- 1Department of Orthopedic, Nantong Traditional Chinese Medicine Hospital, Nantong, Jiangsu, China
- 2Department of Anesthesiology, Affiliated Hospital of Nantong University, Nantong, Jiangsu, China
- 3Department of Traumatology and Orthopedics, Wuxi Affiliated Hospital of Nanjing University of Chinese Medicine, Wuxi, Jiangsu, China
Introduction: Optimizing the physical microstructure of nerve grafts and enhancing their biological functions to create a microenvironment that favors the regeneration of damaged nerves can significantly improve the recovery of damaged nerve function. Fibers constructed using electrospinning technology can effectively replicate the 3D skeleton of the extracellular matrix (ECM). The impact of the porous characteristics of the fiber surface on cellular growth behavior has attracted considerable attention from researchers. However, there are few studies that have explored the synergistic influence of fiber surface nanotopology and silk fibroin (SF) on peripheral nerve cell growth patterns.
Methods: This study presents a polylactic acid (PLA)/silk fibroin (SF) composite fiber membrane featuring a nanopore structure on its surface. The following tests were used to characterize the performance of the fiber membrane: scanning electron microscopy (SEM), Fourier transform infrared spectroscopy (FTIR), tensile testing, water contact angle (WCA) measurements, degradation pH assessment, and topological structure stability tests. In addition, we also explored the biocompatibility, cytotoxicity, and the influence of the PLA@SF aligned porous composite fiber membrane on the growth behavior of peripheral nerve cells.
Results: Results from physical and chemical tests indicate that the PLA@SF composite fiber membrane exhibits an appropriate degradation rate, favorable mechanical and hydrophilic properties, and excellent topological structure stability. MTT assays demonstrate that the PLA@SF composite fiber membrane possesses good biological safety.
Discussion: The synergistic effect of the porous nanostructures and SF improved growth behavior of RSC96 cells: proliferation and migration.
1 Introduction
It is well known that peripheral nerves possess a certain degree of self-repairing ability after damage, which contrasts with the situation following central nerve damage, albeit primarily in cases of mild nerve damage. For severe nerve defects, autologous nerve grafting has remained the best clinical option; nevertheless, this approach is fraught with issues such as limited donor availability, The number of donor nerves is limited, there are nerve size mismatches, and neuroma formation occurs (Yi et al., 2019; Beris et al., 2019; Yan et al., 2020). Furthermore, long or thick nerve trunk defects necessitate a substantial amount of graft material, significantly elevating the risk of surgical trauma and complications. In contrast, artificial nerve grafts offer advantages such as adjustable structural dimensions and functional specifications, abundant sources of raw materials, and the capacity to adapt to diverse clinical applications. Consequently, they have emerged as one of the preferred treatment modalities for replacing autologous nerves in nerve defect repair. Traditional nerve grafts often exhibit suboptimal efficacy due to the absence of biomimetic nanostructures and modifications incorporating biochemical and physical cues. As a result, they struggle to facilitate the rational and straightforward remodeling of imbalanced growth microenvironments. Throughout the process of nerve repair post-injury, creating a suitable microenvironment is essential for facilitating nerve regeneration and restoring functionality (Qian et al., 2021). Nervous tissue has a delicate structure and complex functions, characterized by distinct anisotropy, heterogeneity, and directionality in fiber arrangement (Li et al., 2021). After nerve injury, in the regeneration stage, the orderly arrangement of nerve fibers plays a crucial role in correctly guiding axons and promoting directional cell growth. The directional microstructure is a key factor influencing the growth behavior of nerve cells. Products that are acellular allogeneic nerve grafts, which construct neural micro-structures and retain active ingredients, have been designed to repair damaged peripheral nerves, exemplified by Avance® (Suryavanshi et al., 2020). While these products can yield positive biological effects, they are still subject to risks including immune rejection, limited sources of extracellular matrix (ECM), and potential pathogen transmission. Mimicking the spatial structure of the ECM is a widely recognized method in tissue engineering. In addition, the ECM influences cell growth behavior through various topographical cues and biochemical signals, and is essential for cell survival, activity, and regulation within the external environment (Liang et al., 2007; Chaudhuri et al., 2020). Guan et al. reported that ECM-modified aligned PCL/SF electrospun fiber membranes exhibit effects similar to those of autologous nerves in bridging peripheral nerve defects in rats (Guan et al., 2023). To replicate ECM’s unique 3D structure, researchers have explored numerous methods. Among these, scaffolds prepared based on electrostatic spinning technology have attracted much focus due to their spatial structure approaching the ECM (Lee et al., 2022; Kong et al., 2022; Dai et al., 2024). The nanofiber scaffold effectively simulates the inherent structure of the extracellular matrix of fibers, providing an ideal environment for cell growth and tissue regeneration (Liu et al., 2024a). In principle, cell growth behavior can be modulated through bio-chemistry, microtopography, and electrical stimulation (Yamada et al., 2007; Engler et al., 2006; Entekhabi et al., 2021). Studies have shown that the dimensions, micromorphology, and alignment within electrospun fibers can affect cell proliferation, differentiation, maturation, and the expression of relevant genes (Omidinia-Anarkoli et al., 2019; Xuan et al., 2021; Xie et al., 2009; Tao et al., 2019). Researchers have found that the micron- and nanometer-level topology of individual fibers can mechanically adjust the growth morphology of fibroblasts (Omidinia-Anarkoli et al., 2019). The aligned nanofibers synergistically increase the tendency to differentiate toward neural lineages and also adjust the direction of neural axon growth (Xie et al., 2009). Therefore, the microenvironment established based on physical microstructural cues serves as an effective strategy to regulates the process of nerve cell growth and differentiation. Additionally, researchers have discovered that fiber nanostructures play a significant function in regulating cell proliferation, differentiation, migration, and cell-fiber interactions (Jing et al., 2021; Lanno et al., 2020; Hu et al., 2016; Lu and Xia, 2013). For instance, neural stem cells are more likely to proliferate and differentiate when they adhere to large nanopore, while smaller nanopores facilitate their migration; furthermore, varying arrangements of fibers can synergistically enhance cellular responses (Xuan et al., 2021). Nevertheless, the materials predominantly employed to construct fiber surface microstructures are synthetic polymers: polylactic acid (PLA), polycaprolactone (PCL), polypropylene (PP), polystyrene (PS) ect. The mechanical attributes of these synthetic polymers are outstanding, ease of processing, and non-toxicity, making them widely utilized in the biomedical field (Lee et al., 2021; Li et al., 2023). Nevertheless, their biocompatibility and degradation rates are generally inferior to those of natural biological materials.
As a biodegradable chemically synthesized polymer material, polylactic acid has been used in clinical practice for many years, and absorbable sutures represented by PLA have brought great benefits to surgical patients. In 1974, Johnson & Johnson launched the classic product Vicryl®. This product is composed of polylactic acid and is one of the earliest synthetic absorbable sutures (Chu et al., 2013). In theory, PLA, after microbial decomposition in vitro, produces water and carbon dioxide as the final products, which are green, safe, and have no toxic effects on the human body (Luo et al., 2019). It is one of the few polymer materials approved by the US FDA for use in the medical field (Khouri et al., 2024). PLA has excellent mechanical properties and great potential for application in the field of biomedicine (Santoro et al., 2016). However, PLA itself has poor hydrophilicity and slow degradation rate, which limits its further development and use. Therefore, it is necessary to modify PLA or optimize its performance by combining different materials during actual use. Chen et al. (2021) developed an intelligent photo thermal responsive PLA nanofiber membrane scaffold. After modifying the scaffold, its hydrophilicity and bone conductivity were improved. In vitro studies showed that the modified PLA based fiber membrane scaffold is beneficial for cell adhesion and growth, has good biocompatibility, and has improved biological activity to induce the formation of apatite. Ehterami et al. (2021) developed a high porosity scaffold using a PLA/CS mixture through liquid-liquid phase separation technology. In vitro cell experiments showed that the scaffold provides powerful conditions for the adhesion, vitality, and proliferation of nerve cells, demonstrating its enormous potential for application in the field of neural tissue engineering.
Silk fibroin (SF) is considered ideal materical for repairing various tissues including bones, skin, blood vessels, and nerves, due to its good biocompatibility, suitable immunogenicity, minimal bacterial adhesion and adjustable biodegradability and mechanical properties (Yao et al., 2022). It is considered one of the natural polymers with high potential and application value in biomaterials (Min et al., 2004; Liu et al., 2022; Han et al., 2019). A promising strategy is to reprocess silk fibroin and reconstruct it into functional regenerated silk fibroin (RSF) materials with controllable format and structure. This strategy can endow them with excellent processability and modifiability, thus meeting the needs of different scenarios (Yao et al., 2022). The addition of SF into synthetic polymers to create functionalized biomaterials is a straightforward and effective approach. We hypothesize that integrating SF into electrospun fiber membranes with nanopore structures on their surfaces may positively influence nerve regeneration. However, there is a scarcity of reports addressing the incorporation of SF into electrospun fiber membranes with nanopore structures and their subsequent effects on properties, particularly concerning nerve regeneration.
Characterizing the electrospun PLA-SF porous nanocomposite fiber membrane and assessing its possible applications in nerve regeneration constitute the main purpose of this study. A porous nano-PLA@SF composite fiber membrane was fabricated under controlled temperature and humidity conditions, and a systematic investigation was conducted on the morphology, mechanical properties, degradability, wettability, and topological structural stability of the fibers. Additionally, the cytotoxicity and hemocompatibility of the PLA@SF composite fiber membrane were evaluated. Furthermore, the impact of incorporating a nanoporous structure and silk fibroin (SF) on the growth behavior of RSC96 cells (SCS) was examined.
2 Materials and methods
2.1 Fabrication of electrospun fibrous membranes
At room temperature, PLA particles (Mw = 60,000, Yuanye, Shanghai, China) were oven-dried at 65°C for at least 24 h and subsequently dissolved in a composite solvent of CH2Cl2/HFIP (5:1, w/w) with continuous stirring for 6 h to achieve a homogeneous mixture. The concentration of the pure PLA smooth fiber membrane group was set at 12 wt%, while the concentrations for the porous PLA, PLA@SF-P3, and PLA@SF-P5 sample groups were maintained at 8 wt%. The silk fibroin (SF) addition amounts were 0, 3, and 5 wt% relative to the weight of PLA. A voltage of 13 kV was applied during the electrospinning process. With a solution injection flow rate of 0.75 mL/h, and lasted for 5 min per sample. Collection of randomly oriented fibers using a conductive flat plate collector, while aligned fibers were collected using the same setup but with a fixed-speed drum collector rotating at 2,500 rpm. The electrospinning distance was maintained at 14 cm. Smooth non-porous aligned/disordered PLA fiber membranes were produced under conditions of 30% relative humidity and 25°C, whereas porous aligned PLA and PLA@SF fiber membranes were fabricated at 50% relative humidity and 45°C. After electrospinning, the PLA@SF fiber membrane was soaked in absolute ethanol overnight to denature the silk fibroin, followed by washing and drying for subsequent use. The samples include PLA-SR, PLA-SA, and PLA-PA, whose fibers correspond to random, aligned, and porous aligned distributions, respectively; and PLA@SF-Px composite membranes featuring porous aligned fibers (PLA@SF-Px, x = 3 or 5, x representing the proportion of SF).
2.2 Analysis of the properties of electrospun fiber membranes
The Gemini SEM300 (SEISS, Germany) was utilized to study the morphology of the sur-face of various fiber membrane samples. Using software (ImageJ) to analyze the performance of each group of fiber membranes, including fiber pore size, single fiber diameter, and porosity. A total of 150 fibers from different groups were randomly measured to obtain fiber diameter and diameter distribution. For each set of fibers membranes, Three distinct fibers were arbitrarily chosen and subjected to analysis to ascertain the pore dimensions and the porosity of their surfaces. The infrared spectra of both the electrospun fiber membrane and the pure silk fibroin (SF) were documented utilizing a Tensor 27 spectrometer (Bruker, Germany) at ambient temperature. A uniaxial mechanical testing machine (MTS Insight 30, Washington, United States) was used to assess the tensile properties of the films. Taken the average of four tests as the mechanical tensile data for each group of fibers. The test specimens were rectangular, measuring 0.5 cm × 2 cm. The tensile testing parameters are set to apply a constant displacement rate of 10 mm/min until the fiber sample is completely fractured. The static water contact angle (WCA) was measured using a contact angle meter (DSA20, Klaus, Germany). The tangent angle of different fiber membranes was recorded after contact with a droplet for 2 s, employing the dropping method. Take the average value after 4 times of measurement for each sample. The degradation pH of each group of fiber membranes was measured by using a pH meter (Ohaus, ST2100, China) at different time points. Each group of fiber membranes was immersed in Phosphate-Buffered Saline (PBS) to assess the orientation angle of the fibers at different time intervals, thereby evaluating the stability of the fiber membrane topology. To visualize the distribution of silk fibroin (SF) within the fibers, SF was fluorescently labeled with FITC. Specifically, FITC was mixed with PLA, PLA@SF-P3, and PLA@SF-P5 solutions following the manufacturer’s instructions (MCE, Shanghai, China). Following preparation, the mixtures were incubated in the dark at ambient temperature for 8 h before electrospinning. Subsequently, A Zeiss Axio Scope A1 (Carl Zeiss, Germany) was utilized to capture images of each fiber membrane group.
2.3 Cell viability assay
The RSC96 (rat Schwann cells, Procell, Wuhan, China) cell line was the main cell model used in this study. The MTT assay for cell viability was utilized to evaluate the impact of PLA, PLA@SF-P3, and PLA@SF-P5 fiber membranes on the cytotoxicity of RSC96 cells. Specifically, the aforementioned fiber membrane groups were fully immersed in Dulbecco’s Modified Eagle’s Medium (DMEM) (Sigma, United States) with a 6 cm2/mL ratio and incubated at 37°C for 24 h to obtain the sample medium via immersion liquor extraction. Following the revival and stable passage of the RSC96 cell line three times, The cells were seeded in complete culture medium (DMEM, FBS, penicillin and streptomycin, 90:10:1, V/V/V) for cell experiments. The MTT assay was performed following the protocol provided by the reagent kit (Beyotime, Shanghai, China). Specifically, first add 10 μL of MTT solution to each well, then add 100 μL of formazan, with each step lasting for 4 h (5 × 103 cells/mL/well, 96 well medium). The optical density (OD) was taken at 570 nm with a microplate reader (Bio-Tek, Eon, United States). Each experimental setup was repeated three times to ensure reliability. For cytotoxicity testing, use a microplate reader to measure the OD value at 570 nm (OD570) for calculating Cell viability.
Here ODe: experimental sample group, ODc: control group.
2.4 Hemolysis assay
Material hemolysis tests were conducted using rabbit anticoagulated whole blood (YaJiBio, Shanghai, China). The anticoagulated rabbit blood was mixed with 0.9% physiological saline (5:4, V/V). A suspension of each group of fiber membranes (PLA-PA, PLA@SF-P3, PLA@SF-P5) was prepared using 0.9% normal saline (5 mg/mL). At the same time, distilled water and physiological saline were set as positive control group and negative control group, respectively. Samples were suspended in 5 mL volumes and warmed to 37°C for a duration of 30 min. Following this, 100 μL of rabbit blood that had been anticoagulated and diluted was introduced into each sample. Subsequently, after incubating at 37°C for 1 h, centrifuge at 3,000 rpm for 5 min Lastly, take 100 μL of supernatant from each sample group and measure its absorbance at a wavelength of 545 nm. Hemolysis percentage is determined by the subsequent formula:
Here ODe: experimental sample group, ODn: negative control group, and ODp: positive control group.
2.5 Cell proliferation assay
The EDU cell proliferation kit (Elabscience, Wuhan, China) was utilized to assess the pro-liferation performance of RSC96 cells on each group of fibrous membranes by incorporating EdU into DNA. Specifically, each group of electrospun fiber membranes was cut into disc shapes (11 mm in diameter) to fit the 48-well plate and then attached to the cell spreading sheet before being placed in the 48-well plate and fixed in position. Subsequently, the well plate was irradiated under UV light for 45 min, immersed in 75% ethanol for an additional 45 min, and finally rinsed several times with PBS before being dried for use. The cell culture procedure referred to Section 2.3, with the exception of the cell growth density, which was 4 × 104 cells/mL/well. After culturing the cells for 24 h, 300 μL of EdU solution was added to each well to continue the culture for an additional 2 h. The cells were then fixed with 4% paraformaldehyde for the next half hour and processed according to the kit instructions. The cells were subsequently imaged using a Zeiss Axio Scope A1 (Carl Zeiss, Germany). All experiments were conducted in triplicate.
2.6 Fluorescence staining of SCs
The 24-well plates containing various groups of electrospun fiber membranes were sterilized, washed, and dried prior to use. Cell culture and fixation steps are described in Section 2.3 and then diluting Triton-X 100 to a concentration of 0.5% using PBS, and blocked with 5% bovine serum albumin (BSA, Sigma) in PBS for 30 min. Cells were then incubated with the primary antibody S100-β (1:300, ab52642, Abcam) at 4°C overnight. On the following day, the cells were stained with the secondary anti-body Alexa Fluor 488-conjugated anti-mouse antibody (1:400, ab150113, Abcam) for 2 h, and finally mounted using DAPI-containing mounting buffer (Beyotime, Shanghai, China). Images were captured using a Zeiss Axio Scope A1.
2.7 SCs morphology
After a 24-hour incubation, the aforementioned samples were fixed and rinsed. The samples were then fixed with 1% osmium tetroxide for 2 h under conditions of darkness. Protected from light, the samples were then subjected to a series of ethanol concentration gradients for dehydration, which included 20%, 40%, 60%, 80%, 90%, 95%, and 100% solutions, maintaining each step of the process for 5 min. Lastly, the samples were dried at room temperature and imaged using scanning electron microscopy (SEM) after platinum coating.
2.8 Cell migration
Cell migration experiment was performed using a sample immersion solution. The immersion process, cell culture, and inoculation steps are described in Section 2.3. Awaiting cell confluence, the cell monolayer was scraped with a pipette tip to create straight scratches. After washing with PBS three times, blank control group added complete medium, while the respective material extracts were added to the experimental group. Details regarding the preparation of the material extract, as specified in Section 2.3, involved immersing the fiber membrane in DMEM at a ratio of 6 cm2/mL and incubating it at 37°C for 24 h. Images were taken at 0 h, 12 h, and 24 h to observe cell migration. Cells were imaged using a Nikon inverted microscope (TS2, To-kyo, Japan). All experiments were conducted three times.
2.9 Statistical analysis
All data were presented as means ± SD or means ± SEM and were analyzed using one-way ANOVA, t-test, by Graphpad Prism 10 (GraphPad Software, United States) statistical analysis software. A P-value of less than 0.05 between two groups was considered to indicate a statistically significant difference (*p < 0.05, **p < 0.01, ***p < 0.001, ****p < 0.0001).
3 Results
3.1 Fibrous membranes characterizations
SEM was employed to image each group of fiber membranes. Figure 1 presents the SEM images depicting the morphology of PLA and PLA@SF composite fiber membranes. As observed, electrospun fiber membranes with both random and directional fiber distributions were successfully produced by utilizing different collectors. Furthermore, through the optimization of preparation conditions and specific electrospinning parameters, a porous nanostructure was effectively constructed on the surface of the aligned PLA fiber membrane. The emergence of these porous nanostructures is attributed to the meticulous adjustment of relative environmental humidity and solvent composition, which modulates the vapor pressure of the solvent and water, ultimately influencing the location of hole formation on the fiber surface or within the fiber (Zhang et al., 2023). The generation of the fiber hole structure in this study is a typical outcome of gas phase-induced phase separation. When the electrospinning solution is sprayed from the nozzle tip, the composite solvent that accumulates on the surface of the PLA jet rapidly evaporates, leading to localized cooling and the formation of water vapor. Condensation is likely to occur at the jet/air interface. Consequently, in a relatively high humidity environment, a significant number of pores can be effectively formed on the surface of the nanofibers (Lu and Xia, 2013). Given that PLA is a hydrophobic polymer, condensed water tends to accumulate on the fiber surface, which creates a porous structure. The composition of the electrospinning solution solvent, along with the temperature and humidity during the electrospinning process, are critical factors influencing the generation of fiber hole structures. As a result, this influences the size of the pores and the extent of porosity in the fiber. The diameter distribution of each group of electrospun fiber membranes is illustrated in Figure 1 (A-1, B-1, C-1, D-1, E-1). PLA-SR: 1.1 ± 0.15 μm, PLA-SA: 1.14 ± 0.15 μm, PLA-PA: 1.06 ± 0.13 μm, PLA@SF-P3: 1.05 ± 0.12 μm, and PLA@SF-P5: 1.02 ± 0.11 μm (average diameters). This study demonstrates that, under identical preparation processes, the in-corporation of SF leads to a decrease in the diameter of porous PLA fibers, corroborating findings by Mohammadzadehmoghadam and Dong (2019). The observed reduction in fiber diameter due to the introduction of SF can be attributed to the following factors: (1) the inherent characteristics of the SF fiber itself, typically forms electrospun nanofibers ranging from 250 to 750 nm (Jin et al., 2024; Yang et al., 2024); and (2) The addition of SF enhances the viscosity of the electrospinning solution, which can have significant impacts on the electrospinning properties and the dimensions of the fiber filaments (Mohammadzadehmoghadam and Dong, 2019).
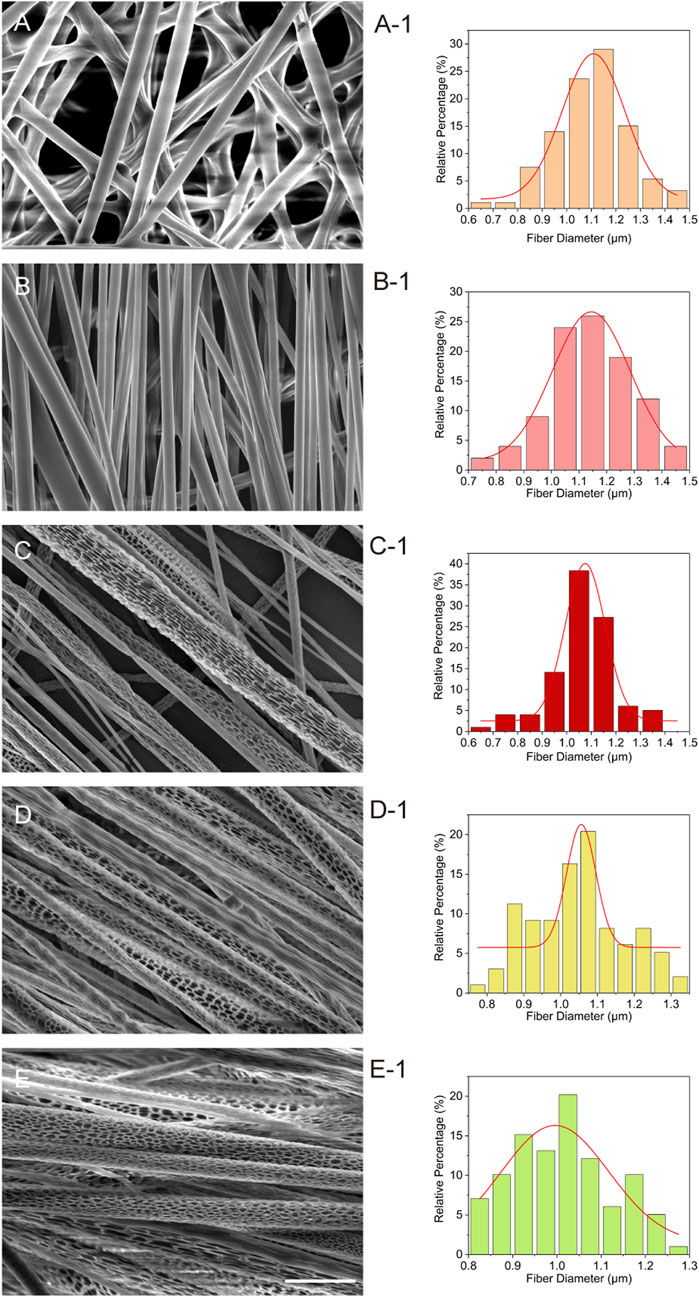
Figure 1. SEM images of electrospun membranes. (A): PLA-SR, (B) PLA-SA, (C) PLA-PA, (D) PLA@SF-P3 and (E) PLA@SF-P5, scale bar: 2 μm). The diameter distribution of the various electrospun fibers (A-1 corresponds to A, and so on).
As shown in Figure 2C, the diameters of the prepared fiber components—PLA-SR, PLA-SA, PLA-PA, PLA@SF-P3, and PLA@SF-P5—are consistently controlled at approxi-mately 1 μm. Additionally, the p-value for all pairwise comparisons exceeded 0.05. Thereby mitigating the potential impact of diameter variability across the fiber groups. A substantial body of research indicates that fiber diameter significantly influences various aspects of cell growth behavior, including cell migration (Ahmed et al., 2018), proliferation (Gao et al., 2024), morphology (Herrero-Herrero et al., 2021), differentiation (Christopherson et al., 2009), and the expression of related genes and proteins (Gao et al., 2024). Moreover, the growth behavior of cells is also regulated by features such as fiber orientation and surface nano patterns (Liu et al., 2024b). Gu et al. (2023) designed a single - layer, parallel - aligned polycaprolactone fiber model with minimal interference. They studied how fiber diameter affects BMSCs behavior. Results show that “interference” cell adhesion between adjacent fibers and fiber diameter are key factors in causing different cell behaviors. In a sparse fiber arrangement, small - diameter fibers (1 μm) limit cell proliferation and osteogenesis, while medium - diameter (8 μm) fibers have the best osteogenic effect. But in a dense fiber arrangement, small - diameter fibers show the highest cell proliferation and osteogenic capacity. This objectively shows that fiber diameter greatly affects stem cell growth. Furthermore, when the difference in fiber diameters reaches a notable fold change (Wang et al., 2010) (759 ± 179 nm vs. 293 ± 65 nm) or an order of magnitude change (Gao et al., 2024) (e.g., 700 nm vs. 3 μm), the effects on cell growth behavior are pronounced. In our study, however, we have meticulously controlled the fiber diameter, ensuring that the diameters of each group of fibers are of the same order of magnitude. Consequently, the potential influence of fiber diameter variations within this study is likely to be negligible.
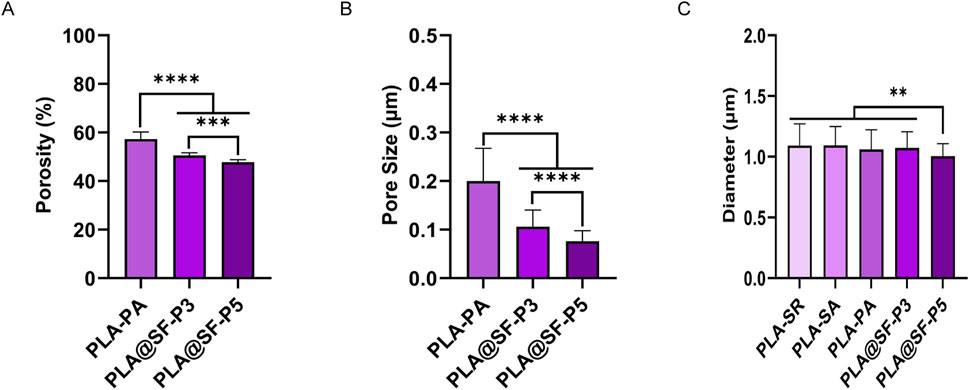
Figure 2. Various porous fiber membranes. (A) Porosity (B) average pore dimensions of various porous fibers (C) Diameter values: mean ± SEM (one-way ANOVA, n = 6).
Figure 1 shows that a multitude of microporous structures emerge on the surfaces of PLA and PLA@SF composite fibers due to high temperature and high humidity. The SEM images captured at high magnification of each group of fiber membranes were thoroughly analyzed using software (ImageJ) to evaluate the pore dimensions and porosity of the fiber membrane surfaces. Figure 2 presents the average pore dimensions and porosity of various groups of porous fiber membranes. Here, porosity refers to the proportion of pore area appearing within a single fiber to the total area. Notably, with the addition of SF, the porosity of the fiber surface exhibits a downward trend (Figures 2A). As the SF content increases, the difference in porosity between the pure PLA fiber membrane and the PLA@SF composite fiber membrane becomes more and more distinct. At the same time, the pore size also diminishes (Figures 2B). The occurrence of this phenomenon may be based on the fact that the incorporation of SF into the PLA system enhances the viscosity of the electrospinning solution to some degree and inhibits phase separation during spinning, resulting in less porous fibers (Xue et al., 2019). The results suggest that incorporating SF is an efficient way to control the formation of pore sizes. The technical advantage of electrospinning lies in its ability to construct biomimetic three-dimensional spatial structures, providing a favorable growth microenvironment for cells. The design of a single fiber porous structure has laid a solid structural foundation for supporting cell growth behavior in the future. The statistical results of porosity indicate that the porosity of multiple groups of fiber membrane samples constructed is all greater than 40%, which greatly facilitates the transportation of nutrients and metabolic waste. The pore size of each group of fiber membranes prepared simultaneously does not exceed 0.2 μm, while the cell diameter of SCS is generally between 10 and 20 μm (Kleitman et al., 1995). This pore size ensures the transport of nutrients without allowing cells to sink in, which can also be supported by a previous migration experiment on SCS (Wang et al., 2021).
FTIR analysis was conducted to explore the relationship between PLA and SF. The FTIR spectra of pure PLA, pure SF, and PLA@SF composite fiber membranes are presented in Figure 3. For the pure PLA fiber membranes, characteristic peaks observed near 1720, 1,450, 1,181, and the peaks at 1,081 cm−1 are attributed to the stretching vibrations of C=O, the bending vibrations of CH, and the stretching vibrations of C-C and C-O bonds. Notably, the C=O and C-N stretching vibrations between 1,625 cm−1 and 1,645 cm−1 are associated with amide bond I of centromere fibroin, while the stretching vibrations between 1,522 cm−1 and 1,534 cm−1 correspond to amide bond II of centromere fibroin. The C-N stretching vibration and N-H deformation vibration are also attributed to the β-sheet structure. Furthermore, the strong absorption peak at 1,635 cm−1 is attributed to the α-helical structure of silk fibroin. In the PLA@SF composite fiber membrane, these peaks are enhanced with increasing SF content. Despite varying amounts of SF, the position of the main absorption peak of PLA remains constant, suggesting that incorporating SF does not modify the primary structure of PLA. However, the typical peaks of other bands of PLA show significant changes (from 1,522 cm−1 to 1,534 cm−1). These changes in the characteristic peaks of the PLA@SF composite fiber membrane suggest that SF has been fully integrated into the PLA system, indicating an interaction between the two. The characteristic peaks of silk fibroin (SF) observed in the FTIR spectrum of the PLA@SF composite fiber membrane suggest that SF was retained throughout the preparation of the electrospun membrane and during subsequent treatment processes. To further substantiate this observation, we conducted fluorescent labeling. As illustrated in Figure Supplementary Material S1, the distribution of SF within the PLA@SF composite fiber membrane is uniform. The increased fluorescence intensity indicates a gradient of SF content within the mixed system.
WCA was employed to characterize the hydrophilic properties of the fiber membrane. In this study, the direction of WCA measurement was aligned with the fiber orientation distribution. As illustrated in Figure 4, the WCA values for PLA-SR and PLA-SA are 105.92 ± 1.72° and 94.08 ± 1.04°, respectively, indicating that PLA exhibits hydrophobic characteristics. Compared to PLA-SR, the WCA of PLA-SA is reduced, which can be attributed to the anisotropic wettability of droplets on the nanomaterials distributed along the fiber orientation (Ge et al., 2020). The presence of a wetting energy barrier facilitates the diffusion and water droplets move more easily along the fiber axis than across it (Youngblood and Mccarthy, 1999). This observation echoes the results reported by Zhang et al. (2015). Additionally, WCA measurements taken parallel to the fiber orientation are typically lower than those measured perpendicular to It (Zhou et al., 2015). The hydrophobic nature of PLA hinders cell growth and spreading on its surface, Consequently, its applicability in medical materials is reduced. There are many mature methods for hydrophilic modification of PLA fibers, including chemical surface modification (Li et al., 2024), mixed spinning (Weng et al., 2025), surface coating (Chen and Su, 2011), surface plasma treatment (Abdulkareem et al., 2021), etc. Enhancing the roughness of electrospun fiber filaments—through the introduction of nanoscale pores or nanoparticles—has been shown to increase WCA, resulting in an overall enhancement of hydrophobicity (Zhou et al., 2015; Ma et al., 2007). The decrease in WCA observed in the PLA-PA composite fiber membrane may be due to the hole structures distributed along individual fiber filaments, which better facilitate the diffusion of water droplets in the direction of fiber orientation (Schaub et al., 2013). Silk fibroin (SF) exhibits good hydrophilicity. As the amount of SF incorporated increases, the WCA of PLA@SF-P3 and PLA@SF-P5 gradually decreases, which can be attributed to the increased diffusion area of water. The addition of SF causes a decrease in the contact angle of the fiber membrane. Although the decrease in contact angle only shows a small trend, considering that the content of SF accounts for a very small proportion relative to the overall composite fiber membrane, this downward trend is also reasonable. Consequently, the enhanced wettability of water droplets on the PLA@SF composite fiber membrane suggests that the construction of a nanopore structure and the introduction of SF can enhance the hydrophilicity of PLA and PLA@SF composite fiber membranes, which is advantageous for cell adhesion (Chi et al., 2021).
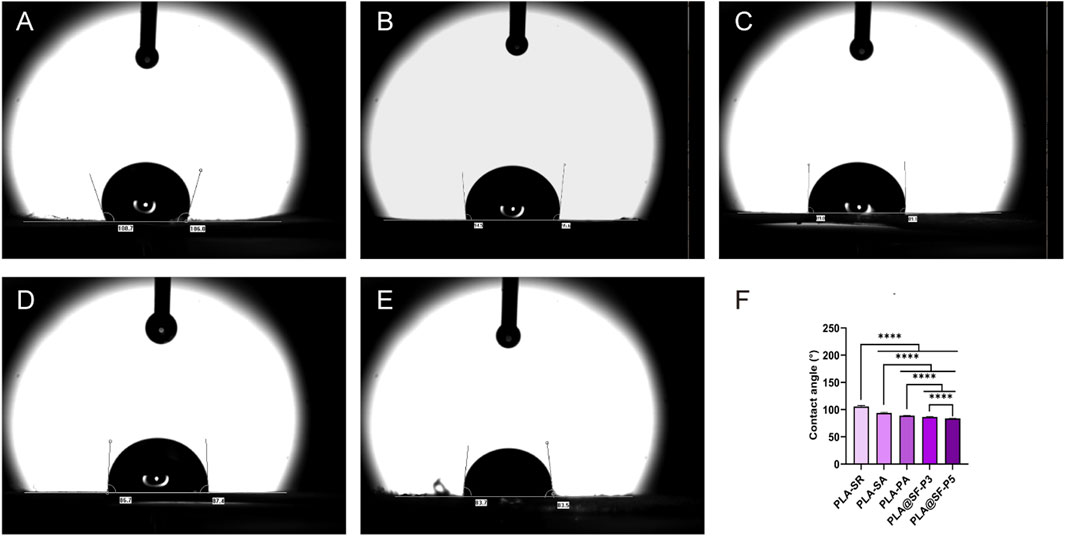
Figure 4. Contact angle test; these images show the true state of water droplets on the membrane surface after 2 s (A) PLA-SR (B) PLA-SA (C) PLA-PA (D) PLA@SF-P3 and (E) PLA@SF-P5. (F) Contact angles: mean ± SEM (one-way ANOVA, n = 4).
Mechanical properties are critical indicators for evaluating biological materials, and adequate mechanical strength is essential. For artificial nerve grafts, the mechanical properties must satisfy the demands of tissue movement during surgical procedures and subsequent recovery processes to ensure the stability of the interface during implantation and tissue movement (He et al., 2024; Vijayavenkataraman, 2020). In this study, The stress and strain of the aligned fiber membrane were assessed in both directions, parallel and perpendicular to the fiber alignment. Figure 5 illustrates the stress-strain curves and the elongation at break for each set of membranes. Compared to the non-porous smooth aligned fiber membrane (PLA-SA), the elongation at break of the randomly distributed fiber membrane (PLA-SR) is significantly higher (Figures 5B). This enhancement is attributed to the random distribution of fibers, which allows for the dispersion of tensile stress from multiple directions. The introduction of porous structure significantly reduces the fracture elongation of PLA fiber membrane in the fiber distribution direction and perpendicular to the fiber distribution direction (Figures 5B,D). Moreover, the tensile strength along the fiber aligned is markedly greater than that in the vertical direction (Figures 5A,C), indicating that the mechanical properties of the aligned distributed fiber membrane are anisotropic, consistent with previous reports (Na et al., 2009). In comparison to PLA-SR, PLA-SA exhibits a higher tensile strength (Figure 5A). Consequently, the PLA-SA fiber membrane is deemed more suitable for promoting nerve growth. In contrast to PLA-SA, the tensile strength of the porous aligned fiber membranes (PLA-PA, PLA@SF-P3, PLA@SF-P5) in the direction parallel to the fibers is comparatively lower. This reduction can be attributed to two main factors: (1) the introduction of a porous structure leads to discontinuities in the fiber architecture, and (2) smooth fiber membranes possess a higher density than their porous counterparts. Measured parallel to the fiber direction, as the content of silk fibroin (SF) increases, The tensile strength of PLA@SF composite fiber membranes (porous) shows a decreasing trend, which is linked to the significantly lower mechanical properties of SF compared to PLA (Yang et al., 2024; Maleki et al., 2020). This observation aligns with previously reported findings (Yang et al., 2024). Nevertheless, even the tensile strength of the sample with the highest SF content (PLA@SF-P5) reached 3.41 ± 0.19 MPa, which is substantially greater than that of natural peripheral nerve tissue (0.272 ± 0.097 MPa) (Borschel et al., 2003). Though the addition of a porous structure and silk fibroin (SF) somewhat reduces the mechanical properties of the composite fiber membrane, it remains adequate for applications in the development of scaffolds to promote nerve regeneration.
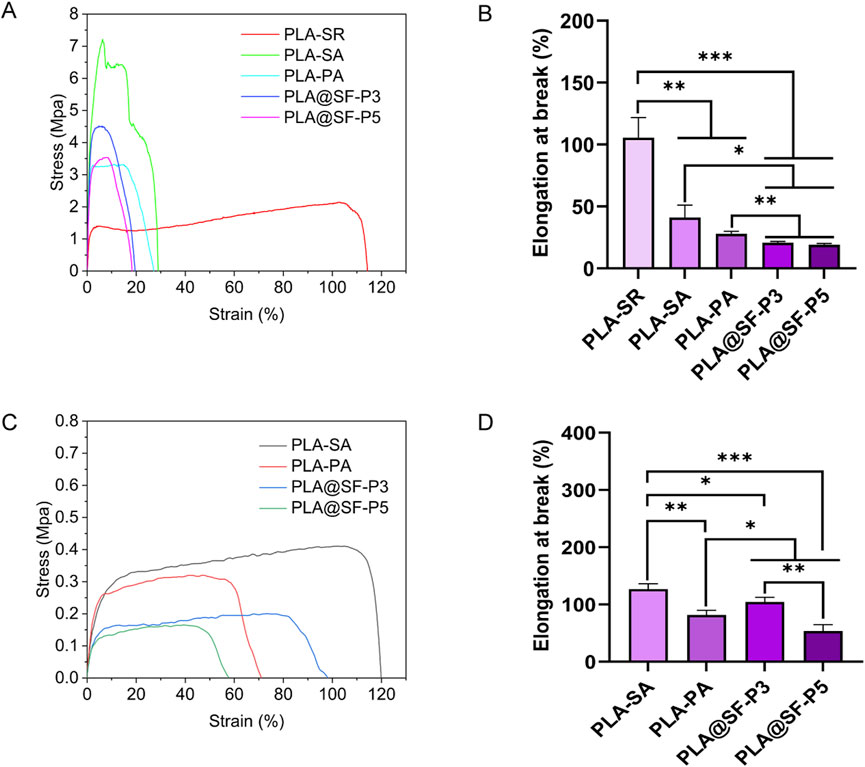
Figure 5. Mechanical properties in parallel directions. (A) Stress–strain curves (B) elongation at break; Vertical direction: (C) Stress–strain curves (D) elongation at break.
To maximize functional recovery following a nerve disconnection, the migration of Schwann cells is essential. These cells move from both the proximal and distal nerve stumps into the nerve bridge, forming cables that aid in axon regeneration (Min et al., 2021). Numerous reports have shown that fiber orientation, fiber size, and the dimensions of the arrangement gap significantly influence cellular growth behavior (Liu et al., 2021; Li et al., 2005). Notably, the construction of aligned topological structures can provide physical guidance channels for the movement of nerve cells (Li et al., 2014; Zhang et al., 2021). To assess whether the topological structure of the fiber membrane, devoid of any components as constructed in this study can stably exist in a bodily fluid environment, we immersed the fiber membranes in PBS. As illustrated in Figure 6A, the aligned topology of the fiber membrane is distinctly visible, with the fibers exhibiting minimal collapse or deformation. The statistical analysis of the fiber aligned angles for each group, presented in Figure 6B, indicates no significant difference in the aligned angles of the fiber membranes before and after immersion, suggesting that the topological structure of the fibers maintains good stability in liquid. In addition, we performed a statistical examination of the pH levels in fiber samples throughout the soaking process. As shown in Figure 6C, the pH of each group of fibers exhibited a gradual decline in the early stages of soaking, eventually stabilizing at a plateau. The degradation products of PLA include lactic acid and its derived small molecular substances (Elmowafy et al., 2019), which can lower the pH of the solution. Given that the in vitro degradation of PLA is an exceptionally slow process, lasting several months (Liu et al., 2019), the relatively stable pH of the PBS solution, combined with the influence of lactic acid, ultimately maintains the degradation pH at a plateau.
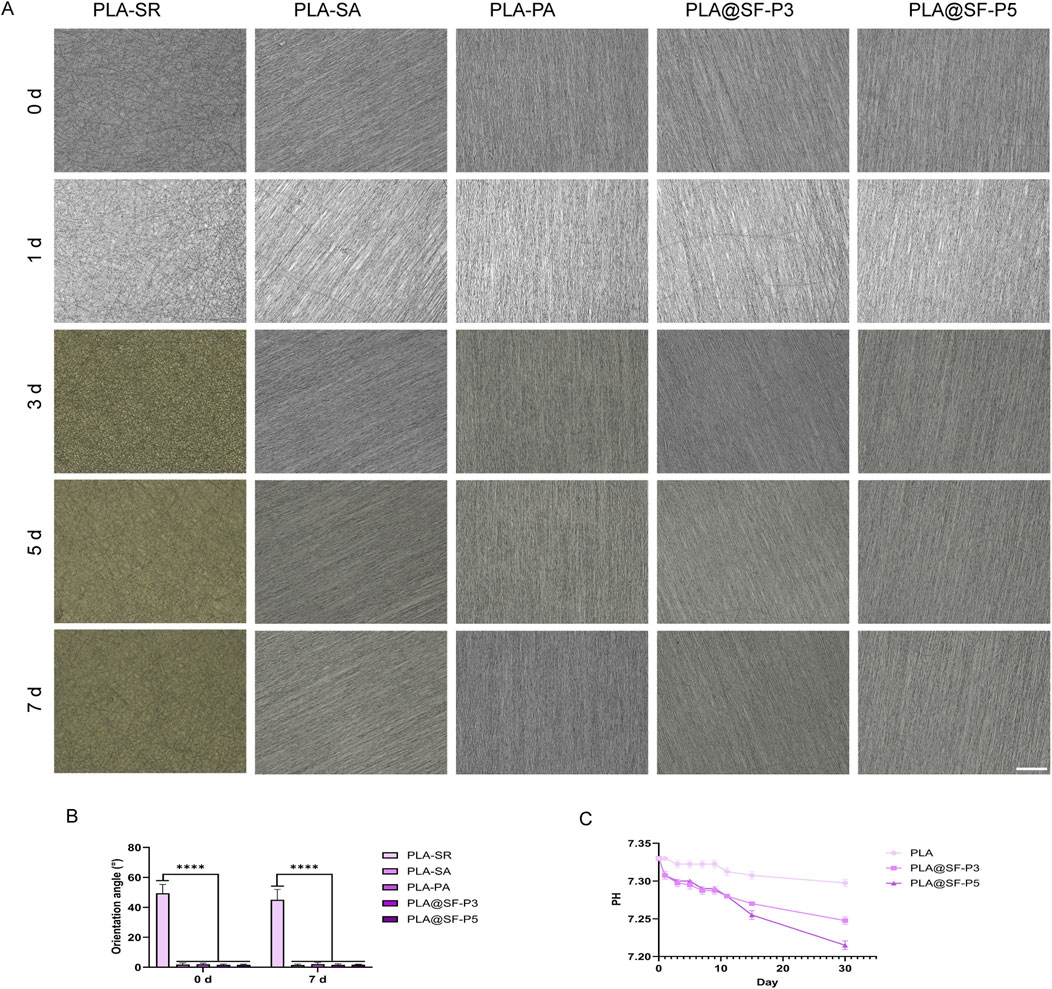
Figure 6. Stability testing and degradation pH of fiber topology structure. (A) Microscopic observation of various fiber membranes immersed in PBS solution under a light microscope (B) The orientation angle of various fiber membranes (C) PH.
3.2 Cytotoxicity and biocompatibility
The MTT method was employed to assess the implication of fiber membranes on cell proliferation and toxicity. RSC96 cells were cultured in complete culture medium, serving as the blank control group, while those cultured in extracts of PLA and PLA@SF composite fiber membranes constituted the experimental group. The cell viability rate reflects whether cells are affected by toxicity. The calculation method for cell viability is based on Formula 1. A rate close to one indicates the material is virtually non-toxic and cells are in good condition. A significantly lower rate suggests some cytotoxicity. As illustrated in Figure 7A, The PLA-PA group had a cell viability rate close to one on Day 1, indicating that PLA is not toxic to RSC96 cells. The cell viability of PLA-PA exhibited a gradual increase after 1, 3, and 5 days of culture. Worth highlighting is the cell viability of PLA@SF-P3 and PLA@SF-P5 was considerably higher than that observed in the PLA-PA group. This enhancement may be attributed to the small amount of silk fibroin (SF) that leached from the PLA@SF composite fiber membrane, which likely promoted cell proliferation (Alessandrino et al., 2019). This finding aligns with the report by Hu et al. (2012), which indicated that electrospun fibroin nanofibers can improve cell growth behavior, such as enhance the adhesion, migration, and promote proliferation. Furthermore, the OD value of PLA@SF-P5 was higher than that of PLA@SF-P3, potentially due to the greater quantity of SF added; a higher addition amount results in increased SF leaching per unit extraction time.
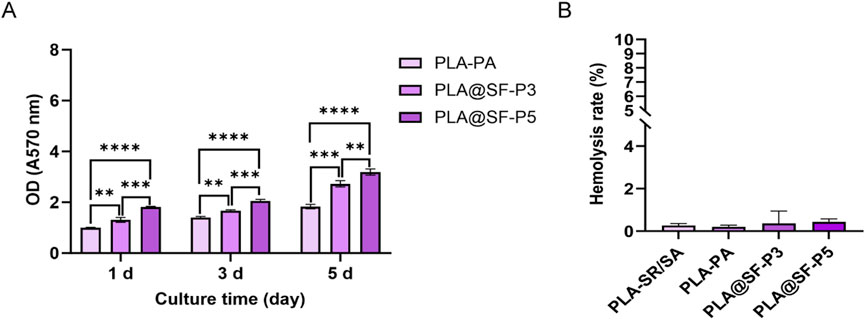
Figure 7. Cytotoxicity and biocompatibility of the electrospun membranes. (A) OD data of RSC96 cells following incubation in electrospun membranes extracts for 1, 3, and 5 days. One-way ANOVA, n = 6. (B) The hemolysis ratio of electrospun membranes.
Hemolysis testing was utilized to evaluate the blood compatibility of the various prepared fiber membranes. The calculation method of hemolysis rate is based on Formula 2. According to the YY/T 1651.1-2019 standard, a hemolysis rate measurement result of less than 5% indicates that the test sample exhibits no hemolysis. As shown in Figure 7B, all fiber membrane groups had hemolysis rates below 1%, well under the 5% threshold, demonstrating that both PLA and PLA@SF composite fiber membranes possess good blood compatibility. Prior investigations have demonstrated that the pore structure of electrospun fibers can influence the hemolysis characteristics of the material. The structure, size, and overall porosity of the holes on the fiber surface may directly or indirectly affect the occurrence of hemolysis. Fibers with larger pores may provide more space for red blood cells to pass through, potentially increasing the risk of hemolysis (Yang et al., 2022). However, the outcomes of the hemolysis test suggest that the pore structure and the addition of SF do not adversely affect the blood compatibility of the constructed PLA fiber membrane. Both cytotoxicity tests and hemolysis tests confirm that the PLA@SF composite fiber membrane meets the fundamental requirements for use as a tissue engineering artificial nerve graft (Vijayavenkataraman, 2020; Wang et al., 2024).
3.3 Response of nerve cells
3.3.1 Proliferation of SCs
SCs are the primary components of peripheral nerves, responsible for forming myelin sheaths, providing support and nutrition to axons, and transforming into a repair phenotype following injury to promote axonal regeneration and target organ reinnervation (Min et al., 2021; Wei et al., 2024). The evaluation of cell proliferation on the fibrous membrane is performed using an EDU kit. As illustrated in Figure 8, the rate of cell proliferation was assessed by the percentage of cells incorporating EDU. Statistical analysis revealed no significant difference between PLA-SA and PLA-SR, indicating that merely altering the distribution direction of fibers does not enhance cell proliferation behavior. Notably, The proliferation of RSC96 cells on PLA-PA was considerably higher compared to those on PLA-SR and PLA-SA, suggesting that the introduction of a hole structure can promote RSC96 cell proliferation (Yadav et al., 2021; Liu et al., 2023). This finding aligns with the report by Xuan et al., which indicated that fibers with well-aligned and nanoporous surfaces can decrease bacterial adhesion and improve cell reactions; moreover, fiber membranes with larger nanopores are more advantageous for the proliferation and differentiation of neural stem cells (NSCs) compared to non-porous fibers. Chen et al. (2020) assert that the high porosity of fiber membranes provides a permeable network structure capable of regulating the exchange of nutrients and transportation of oxygen gas. Samourides et al. (2020) also reported similar experimental results. The experimenters prepared poly (glyceryl sebacate) carbamate (PGSU) scaffolds with different porous structures by changing the polymer concentration of the freeze-drying solution, and studied the effects of porous structures on cell proliferation, tissue ingrowth, and angiogenesis characteristics. The results indicate that pore size controls tissue growth, angiogenesis, and affects the mechanical properties of materials. Figure 8B illustrates that the introduction of silk fibroin (SF) significantly enhanced the proliferation of RSC96 cells on the PLA-PA, aligning well with our predictions. This enhancement may be attributed to the specific amino acid sequence, such as Arg-Gly-Asp (RGD), introduced to the surface of the composite fiber membrane by SF, which functions as a biological signal for cell adhesion—a necessary condition for cell proliferation (Li et al., 2019). Furthermore, the incorporation of SF improves the hydrophilicity of the PLA fiber membrane, thereby facilitating cell spreading on its surface. This characteristic contributes to the superior ability of the PLA@SF composite fiber membrane to support nerve cell proliferation compared to the PLA fiber membrane alone. Additionally, the cell proliferation effect observed with PLA@SF-P5 is markedly different from that of PLA@SF-P3, indicating that an increased amount of SF promotes cell proliferation. Beyond the intrinsic properties of the material, factors like surface porosity, arrangement, pore size, fiber diameter, versus hydrophobicity and hydrophilicity also play significant roles in influencing cell proliferation. Although the porosity and pore size of PLA@SF-P3 are greater than those of PLA@SF-P5, the proliferation effect is more pronounced in PLA@SF-P5 than in PLA@SF-P3, suggesting a complex interplay among the aforementioned factors. The ultimate result is the product of the balance of various factors.
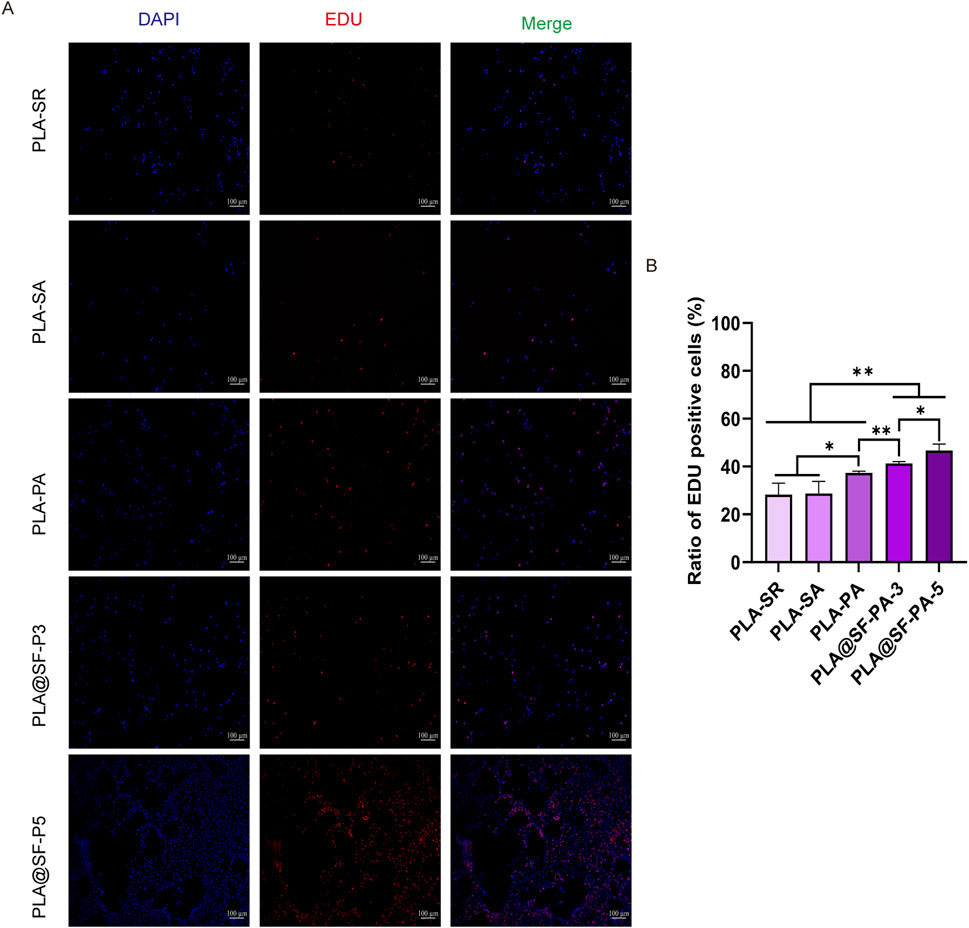
Figure 8. Proliferation of SCs after 24 h of incubation was measured by the EdU assay. (A) Typical fluorescent micrographs depicting proliferating cells on diverse fibrous matrices. Red: EdU, Blue: DAPI, scale bar: 100 μm. (B) The ratio of EdU positive cells. (one-way ANOVA, t-test, n = 5).
3.3.2 Fluorescence staining of SCs
Within the peripheral nervous system, Schwann cells are the principal glial cells. During the repair process following an injury, it is essential to promote their directional arrangement. The directional distribution of Schwann cells facilitates axon guidance and supports axon growth and regeneration. Immunofluorescence techniques were employed to investigate the morphology of RSC96 cells cultured on various fibrous membranes. As shown in Figure 9F, the statistical results indicate that the constructed multiple groups of fiber membranes have significant differences in orientation compared to PLA-SR, indicating stable construction and existence of fiber membrane orientation. Illustrated within Figures 9A–E, RSC96 cells exhibited disordered growth along all directions on the PLA-SR fiber membrane, displaying a broad shape and irregular cell growth behavior. The length-to-width (L/W) ratio was utilized to assess the elongation rate of RSC96 cells on different membranes. Illustrated within Figure 9G, the L/W value of RSC96 cells cultured on PLA-SA, PLA-PA, and PLA@SF was markedly higher than that PLA-SR. This suggests that fiber alignment can effectively direct cellular growth and encourage cell oriented extension. Notably, the L/W ratio of RSC96 cells cultured on the surface of PLA-PA was marginally greater compared to that on PLA-SA, though this difference did not reach statistical significance. This suggests that the simple hole structure can influence the stretching state of cells to some extent, albeit not significantly. A similar phenomenon was observed in the PLA@SF composite fiber membrane group. When silk fibroin (SF) was incorporated, the length-to-width (L/W) ratios of RSC96 cells on PLA@SF-P3 and PLA@SF-P5 fiber membranes exhibited significant differences compared to the PLA-PA fiber membranes. These findings further demonstrate that the nanoporous structure, along with the addition of SF, exerts a synergistic regulatory effect. However, no notable differences were observed between the groups with varying amounts of SF. Overall, the introduction of porous structures and the addition of SF synergistically promote the proliferation of SCS. Undoubtedly, the vigorous proliferation activity of cells brings a sufficient number of cells, and directional structural design can shorten the repair process of nerve damage to the greatest extent possible.
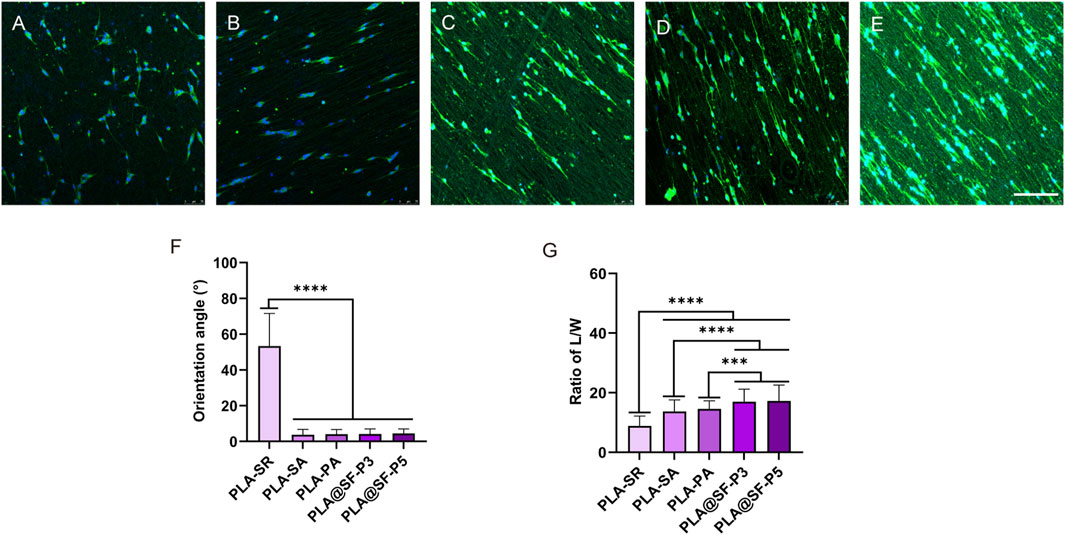
Figure 9. Morphological analysis of SCs on assorted electrospun fibers: green for cytoskeletal labeling, blue for cell nuclear staining. (A) PLA-SR, (B) PLA-SA, (C) PLA-PA, (D) PLA@SF-P3, (E) PLA@SF-P5; scale bar: 75 μm. (F) The orientation angle of various fiber membranes: the angle of fiber orientation is defined as follows—For random fibers, this angle is the angle between the horizontal plane and the di-rection of the cell’s long axis, with the X-axis as the reference for the horizontal plane. For aligned ar-ranged fibers, it is the angle between the direction of fiber arrangement and the long axis of the cell. (G) The ratio of length to width (L/W) of SCs (one-way ANOVA, t-test, n = 100).
3.3.3 SCs morphology
Scanning electron microscopy was employed to further examine RSC96 cells on various fiber membranes. Supplementary Figures S2A−F demonstrates that RSC96 cells adhered tightly to the fibers, exhibiting a disordered diffusion morphology on the PLA-SR fiber membrane, while displaying a fiber orientation distribution. Conversely, on the PLA-SA, PLA-PA, and PLA@SF composite fiber membranes, elongation of RSC96 cells proceeded along a direction parallel to fiber distribution, in agreement with the fluorescence observation results of the cytoskeleton. According to Kuo et al. (2014), Cell adhesion behavior is size-dependent. Moreover, fiber nanotopography exerts distinct influences on cell adhesion among different cell types (Jing et al., 2021). Current observations indicate no significant difference in RSC96 cell adhesion across the different fiber membrane groups. Given that all prepared fiber membranes are of comparable dimensions, we can confidently assert that the porous structure of the fiber surface did not have a notable impact on the in vitro adhesion of RSC96 cells.
3.3.4 Cell migration
Following nerve injury, axonal regeneration necessitates cell migration to restore nerve connections, making the migratory capacity of nerve cells crucial for repair following such injuries (Wang et al., 2021). Given the challenges associated with directly observing the distribution of cells on the fiber surface using light microscopy, this study employed an extraction solution. As illustrated in Figure 10, The analysis showed no significant differences between the PLA-PA group and the blank control group (using complete growth medium). Previous research has indicated that the microporous structure and porosity of the PLA surface can enhance cell migration abilities (Liu et al., 2020). The absence of a significant difference between the PLA-PA and complete culture groups may be attributed to two factors: (1) the use of an extraction solution, which may negate the positive influence of the material’s microstructure on cell migration behavior (2) the inherent resistance of PLA to degradation, with a short extraction duration (24 h) insufficient to significantly impact the cell migration behavior of the extract components in both groups. Notably, compared to the PLA-PA group, the cell migration rate in the PLA@SF composite fiber membrane group exhibited a significant difference, with increased amounts of SF correlating with more pronounced migratory activity.
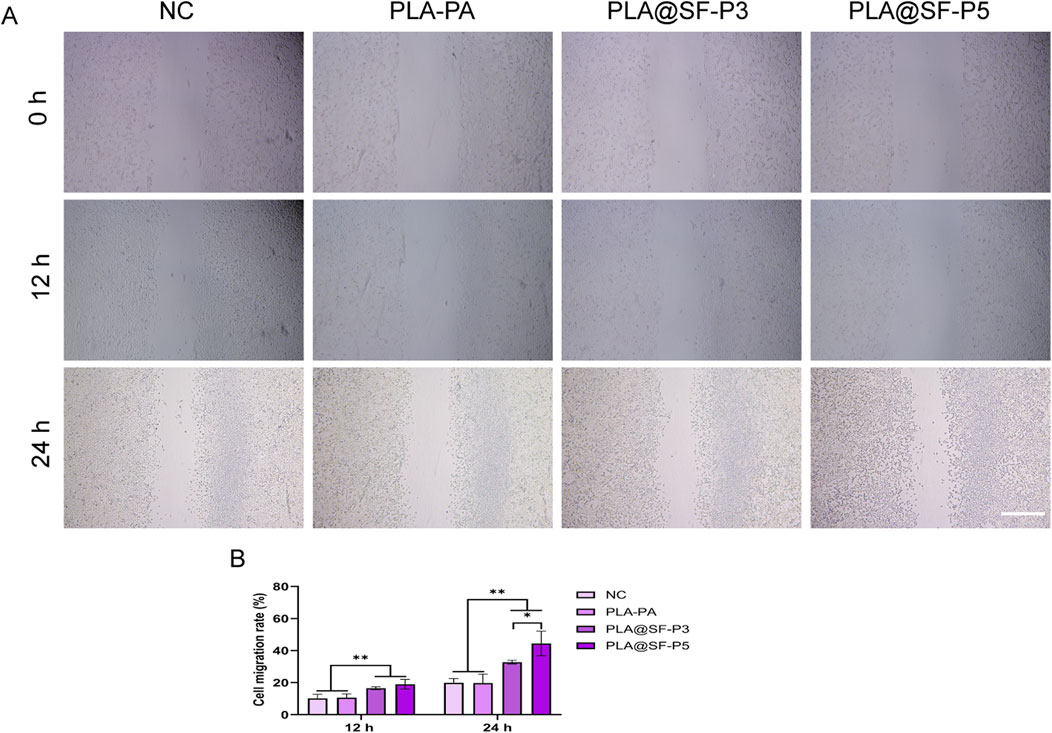
Figure 10. Migration images of cells in different electrospun fiber membrane extraction solutions. (A) SCs scratch (B) Cell migration rate, scale bar:500 μm. (one-way ANOVA, t-test, n = 5).
4 Conclusion
In this study, a high-temperature and high-humidity electrospinning process was employed to prepare PLA and PLA@SF composite fiber membranes featuring aligned porous structures. Characterization of the physical and chemical properties reveals a notable interaction between PLA and SF. The incorporation of SF enhances the hydrophilic properties of the fiber membrane. As the primary component, PLA ensures the excellent mechanical properties of the composite fiber membrane. MTT and hemolysis tests indicate that the PLA@SF composite fiber membrane is non-toxic and exhibits good blood compatibility. Stability experiments demonstrate that the constructed multi-group aligned fiber membrane can reliably maintain its orientation topology in a liquid environment. While PLA degrades slowly, the introduction of SF can accelerate this process to a certain extent; however, insufficient amounts of SF do not yield significant effects. As soaking time increases, the pH values of all fiber membrane groups stabilize. The synergistic effect of SF and the porous structure promotes the growth behavior of RSC96 cells: proliferation and migration. Scratch experiments show that the addition of SF effectively enhances cell migration, which is crucial for accelerating the regeneration of damaged nerves. These findings suggest that in neural tissue engineering, micro-scale alteration of the electrospun fibers’ surface topography, along with the introduction of suitable active ingredients, can serve as an effective strategy to regulate and enhance cell growth behavior.
5 Discussion
In the more than 100 years since the birth of electrospinning technology, there have been numerous developments and related research on biomaterials in the field of tissue engineering and neural regeneration centered around this technology. More and more reports have confirmed that fiber membranes manufactured based on electrospinning technology can effectively regulate the growth behavior of nerve cells through the microscopic morphology clues on the fiber surface (Omidinia-Anarkoli et al., 2019; Denchai et al., 2018). The introduction of fiber orientation distribution, porous structure, or other compound blending methods can have certain regulatory effects on cell adhesion, proliferation, and migration. The stable and effective construction of porous structures is related to the biological function of fiber membranes in this study. The nanopore characteristics on the fiber surface constructed by electrospinning technology can be effectively controlled. By adjusting parameters such as the concentration of polymer solution, solvent type, spinning voltage, flow rate, and receiving distance, as well as using methods such as adding pore forming agents, biaxial spinning, and high temperature and humidity, the pore size, distribution, and connectivity of nanopores on the fiber surface can be controlled (Kalluri et al., 2021; Ahmadi bonakdar and Rodrigue, 2024; Lu et al., 2020; Zhu et al., 2020; Augustine et al., 2019). These methods for regulating the stable generation of pore size have been extensively reported in previous studies. For the field of neural repair, electrospun fibers with high porosity and high specific surface area can provide a good adhesion and proliferation environment for nerve cells, promoting their adhesion and proliferation. Lu et al. (2020) modified a single fiber with pores on a PLLA/DP/Sinp composite electrospun fiber membrane. The results showed that the pore structure could bring higher specific surface area to the fiber membrane scaffold, provide more adhesion sites for cells and opportunities for biochemical signal communication with the surrounding environment, and improve cell adhesion and proliferation; Nanoporous fibers can be loaded with drugs such as neurotrophic factors, and with different modification methods, drug release can be achieved, making the neural repair process more controllable, Sun et al. (2024) developed a genetically engineered electrospun scaffold (GEES) that achieves sustained delivery by loading lipid nanoparticles (LNPs) containing IL-10 plasmid (pIL10) and nerve growth factor (NGF). This stent significantly inhibits the inflammatory response after spinal cord injury (reduces polarization of M1 macrophages) and promotes the differentiation of neural stem cells into neurons, ultimately improving motor function recovery; The deepening of demand is driving the innovation of research methods, and multifunctional and intelligent electrospun scaffolds are gradually becoming the darling of researchers. For example, by combining conductive materials to construct composite scaffolds with electrical activity, neural circuit reconstruction can be promoted through electrical signal stimulation. Qi et al. (2025) studied and prepared a conductive neural conduit, in which the inner layer was blended with conductive polymer PEDOT: PSS and chitosan using electrospinning technology to form a highly oriented arrangement of nanofiber inner walls, endowing the conduit with conductivity. Combined with postoperative electrical stimulation, the catheter significantly promotes nerve regeneration and functional recovery, with effects comparable to autologous nerve transplantation; Constructing a spatiotemporal sequence scaffold to achieve synchronous on-demand degradation and functional reconstruction (Jun et al., 2018). This provides different research ideas for further translating research results into clinical practice.
Data availability statement
The original contributions presented in the study are included in the article/Supplementary Material, further inquiries can be directed to the corresponding author.
Ethics statement
Ethical approval was not required for the studies on animals in accordance with the local legislation and institutional requirements because only commercially available established cell lines were used.
Author contributions
TF: Conceptualization, Funding acquisition, Methodology, Writing – original draft. YaL: Methodology, Software, Visualization, Writing – original draft. YeL: Formal Analysis, Software, Validation, Visualization, Writing – original draft. YS: Data curation, Funding acquisition, Investigation, Writing – original draft. LN: Resources, Visualization, Writing – review and editing. DM: Funding acquisition, Project administration, Supervision, Validation, Writing – review and editing.
Funding
The author(s) declare that financial support was received for the research and/or publication of this article. This research was funded by the Jiangsu Provincial Key Project of Traditional Chinese Medicine Science and Technology Development Plan (Grant No. ZD202428), the Nantong Municipal Health Commission Research Project (Grant No. MS2024041, MS2024039), and the Nantong Science and Technology Project (Grant No. MS2024014).
Conflict of interest
The authors declare that the research was conducted in the absence of any commercial or financial relationships that could be construed as a potential conflict of interest.
Generative AI statement
The authors declare that no Generative AI was used in the creation of this manuscript.
Publisher’s note
All claims expressed in this article are solely those of the authors and do not necessarily represent those of their affiliated organizations, or those of the publisher, the editors and the reviewers. Any product that may be evaluated in this article, or claim that may be made by its manufacturer, is not guaranteed or endorsed by the publisher.
Supplementary material
The Supplementary Material for this article can be found online at: https://www.frontiersin.org/articles/10.3389/fmats.2025.1570738/full#supplementary-material
References
Abdulkareem, A., Kasak, P., Nassr, M. G., Mahmoud, A. A., Al-Ruweidi, M. K. A. A., Mohamoud, K. J., et al. (2021). Surface modification of poly(lactic acid) film via cold plasma assisted grafting of fumaric and ascorbic acid. Polymers 13 (21), 3717. doi:10.3390/polym13213717
Ahmadi Bonakdar, M., and Rodrigue, D. (2024). Electrospinning: processes, structures, and materials. Macromol. 4 (1), 58–103. doi:10.3390/macromol4010004
Ahmed, M., Ramos, T., Wieringa, P., Blitterswijk, C. v., Boer, J. d., and Moroni, L. (2018). Geometric constraints of endothelial cell migration on electrospun fibres. Sci. Rep. 8 (1), 6386. doi:10.1038/s41598-018-24667-7
Alessandrino, A., Fregnan, F., Biagiotti, M., Muratori, L., Bassani, G. A., Ronchi, G., et al. (2019). SilkBridge™: a novel biomimetic and biocompatible silk-based nerve conduit. Biomater. Sci. 7 (10), 4112–4130. doi:10.1039/C9BM00783K
Augustine, R., Zahid, A. A., Hasan, A., Wang, M., and Webster, T. J. (2019). CTGF loaded electrospun dual porous core-shell membrane for diabetic wound healing. Int. J. Nanomed. 14, 8573–8588. doi:10.2147/IJN.S224047
Beris, A., Gkiatas, I., Gelalis, I., Papadopoulos, D., and Kostas-Agnantis, I. (2019). Current concepts in peripheral nerve surgery. Eur. J. Orthop. Surg. Traumatol. Orthop. Traumatol. 29 (2), 263–269. doi:10.1007/s00590-018-2344-2
Borschel, G. H., Kia, K. F., Kuzon, W. M., and Dennis, R. G. (2003). Mechanical properties of acellular peripheral nerve. J. Surg. Res. 114 (2), 133–139. doi:10.1016/S0022-4804(03)00255-5
Chaudhuri, O., Cooper-White, J., Janmey, P. A., Mooney, D. J., and Shenoy, V. B. (2020). Effects of extracellular matrix viscoelasticity on cellular behaviour. Nature 584 (7822), 535–546. doi:10.1038/s41586-020-2612-2
Chen, J.-P., and Su, C.-H. (2011). Surface modification of electrospun PLLA nanofibers by plasma treatment and cationized gelatin immobilization for cartilage tissue engineering. Acta Biomater. 7 (1), 234–243. doi:10.1016/j.actbio.2010.08.015
Chen, S., Guo, R., Liang, Q., and Xiao, X. (2021). Multifunctional modified polylactic acid nanofibrous scaffold incorporating sodium alginate microspheres decorated with strontium and black phosphorus for bone tissue engineering. J. Biomater. Sci. Polym. Ed. 32 (12), 1598–1617. doi:10.1080/09205063.2021.1927497
Chen, Y., Qiu, Y., Chen, W., and Wei, Q. (2020). Electrospun thymol-loaded porous cellulose acetate fibers with potential biomedical applications. Mat. Sci. Eng. C-MATER 109, 110536. doi:10.1016/j.msec.2019.110536
Chi, J., Zhang, X., Wang, Y., Shao, C., Shang, L., and Zhao, Y. (2021). Bio-inspired wettability patterns for biomedical applications. Mater. Horiz. 8 (1), 124–144. doi:10.1039/D0MH01293A
Christopherson, G. T., Song, H., and Mao, H. Q. (2009). The influence of fiber diameter of electrospun substrates on neural stem cell differentiation and proliferation. Biomaterials 30 (4), 556–564. doi:10.1016/j.biomaterials.2008.10.004
Chu, C. C. (2013). Biotextiles as medical implants. in 11 - materials for absorbable and nonabsorbable surgical sutures [M]. Editors M. W. KING, B. S. GUPTA, and R. GUIDOIN (United Kingdom: Woodhead Publishing), 275–334. doi:10.1533/9780857095602.2.275
Dai, Y., Lu, T., Li, L., Zhang, F., Xu, H., Li, H., et al. (2024). Electrospun composite PLLA-PPSB nanofiber nerve conduits for peripheral nerve defects repair and regeneration. Adv. Healthc. Mater. 13 (10), e2303539. doi:10.1002/adhm.202303539
Denchai, A., Tartarini, D., and Mele, E. (2018). Cellular response to surface morphology: electrospinning and computational modeling. Front. Bioeng. Biotechnol. 6, 155. doi:10.3389/fbioe.2018.00155
Ehterami, A., Masoomikarimi, M., Bastami, F., Jafarisani, M., Alizadeh, M., Mehrabi, M., et al. (2021). Fabrication and characterization of nanofibrous poly (L-Lactic acid)/chitosan-based scaffold by liquid-liquid phase separation technique for nerve tissue engineering. Mol. Biotechnol. 63 (9), 818–827. doi:10.1007/s12033-021-00346-3
Elmowafy, E. M., Tiboni, M., and Soliman, M. E. (2019). Biocompatibility, biodegradation and biomedical applications of poly(lactic acid)/poly(lactic-co-glycolic acid) micro and nanoparticles. J. Pharm. Investig. 49 (4), 347–380. doi:10.1007/s40005-019-00439-x
Engler, A. J., Sen, S., Sweeney, H. L., and Discher, D. E. (2006). Matrix elasticity directs stem cell lineage specification. Cell 126 (4), 677–689. doi:10.1016/j.cell.2006.06.044
Entekhabi, E., Haghbin Nazarpak, M., Shafieian, M., Mohammadi, H., Firouzi, M., and Hassannejad, Z. (2021). Fabrication and in vitro evaluation of 3D composite scaffold based on collagen/hyaluronic acid sponge and electrospun polycaprolactone nanofibers for peripheral nerve regeneration. J. Biomed. Mater. Res. Part A 109 (3), 300–312. doi:10.1002/jbm.a.37023
Gao, X., Hou, T., Wang, L., Liu, Y., Guo, J., Zhang, L., et al. (2024). Aligned electrospun fibers of different diameters for improving cell migration capacity. Colloids Surf. B 234, 113674. doi:10.1016/j.colsurfb.2023.113674
Ge, P., Wang, S., Zhang, J., and Yang, B. (2020). Micro-/nanostructures meet anisotropic wetting: from preparation methods to applications. Mater. Horiz. 7 (10), 2566–2595. doi:10.1039/D0MH00768D
Guan, Y., Ren, Z., Yang, B., Xu, W., Wu, W., Li, X., et al. (2023). Dual-bionic regenerative microenvironment for peripheral nerve repair. Bioact. Mater. 26, 370–386. doi:10.1016/j.bioactmat.2023.02.002
Gu, Z., Fan, S., Kundu, S. C., Yao, X., and Zhang, Y. (2023). Fiber diameters and parallel patterns: proliferation and osteogenesis of stem cells. Regen. Biomater. 10, rbad001. doi:10.1093/rb/rbad001
Han, F., Hu, Y., Li, J., Gong, J., Guo, Q., Zhu, C., et al. (2019). In situ silk fibroin-mediated crystal formation of octacalcium phosphate and its application in bone repair. Mater. Sci. Eng. C 95, 1–10. doi:10.1016/j.msec.2018.10.041
He, J., Qiao, L., Li, J., Lu, J., Fu, Z., Chen, J., et al. (2024). Advanced strategies for 3D-printed neural scaffolds: materials, structure, and nerve remodeling. Bio-Des. Manuf. 7 (5), 747–770. doi:10.1007/s42242-024-00291-5
Herrero-Herrero, M., Alberdi-Torres, S., GonzáLEZ-FernáNDEZ, M. L., Vilariño-Feltrer, G., Rodríguez-Hernández, J. C., Vallés-Lluch, A., et al. (2021). Influence of chemistry and fiber diameter of electrospun PLA, PCL and their blend membranes, intended as cell supports, on their biological behavior. Polym. Test. 103, 107364. doi:10.1016/j.polymertesting.2021.107364
Hu, A., Zuo, B., Zhang, F., Lan, Q., and Zhang, H. (2012). Electrospun silk fibroin nanofibers promote Schwann cell adhesion, growth and proliferation. NRR 7 (15), 1171–1178. doi:10.3969/j.issn.1673-5374.2012.15.008
Hu, Y., Wu, Y., Gou, Z., Tao, J., Zhang, J., Liu, Q., et al. (2016). 3D-engineering of cellularized conduits for peripheral nerve regeneration. Sci. Rep. 6, 32184. doi:10.1038/srep32184
Jing, L., Wang, X., Leng, B., Zhan, N., Liu, H., Wang, S., et al. (2021). Engineered nanotopography on the microfibers of 3D-printed PCL scaffolds to modulate cellular responses and establish an in vitro tumor model. ACS Appl. Bio Mater. 4 (2), 1381–1394. doi:10.1021/acsabm.0c01243
Jin, L., Tai, Y., and Nam, J. (2024). Piezoelectric silk fibroin nanofibers: structural optimization to enhance piezoelectricity and biostability for neural tissue engineering. Nano Energy 132, 110367. doi:10.1016/j.nanoen.2024.110367
Jun, I., Han, H. S., Edwards, J. R., and Jeon, H. (2018). Electrospun fibrous scaffolds for tissue engineering: viewpoints on architecture and fabrication. Int. J. Mol. Sci. 19 (3), 745. doi:10.3390/ijms19030745
Kalluri, L., Satpathy, M., and Duan, Y. (2021). Effect of electrospinning parameters on the fiber diameter and morphology of PLGA nanofibers. J. Oral Biol. Craniofacial Res. 4 (2), 1–7. doi:10.31487/j.dobcr.2021.02.04
Khouri, N. G., Bahú, J. O., Blanco-Llamero, C., Severino, P., Concha, V. O., and Souto, E. B. (2024). Polylactic acid (PLA): properties, synthesis, and biomedical applications – a review of the literature. J. Mol. Struct. 1309, 138243. doi:10.1016/j.molstruc.2024.138243
Kleitman, N., and Bunge, R. P. (1995). The axon: structure, function and pathophysiology. in The Schwann cell: morphology and development [M]. Editors S. G. WAXMAN, J. D. KOCSIS, and P. K. STYS (New York: Oxford University Press). doi:10.1093/ACPROF:OSO/9780195082937.003.0005
Kong, Y., Xu, J., Han, Q., Zheng, T., Wu, L., Li, G., et al. (2022). Electrospinning porcine decellularized nerve matrix scaffold for peripheral nerve regeneration. Int. J. Biol. Macromol. 209, 1867–1881. doi:10.1016/j.ijbiomac.2022.04.161
Kuo, C. W., Chueh, D. Y., and Chen, P. (2014). Investigation of size-dependent cell adhesion on nanostructured interfaces. J. Nanobiotechnol. 12, 54. doi:10.1186/s12951-014-0054-4
Lanno, G. M., Ramos, C., Preem, L., Putrinš, M., Laidmäe, I., Tenson, T., et al. (2020). Antibacterial porous electrospun fibers as skin scaffolds for wound healing applications. ACS omega 5 (46), 30011–30022. doi:10.1021/acsomega.0c04402
Lee, J., Oh, S., and Jeon, M. J. (2021). Suture complication rates and surgical outcomes according to the nonabsorbable suture materials used in vaginal uterosacral ligament suspension: polyester versus polypropylene. J. Minim. Invasive Gynecol. 28 (8), 1503–1507. doi:10.1016/j.jmig.2020.12.008
Lee, S., Patel, M., and Patel, R. (2022). Electrospun nanofiber nerve guidance conduits for peripheral nerve regeneration: a review. Eur. Polym. J. 181, 111663. doi:10.1016/j.eurpolymj.2022.111663
Li, W. J., Tuli, R., Okafor, C., Derfoul, A., Danielson, K. G., Hall, D. J., et al. (2005). A three-dimensional nanofibrous scaffold for cartilage tissue engineering using human mesenchymal stem cells. Biomaterials 26 (6), 599–609. doi:10.1016/j.biomaterials.2004.03.005
Liang, D., Hsiao, B. S., and Chu, B. (2007). Functional electrospun nanofibrous scaffolds for biomedical applications. Adv. Drug Deliv. Rev. 59 (14), 1392–1412. doi:10.1016/j.addr.2007.04.021
Li, C., Ouyang, L., Armstrong, J. P. K., and Stevens, M. M. (2021). Advances in the fabrication of biomaterials for gradient tissue engineering. Trends Biotechnol. 39 (2), 150–164. doi:10.1016/j.tibtech.2020.06.005
Li, G., Chen, K., You, D., Xia, M., Li, W., Fan, S., et al. (2019). Laminin-coated electrospun regenerated silk fibroin mats promote neural progenitor cell proliferation, differentiation, and survival in vitro. Front. Bioeng. Biotechnol. 7, 190. doi:10.3389/fbioe.2019.00190
Li, G., Zhao, X., Zhang, L., Wang, C., Shi, Y., and Yang, Y. (2014). Regulating Schwann cells growth by chitosan micropatterning for peripheral nerve regeneration in vitro. Macromol. Biosci. 14 (8), 1067–1075. doi:10.1002/mabi.201400098
Liu, F., Xu, J., Wu, L., Zheng, T., Han, Q., Liang, Y., et al. (2021). The influence of the surface topographical cues of biomaterials on nerve cells in peripheral nerve regeneration: a review. Stem Cells Int. 2021, 8124444. doi:10.1155/2021/8124444
Liu, S., Qin, S., He, M., Zhou, D., Qin, Q., and Wang, H. (2020). Current applications of poly(lactic acid) composites in tissue engineering and drug delivery. Compos. Part B Eng. 199, 108238. doi:10.1016/j.compositesb.2020.108238
Liu, S., Wu, G., Chen, X., Zhang, X., Yu, J., Liu, M., et al. (2019). Degradation behavior in vitro of carbon nanotubes (CNTs)/Poly(lactic acid) (PLA) composite suture. POLYMERS-BASEL 11 (6), 1015. doi:10.3390/polym11061015
Liu, X., Ouyang, Q., Yao, X., and Zhang, Y. (2024b). A facile nanopattern modification of silk fibroin electrospun scaffold and the corresponding impact on cell proliferation and osteogenesis. Regen. Biomater. 11, rbae117. doi:10.1093/rb/rbae117
Liu, X., Sun, Y., Chen, B., Li, Y., Zhu, P., Wang, P., et al. (2022). Novel magnetic silk fibroin scaffolds with delayed degradation for potential long-distance vascular repair. Bioact. Mater. 7, 126–143. doi:10.1016/j.bioactmat.2021.04.036
Liu, X., Yao, X., Ouyang, Q., Oliveira, A. L., Yan, L., and Zhang, Y. (2024a). Nanofiber scaffold-based tissue engineering for the treatment of acute liver failure. Adv. Fiber Mater. 6 (3), 686–712. doi:10.1007/s42765-024-00395-8
Liu, Y., Guo, Q., Zhang, X., Wang, Y., Mo, X., and Wu, T. (2023). Progress in electrospun fibers for manipulating cell behaviors. Adv. Fiber Mater. 5 (4), 1241–1272. doi:10.1007/s42765-023-00281-9
Li, W., Yang, Y., XI, X., and Feng, J. (2024). Hydrophilic modification of polylactic acid fiber and the usage of natural dye for multi-levered improvement of the fabric staining depth and the stability effect. Langmuir 40 (7), 3859–3872. doi:10.1021/acs.langmuir.3c03721
Li, Y., Meng, Q., Chen, S., Ling, P., Kuss, M. A., Duan, B., et al. (2023). Advances, challenges, and prospects for surgical suture materials. Acta Biomater. 168, 78–112. doi:10.1016/j.actbio.2023.07.041
Luo, Y., Lin, Z., and Guo, G. (2019). Biodegradation assessment of poly (lactic acid) filled with functionalized titania nanoparticles (PLA/TiO(2)) under compost conditions. Nanoscale Res. Lett. 14 (1), 56. doi:10.1186/s11671-019-2891-4
Lu, P., and Xia, Y. (2013). Maneuvering the internal porosity and surface morphology of electrospun polystyrene yarns by controlling the solvent and relative humidity. LANGMUIR 29 (23), 7070–7078. doi:10.1021/la400747y
Lu, Z., Wang, W., Zhang, J., Bártolo, P., Gong, H., and Li, J. (2020). Electrospun highly porous poly(L-lactic acid)-dopamine-SiO(2) fibrous membrane for bone regeneration. Mater. Sci. Eng. C 117, 111359. doi:10.1016/j.msec.2020.111359
Maleki, H., Semnani Rahbar, R., and Nazir, A. (2020). Improvement of physical and mechanical properties of electrospun poly(lactic acid) nanofibrous structures. Iran. Polym. J. 29 (9), 841–851. doi:10.1007/s13726-020-00844-2
Ma, M., Gupta, M., Li, Z., Zhai, L., Gleason, K., Cohen, R., et al. (2007). Decorated electrospun fibers exhibiting superhydrophobicity. Adv. Mater. 19 (2), 255–259. doi:10.1002/adma.200601449
Min, B. M., Lee, G., Kim, S. H., Nam, Y. S., Lee, T. S., and Park, W. H. (2004). Electrospinning of silk fibroin nanofibers and its effect on the adhesion and spreading of normal human keratinocytes and fibroblasts in vitro. Biomaterials 25 (7-8), 1289–1297. doi:10.1016/j.biomaterials.2003.08.045
Min, Q., Parkinson, D. B., and Dun, X. P. (2021). Migrating Schwann cells direct axon regeneration within the peripheral nerve bridge. GLIA 69 (2), 235–254. doi:10.1002/glia.23892
Mohammadzadehmoghadam, S., and Dong, Y. (2019). Fabrication and characterization of electrospun silk fibroin/gelatin scaffolds crosslinked with glutaraldehyde vapor. Front. Mater. 6. doi:10.3389/fmats.2019.00091
Na, H., Li, Q., Sun, H., Zhao, C., and Yuan, X. (2009). Anisotropic mechanical properties of hot-pressed PVDF membranes with higher fiber alignments via electrospinning. Polym. Eng. Sci. 49 (7), 1291–1298. doi:10.1002/pen.21368
Omidinia-Anarkoli, A., Rimal, R., Chandorkar, Y., Gehlen, D. B., Rose, J. C., Rahimi, K., et al. (2019). Solvent-induced nanotopographies of single microfibers regulate cell mechanotransduction. ACS Appl. Mater. Interfaces 11 (8), 7671–7685. doi:10.1021/acsami.8b17955
Qian, Y., Lin, H., Yan, Z., Shi, J., and Fan, C. (2021). Functional nanomaterials in peripheral nerve regeneration: scaffold design, chemical principles and microenvironmental remodeling. Mater. Today 51, 165–187. doi:10.1016/j.mattod.2021.09.014
Qi, T., Wang, X., Zhang, J., and Gou, M. (2025). Percutaneous electrical stimulation combined with conductive nerve guidance conduits for peripheral nerve regeneration. Chem. Eng. J. 511, 162124. doi:10.1016/j.cej.2025.162124
Samourides, A., Browning, L., Hearnden, V., and Chen, B. (2020). The effect of porous structure on the cell proliferation, tissue ingrowth and angiogenic properties of poly(glycerol sebacate urethane) scaffolds. Mater. Sci. Eng. C 108, 110384. doi:10.1016/j.msec.2019.110384
Santoro, M., Shah, S. R., Walker, J. L., and Mikos, A. G. (2016). Poly(lactic acid) nanofibrous scaffolds for tissue engineering. Adv. Drug Deliv. Rev. 107, 206–212. doi:10.1016/j.addr.2016.04.019
Schaub, N. J., Britton, T., Rajachar, R., and Gilbert, R. J. (2013). Engineered nanotopography on electrospun PLLA microfibers modifies RAW 264.7 cell response. ACS Appl. Mater. Interfaces 5 (20), 10173–10184. doi:10.1021/am402827g
Sun, Y., Wu, J., Zhou, L., Wang, W., Wang, H., Sun, S., et al. (2024). Genetically engineered electrospinning contributes to spinal cord injury repair by regulating the immune microenvironment. Front. Bioeng. Biotechnol. 12, 1415527. doi:10.3389/fbioe.2024.1415527
Suryavanshi, J. R., Cox, C., Osemwengie, B. O., Jones, H. B., and MacKay, B. J. (2020). Sutureless repair of a partially transected median nerve using Tisseel glue and Axoguard nerve protector: a case report. Microsurgery 40 (8), 896–900. doi:10.1002/micr.30593
Tao, J., Zhang, J., Du, T., Xu, X., Deng, X., Chen, S., et al. (2019). Rapid 3D printing of functional nanoparticle-enhanced conduits for effective nerve repair. Acta Biomater. 90, 49–59. doi:10.1016/j.actbio.2019.03.047
Vijayavenkataraman, S. (2020). Nerve guide conduits for peripheral nerve injury repair: a review on design, materials and fabrication methods. Acta Biomater. 106, 54–69. doi:10.1016/j.actbio.2020.02.003
Wang, H. B., Mullins, M. E., Cregg, J. M., McCarthy, C. W., and Gilbert, R. J. (2010). Varying the diameter of aligned electrospun fibers alters neurite outgrowth and Schwann cell migration. Acta Biomater. 6 (8), 2970–2978. doi:10.1016/j.actbio.2010.02.020
Wang, S., Wen, X., Fan, Z., Ding, X., Wang, Q., Liu, Z., et al. (2024). Research advancements on nerve guide conduits for nerve injury repair. Rev. Neurosci. 35 (6), 627–637. doi:10.1515/revneuro-2023-0093
Wang, Y., Zhang, F., Zhang, Y., Shan, Q., Liu, W., et al. (2021). Betacellulin regulates peripheral nerve regeneration by affecting Schwann cell migration and axon elongation. Mol. Med. 27 (1), 27. doi:10.1186/s10020-021-00292-5
Wei, C., Guo, Y., Ci, Z., Li, M., Zhang, Y., and Zhou, Y. (2024). Advances of Schwann cells in peripheral nerve regeneration: from mechanism to cell therapy. Biomed. Pharmacother. 175, 116645. doi:10.1016/j.biopha.2024.116645
Weng, Q.-H., Hu, M.-H., Wang, J.-F., and Hu, J. J. (2025). Enhancing the flexibility and hydrophilicity of PLA via polymer blends: electrospinning vs. Solvent casting. Polymers 17 (6), 800. doi:10.3390/polym17060800
Xie, J., Willerth, S. M., Li, X., Macewan, M. R., Rader, A., Sakiyama-Elbert, S. E., et al. (2009). The differentiation of embryonic stem cells seeded on electrospun nanofibers into neural lineages. Biomaterials 30 (3), 354–362. doi:10.1016/j.biomaterials.2008.09.046
Xuan, H., Li, B., Xiong, F., Wu, S., Zhang, Z., Yang, Y., et al. (2021). Tailoring nano-porous surface of aligned electrospun poly (L-Lactic acid) fibers for nerve tissue engineering. Int. J. Mol. Sci. 22 (7), 3536. doi:10.3390/ijms22073536
Xue, J., Wu, T., Dai, Y., and Xia, Y. (2019). Electrospinning and electrospun nanofibers: methods, materials, and applications. Chem. Rev. 119 (8), 5298–5415. doi:10.1021/acs.chemrev.8b00593
Yadav, P., Beniwal, G., and Saxena, K. K. (2021). A review on pore and porosity in tissue engineering. Mater. Today Proc. 44, 2623–2628. doi:10.1016/j.matpr.2020.12.661
Yamada, M., Tanemura, K., Okada, S., Iwanami, A., Nakamura, M., Mizuno, H., et al. (2007). Electrical stimulation modulates fate determination of differentiating embryonic stem cells. Stem Cells 25 (3), 562–570. doi:10.1634/stemcells.2006-0011
Yang, L., Wang, X., Xiong, M., Liu, X., Luo, S., Luo, J., et al. (2024). Electrospun silk fibroin/fibrin vascular scaffold with superior mechanical properties and biocompatibility for applications in tissue engineering. Sci. Rep. 14 (1), 3942. doi:10.1038/s41598-024-54638-0
Yang, Y., Du, Y., Zhang, J., Zhang, H., and Guo, B. (2022). Structural and functional design of electrospun nanofibers for hemostasis and wound healing. Adv. Fiber Mater. 4 (5), 1027–1057. doi:10.1007/s42765-022-00178-z
Yan, J., Wu, R., Liao, S., Jiang, M., and Qian, Y. (2020). Applications of polydopamine-modified scaffolds in the peripheral nerve tissue engineering. Front. Bioeng. Biotechnol. 8, 590998. doi:10.3389/fbioe.2020.590998
Yao, X., Zou, S., Fan, S., Niu, Q., and Zhang, Y. (2022). Bioinspired silk fibroin materials: from silk building blocks extraction and reconstruction to advanced biomedical applications. Mate.today Bio 16, 100381. doi:10.1016/j.mtbio.2022.100381
Yi, S., Xu, L., and Gu, X. (2019). Scaffolds for peripheral nerve repair and reconstruction. Exp. Neurol. 319, 112761. doi:10.1016/j.expneurol.2018.05.016
Youngblood, J. P., and Mccarthy, T. J. (1999). Ultrahydrophobic polymer surfaces prepared by simultaneous ablation of polypropylene and sputtering of poly(tetrafluoroethylene) using radio frequency plasma. Abstr. Pap. AMER Chem. Soc. 218, 6800–6806. doi:10.1021/ma9903456
Zhang, F., Si, Y., Yu, J., and Ding, B. (2023). Electrospun porous engineered nanofiber materials: a versatile medium for energy and environmental applications. Chem. Eng. J. 456, 140989. doi:10.1016/j.cej.2022.140989
Zhang, M., Wang, L., Hou, Y., Shi, W., Feng, S., and Zheng, Y. (2015). Controlled smart anisotropic unidirectional spreading of droplet on a fibrous surface. Adv. Mater. 27 (34), 5057–5062. doi:10.1002/adma.201502143
Zhang, S., Wang, J., Zheng, Z., Yan, J., Zhang, L., Li, Y., et al. (2021). Porous nerve guidance conduits reinforced with braided composite structures of silk/magnesium filaments for peripheral nerve repair. Acta Biomater. 134, 116–130. doi:10.1016/j.actbio.2021.07.028
Zhou, Q., Xie, J., Bao, M., Yuan, H., Ye, Z., Lou, X., et al. (2015). Engineering aligned electrospun PLLA microfibers with nano-porous surface nanotopography for modulating the responses of vascular smooth muscle cells. J. Mater. Chem. B 3 (21), 4439–4450. doi:10.1039/C5TB00051C
Keywords: polylactic acid, silk fibroin, electrospun fiber membranen, nanotopography, nerve regeneration
Citation: Feng T, Liu Y, Lv Y, Shao Y, Niu L and Mi D (2025) Nanopore structure of PLA@SF aligned composite electrospun fiber membrane enhances growth behavior of peripheral nerve cells. Front. Mater. 12:1570738. doi: 10.3389/fmats.2025.1570738
Received: 04 February 2025; Accepted: 28 April 2025;
Published: 09 May 2025.
Edited by:
Dan Li, Jinzhou Medical University, ChinaReviewed by:
Xiang Yao, Donghua University, ChinaBhavani Patel, Medline Industries, LP, United States
Copyright © 2025 Feng, Liu, Lv, Shao, Niu and Mi. This is an open-access article distributed under the terms of the Creative Commons Attribution License (CC BY). The use, distribution or reproduction in other forums is permitted, provided the original author(s) and the copyright owner(s) are credited and that the original publication in this journal is cited, in accordance with accepted academic practice. No use, distribution or reproduction is permitted which does not comply with these terms.
*Correspondence: Daguo Mi, bWlkYWd1b0AxMjYuY29t
†These authors have contributed equally to this work