitani, S., Goto, T., Young, R. G., Mansour, J. M., Goldberg, V. M., and Caplan, A. I. (1998). Repair of large full-thickness articular cartilage defects with allograft articular chondrocytes embedded in a collagen gel. Tissue Eng. 4, 429–444. doi:10.1089/ten.1998.4.429
Wang, P., Sun, Y., Shi, X., Shen, H., Ning, H., and Liu, H. (2021). Bioscaffolds embedded with regulatory modules for cell growth and tissue formation: a review. Bioact. Mater. 6, 1283–1307. doi:10.1016/j.bioactmat.2020.10.014
Wegst, U. G. K., Bai, H., Saiz, E., Tomsia, A. P., and Ritchie, R. O. (2015). Bioinspired structural materials. Nat. Mater 14, 23–36. doi:10.1038/nmat4089
Xiao, C., You, R., Fan, Y., and Zhang, Y. (2016). Tunable functional hydrogels formed from a versatile water-soluble chitosan. Int. J. Biol. Macromol. 85, 386–390. doi:10.1016/j.ijbiomac.2016.01.006
Xu, J., Ma, L., Liu, Y., Xu, F., Nie, J., and Ma, G. (2012). Design and characterization of antitumor drug paclitaxel-loaded chitosan nanoparticles by W/O emulsions. Int. J. Biol. Macromol. 50, 438–443. doi:10.1016/j.ijbiomac.2011.12.034
Xue, X., Hu, Y., Wang, S., Chen, X., Jiang, Y., and Su, J. (2022). Fabrication of physical and chemical crosslinked hydrogels for bone tissue engineering. Bioact. Mater. 12, 327–339. doi:10.1016/j.bioactmat.2021.10.029
Yadav, S., Khan, J., and Yadav, A. (2024). Applications of scaffolds in tissue engineering: current utilization andFuture prospective. CGT 24, 94–109. doi:10.2174/0115665232262167231012102837
Yang, M.-C., Wang, S.-S., Chou, N.-K., Chi, N.-H., Huang, Y.-Y., Chang, Y.-L., et al. (2009). The cardiomyogenic differentiation of rat mesenchymal stem cells on silk fibroin–polysaccharide cardiac patches in vitro. Biomaterials 30, 3757–3765. doi:10.1016/j.biomaterials.2009.03.057
Yang, W., Xu, H., Lan, Y., Zhu, Q., Liu, Y., Huang, S., et al. (2019a). Preparation and characterisation of a novel silk fibroin/hyaluronic acid/sodium alginate scaffold for skin repair. Int. J. Biol. Macromol. 130, 58–67. doi:10.1016/j.ijbiomac.2019.02.120
Yang, X., Yang, D., Zhu, X., Nie, J., and Ma, G. (2019b). Electrospun and photocrosslinked gelatin/dextran–maleic anhydride composite fibers for tissue engineering. Eur. Polym. J. 113, 142–147. doi:10.1016/j.eurpolymj.2019.01.059
Yee, D., Hanjaya-Putra, D., Bose, V., Luong, E., and Gerecht, S. (2011). Hyaluronic acid hydrogels support cord-like structures from endothelial colony-forming cells. Tissue Eng. Part A 17, 1351–1361. doi:10.1089/ten.tea.2010.0481
Yue, S., He, H., Li, B., and Hou, T. (2020). Hydrogel as a biomaterial for bone tissue engineering: a review. Nanomaterials 10, 1511. doi:10.3390/nano10081511
Zeltz, C., and Gullberg, D. (2016). The integrin–collagen connection – a glue for tissue repair? J. Cell. Sci. 129, 653–664. doi:10.1242/jcs.180992
Zhang, K., Shi, X., Lin, X., Yao, C., Shen, L., and Feng, Y. (2015). Poloxamer-based in situ hydrogels for controlled delivery of hydrophilic macromolecules after intramuscular injection in rats. Drug Deliv. 22, 375–382. doi:10.3109/10717544.2014.891272
Zhang, Q., Qiao, Y., Li, C., Lin, J., Han, H., Li, X., et al. (2021). Chitosan/gelatin-tannic acid decorated porous tape suture with multifunctionality for tendon healing. Carbohydr. Polym. 268, 118246. doi:10.1016/j.carbpol.2021.118246
Zhang, W., Wang, X., Wang, S., Zhao, J., Xu, L., Zhu, C., et al. (2011). The use of injectable sonication-induced silk hydrogel for VEGF165 and BMP-2 delivery for elevation of the maxillary sinus floor. Biomaterials 32, 9415–9424. doi:10.1016/j.biomaterials.2011.08.047
Zhang, Y., Xu, Y., and Gao, J. (2023). The engineering and application of extracellular matrix hydrogels: a review. Biomater. Sci. 11, 3784–3799. doi:10.1039/D3BM00183K
Zhang, Y. S., and Khademhosseini, A. (2017). Advances in engineering hydrogels. Science 356, eaaf3627. doi:10.1126/science.aaf3627
Zhao, C., Tan, A., Pastorin, G., and Ho, H. K. (2013). Nanomaterial scaffolds for stem cell proliferation and differentiation in tissue engineering. Biotechnol. Adv. 31, 654–668. doi:10.1016/j.biotechadv.2012.08.001
Zheng, M., Wang, X., Chen, Y., Yue, O., Bai, Z., Cui, B., et al. (2023). A review of recent progress on collagen-based biomaterials. Adv. Healthc. Mater. 12, 2202042. doi:10.1002/adhm.202202042
Zhu, Y., Hideyoshi, S., Jiang, H., Matsumura, Y., Dziki, J. L., LoPresti, S. T., et al. (2018a). Injectable, porous, biohybrid hydrogels incorporating decellularized tissue components for soft tissue applications. Acta Biomater. 73, 112–126. doi:10.1016/j.actbio.2018.04.003
Keywords: natural materials, hydrogel, vascularization, soft tissue, medical applications
Citation: Xia Z, Guo B, Wu D, Yang F and Ding Y (2024) Advances of natural hydrogel-based vascularization strategies for soft tissue repair. Front. Mater. 11:1446035. doi: 10.3389/fmats.2024.1446035
Received: 08 June 2024; Accepted: 01 October 2024;
Published: 15 October 2024.
Edited by:
Elisa Mazzoni, University of Ferrara, ItalyReviewed by:
Kun Liang, Agency for Science, Technology and Research (A*STAR), SingaporeDejian Li, Fudan University Pudong Medical Center, China
Copyright © 2024 Xia, Guo, Wu, Yang and Ding. This is an open-access article distributed under the terms of the Creative Commons Attribution License (CC BY). The use, distribution or reproduction in other forums is permitted, provided the original author(s) and the copyright owner(s) are credited and that the original publication in this journal is cited, in accordance with accepted academic practice. No use, distribution or reproduction is permitted which does not comply with these terms.
*Correspondence: Fan Yang, eWFuZ2ZhbkBobWMuZWR1LmNu; Yude Ding, ZGluZ3l1ZGVAaG1jLmVkdS5jbg==