- 1School of Metallurgy and Environment, Central South University, Changsha, China
- 2School of Chemistry, Beihang University, Beijing, China
- 3Aerospace Internet of Things Technology Co., Ltd., Beijing, China
Nanotechnology, centered on nanomaterials, represents a cutting-edge research domain. This review focuses on amorphous micro-nano materials (AMNMs), presenting their classification, structural features, basic properties, and synthesis methods. It details the research progress of AMNMs, including their structural characteristics, single-scale and multiscale composite structures, and construction processes. AMNMs exhibit significant potential in various applications, such as lightweight and high-strength materials, electronics and energy storage, medicine, and aerospace. However, challenges such as controllable synthesis and assembly into macroscopic materials remain. Future research should concentrate on developing new theoretical frameworks and synthesis methods to facilitate the practical application of AMNMs, deepening our understanding and driving their widespread use across multiple industries.
1 Introduction
Nanomaterials serve as a crucial symbol of the contemporary technological revolution, embodying humanity’s deepening comprehension and transformation of the objective world. Nanotechnology, with nanomaterials at its core, has evolved into one of the cutting-edge scientific and technological frontiers in the 21st century. It has been designated as a major national strategic development direction by countries globally. Nanotechnology research operates in the mesoscopic field between the microscopic and macroscopic levels, encompassing cluster sizes ranging from a few to tens of atoms up to the micron scale (Zhang, 2001). As Nobel laureate Richard P. Feynman predicted in his 1959 speech “There’s Plenty of Room at the Bottom,” manipulating micro-scale entities can lead to a significantly expanded range of physical properties (Feynman, 1956). Nanotechnology, through research on synthesis, preparation, structure-activity relationships, and practical applications, has opened up a new dimension for humanity’s understanding of the world at an astonishing pace.
In a broad sense, materials with at least one dimension in the three-dimensional space at the nanometer scale or materials with those as constituent units can be called nanomaterials. Therefore, nano-units include three types: zero-dimensional (0 D), one-dimensional (1 D), and two-dimensional (2 D), and the corresponding forms are nanoclusters or particles, nanowires or rods, nanosheets or films, etc. (Figure 1). In addition, although bulk nanomaterials and composite nanomaterials can reach the micron level and above in spatial scale, their substructures are composed of a large number of nano-units and still have nano-characteristics. Compared with the corresponding bulk materials, nanomaterials exhibit peculiar physical and chemical properties under the action of nano-effects, and are widely used in all aspects of today’s society. For example, the application of carbon nanotubes and graphene in energy storage such as lithium-ion batteries, fuel cells, and supercapacitors; the application of noble metal-based nanomaterials in environmental protection fields such as automobile exhaust purification and petroleum desulfurization; and nano-TiO2, nano-hydroxyapatite in the application of biomedicine such as antibacterial and stomatology.
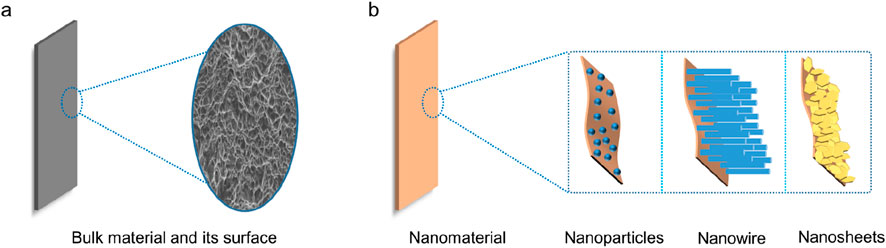
Figure 1. The scheme of bulk material (a) and nanomaterial including nanoparticles, nanowires, and nanosheets (b).
The morphology of nanomaterials determines their functions and uses. The morphology here refers not only to the geometric structure in three-dimensional space, but more importantly, to the atomic arrangement. In the study of solid-state material chemistry, perfect crystals are often used as models to investigate the properties of materials when the atomic arrangement satisfies both short-range order (less than 1 nm) and long-range order (greater than 1 nm). However, perfect crystals do not actually exist, and their application value under actual service scenario is even less. Therefore, in the synthesis and preparation design of conventional crystalline nanomaterials, the properties of materials are often improved or expanded by deliberately introducing defects such as vacancies, atomic doping, dislocations, grain boundaries or phase interfaces. Different from crystalline nanomaterials, amorphous nanomaterials are characterized by short-range order and long-range disorder that is rich in defect structures, showing macroscopic uniformity and isotropy, and exhibiting microscopic reaction mechanism and macroscopic mechanical properties, out of the unique properties compared with crystalline nanomaterials (Guo, 2021; Andersen, 1972). Therefore, a large number of studies on the design, synthesis and performance regulation of amorphous nanomaterials have emerged in recent years.
In recent years, significant progress has been made in the research of amorphous materials. Researchers have synthesized a series of amorphous materials, including five types of amorphous substrate materials (Sun et al., 2024), two-dimensional amorphous materials (Tian et al., 2023; Li et al., 2023), and three-dimensional porous materials (Lee et al., 2024). They have explored the synthesis methods, characterization techniques, and reaction mechanisms of amorphous materials, and promoted their applications in fields such as energy, catalysis, and medicine (Baghestani et al., 2024; Jia et al., 2024; Meng et al., 2024). Based on the design of the structure-activity relationship, the disordered structure of amorphous materials provides a theoretical platform and a research and development direction for the development of new materials (Tian et al., 2023; Zhang et al., 2023). However, when considering the application of amorphous materials in actual industrial production, there is currently a lack of relevant summaries and collations for the design of amorphous materials with multi-scale composite structures.
The study of amorphous nanomaterials has greatly broadened the family of nanomaterials, led new research directions, and prompted the creation and invention of a large number of new concepts, methods, theories, and materials. Amorphous nanomaterials are in a metastable state in terms of energy, and have complex electronic states and coordination bonding relationships. Therefore, a deep understanding of the structural characteristics and physical and chemical properties of amorphous nanomaterials is crucial for the synthesis and preparation of new amorphous nanostructures. Both the construction of theoretical framework and its engineering application are of great significance. Based on this, this paper firstly introduces the current research basis of amorphous nanomaterials, including the structural characteristics, basic properties and synthesis process of amorphous materials; followed by the structure and multi-level composite amorphous nanostructures, and their construction process; finally, the development prospects of amorphous micro-nano materials are discussed.
2 Basis of amorphous materials
According to the classification of modern condensed matter physics, solid phase substances can be divided into crystals, quasicrystals and glasses, among which quasicrystals can usually be regarded as a type of crystals. The research on crystals can be traced back to the middle of the 17th century. The law of constancy of interfacial angles (Steno’s law) proposed by the Danish geologist Nicolaus Steno in 1669 laid the foundation for geometric crystallography (Qin, 2004). Subsequently, the study of crystallography entered a stage of rapid development. Scholars from various countries successively participated in the establishment of rich research methods and perfect theoretical systems, such as X-ray diffraction technology as the basis of crystal structure methodology and 17 plane groups and 230 space groups (Huang, 2014). Quasicrystals were first discovered in 1982 (Shechtman et al., 1984). Although the internal atomic arrangement does not have the translational symmetry of crystals, it has five-fold symmetry or higher rotational symmetry, while the rotational symmetry of crystals is usually quadratic, cubic, quartic or sextic (Figure 2). In the study of crystals and quasicrystals, X-ray diffraction and electron microscopy have greatly promoted the development of crystal physics and solid-state physics (shown in the insets of Figure 2). For instance, in recent research, deep learning techniques have been integrated with X-ray diffraction data analysis, enabling more accurate identification of crystal structures and phase transitions (Spurgeon et al., 2021). Additionally, advanced electron microscopy techniques, have been crucial for exploring the atomic-scale structures of materials, providing new insights into the physical properties of crystals and quasicrystals (Suarez, 2024; Choudhary et al., 2022). Human understanding of ordered systems is approaching maturity.
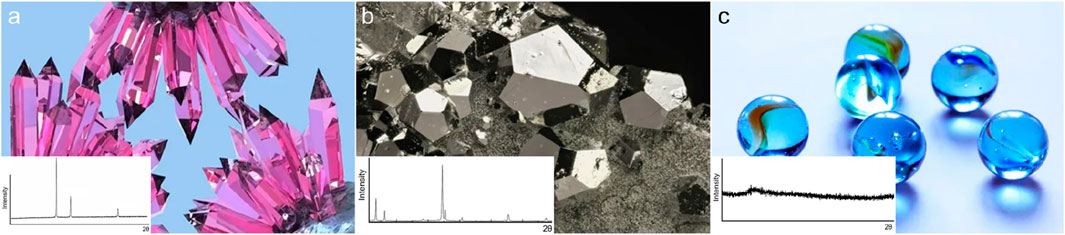
Figure 2. Optical images of crystal (a), quasicrystals (b), and glass (c). The insets demonstrate the expected XRD patterns.
Amorphous substances are also known as glasses, the atomic structure of which is relatively complex, does not show periodicity and translational symmetry, and only has a short program in a small area of a few atoms (Stachurski, 2011). Although humans have a long history of using amorphous materials, which can be traced back to the manufacture and use of glass by the ancient Egyptians more than 4,000 years ago, the research on metal-based amorphous materials and the methods and theories of amorphization only began in the second half of the 20th century. The traditional glass manufacturing process was developed to the manufacture of amorphous metal or alloy materials (Klement et al., 1960). In recent years, the research on amorphous materials has developed rapidly, and a large number of researchers in the community have devoted themselves to the research of amorphous materials, building a solid foundation in theory and application.
2.1 Structural features
(1) Long-range disorder. The atomic arrangement of amorphous materials is not periodic, so the study of its short-range ordered local structure cannot directly use the theories and methods applied to crystal structures. In order to describe the local structure of amorphous materials, several structural models have been proposed, including microcrystalline model, hard-sphere random close-packed model, random network model, and random coil model (Wu et al., 2021). To better understand the local structure of amorphous materials, the atomic radial distribution function RDF is also introduced. RDF can be derived using X-ray, electron, or neutron beams of appropriate wavelengths as probes, or using X-ray absorption fine structure spectroscopy (EXAFS) (Wilding et al., 2006; Cockayne, 2007). In general, the radial distribution function of amorphous materials exhibits only short-range peaks (Figure 3a), while crystalline materials exhibit long-range peaks. In addition, amorphous materials are rich in defect structures, including atomic-sized dangling bonds and nanoscale voids and doping.
(2) Electronic state. In the description of electronic states, it was initially considered to be based on the periodic arrangement of atoms. According to the energy band theory, the wave function of electrons in crystal materials can be described by Bloch function, and the state of electrons can be marked by wave vector (k). Subsequent studies have shown that electrons in amorphous materials also have energy band structures, and various energy band models to describe electronic states have been proposed. For example, the localized electronic state model of disordered system proposed by P. W. Anderson in 1958, which laid the foundation for the study of disordered systems in amorphous physics (Palmer et al., 1984).
(3) Diffraction pattern. From the perspective of X-ray diffraction, due to the short-range order of amorphous materials, a “steamed bun” peak will appear in the diffraction spectrum instead of a sharp crystal diffraction peak. Usually, to distinguish the amorphous structure of the material, it is not enough to use XRD only, because when there is a small number of nanocrystals in the amorphous material, its spectrum also appears as a steamed bun peak (Huang, 2014). At this time, high-resolution transmission electron microscopy (HR-TEM) and selected area electron diffraction (SAED) are required for characterization (Figures 3b,c). Electron diffraction patterns of amorphous materials appear as a diffuse central spot.
(4) Metastable state. Compared with the corresponding crystalline materials, amorphous materials are in a metastable state and have a tendency of crystallization transition. The crystallization process is a structural relaxation process, in which small changes in the relative positions of atoms mainly occur, and then the material gradually tends to a steady state. This process depends on the atomic diffusion coefficient, interfacial energy, and melting entropy of the material at a certain temperature, which will be discussed later.
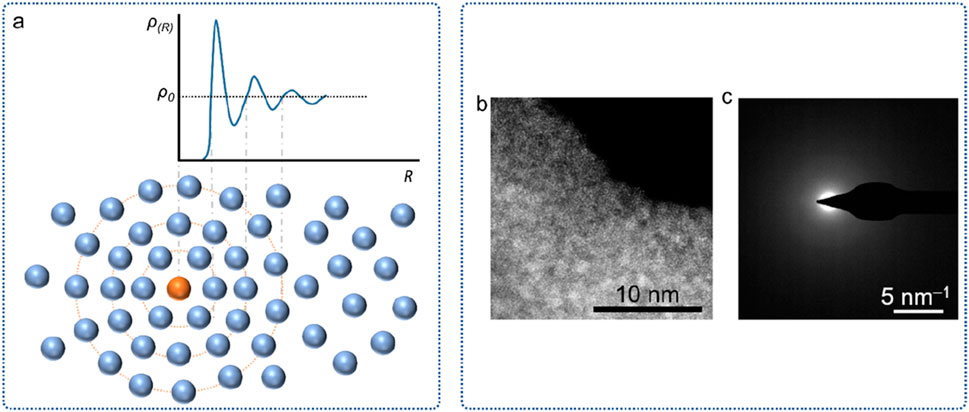
Figure 3. The structural features of amorphous materials. (a) RDF plot and the atomic structural models. (b) HR-TEM image. (c) SAED image.
2.2 Basic properties
(1) Electrical properties. Taking amorphous semiconductor materials as an example, its electronic state has two states: extended state and localized state, so it exhibits different conduction mechanisms at diverse temperatures (Huang, 2014). When the temperature is high, the electrons absorb energy and undergo excitation transitions to form a diffuse state conductance; when the temperature is low, the electrons are excited to a localized state with a tail and transition to a tail state with the help of phonons; further lowering the temperature, electrons are below the Fermi level, and can only form localized state conductance by means of phonon transitions to adjacent empty states above the Fermi level. Amorphous nanomaterials usually have special dielectric and piezoelectric effects.
(2) Optical properties. Amorphous materials have intrinsic absorption similar to crystals, that is, the process in which valence electrons absorb photons and transition to the conduction band to form electron-hole pairs. In addition, due to the presence of localized electronic states in amorphous materials, the electronic transition process also depends on the localized range of the localized state wave function. Amorphous nanomaterials have a high concentration of unsaturated coordination and structural defects, and can usually absorb infrared light and ultraviolet-visible light.
(3) Chemical properties. There are a large number of unsaturated coordination bonds in the local structure of the interior and surface of amorphous materials, which can be used as highly active sites for chemical reactions, and have very important theoretical research and practical application significance in the field of electrocatalysis. In addition, amorphous materials also have good corrosion resistance, and have good stability in harsh environments such as oceans and space environments.
(4) Mechanical properties. Due to the disordered arrangement and isotropy of internal atoms, and the absence of crystal defects such as dislocations and grain boundaries, amorphous materials often exhibit mechanical properties such as high strength, high toughness, high hardness, high viscoelasticity, and high fatigue resistance. For example, the disordered structure in amorphous alloys is similar to grain boundaries, and grain boundary migration and viscous rheology are prone to occur, so the tensile strength and hardness of amorphous alloys are usually three-times higher than that of corresponding crystalline alloys. The advantages of amorphization often outweigh the effect of fine grain strengthening.
2.3 Stability of amorphous materials
Amorphous substances in nature such as obsidian are considered to be one of the most stable substances, they can exist stably for hundreds of millions of years, but because amorphous materials are in a metastable state, they always have a tendency to spontaneously crystallize thermodynamically. Researchers are very concerned about the stability of the prepared amorphous materials. It is generally believed that the influencing factors of amorphous stability include kinetic factors, configuration entropy, and size effects.
The kinetic factor refers to that in the amorphous solid obtained by rapid cooling, the high viscosity of the system hinders the formation of crystals. Therefore, according to the viscosity-temperature relationship, keeping the system at a lower temperature can ensure that the crystallization process is blocked break, thereby maintaining the stability of the amorphous. Under service conditions, when the local temperature or pressure of the material is too high, it may cause the crystallization of the amorphous. The form-position entropy (Sc) is used to describe the degree of confusion caused by the size difference or irregular arrangement of the system’s constituent particles (Lichtenthaler et al., 1973; Pinal, 2008; Ye et al., 2015). The stability of amorphous depends on the potential barrier of particle rearrangement, and the entropy mainly affects the viscosity of the system to increase the potential barrier, so entropy is usually a key parameter to discuss the stability of amorphous.
The size effect means that when there is a large difference in the size of the particles constituting the amorphous, the rearrangement barrier will increase, thereby improving the stability of the amorphous. For amorphous alloys, when the atomic radius difference of components is greater than 10%, the stability of the amorphous alloy increases significantly. What needs to be pointed out here is that alloying may occur between adjacent atoms of components, which will reduce the stability of amorphous. In short, the factors affecting the stability of amorphous materials are complex, and there is no unified theory, so it is necessary to analyze and calculate specific amorphous materials.
2.4 Preparation methods
Based on the above, two problems need to be solved in the preparation of amorphous materials: creating a high crystallization barrier and hindering the crystallization transition. At present, the relatively mature preparation methods of amorphous materials are mainly divided into the following three categories (Figure 4). Several commonly used amorphous preparation methods are briefly described below.
(1) Gas phase method. Vapor phase methods include physical or chemical vapor deposition (PVD/CVD), vacuum evaporation, magnetron sputtering and other means, usually to obtain thin film amorphous materials, which are widely used in the preparation of amorphous semiconductor materials (Li et al., 2011). Unlike melt quenching, vapor deposition condenses hot vapor onto a supercooled substrate (Figure 5A). The principle of the magnetron sputtering method is similar. The polycrystalline sample or powder is pre-cast into a sputtering target, and the components in the target are dissociated in gaseous form under vacuum conditions, and deposited on the supercooled substrate in disorder.
(2) Liquid phase method. The liquid phase method includes melt quenching method, liquid phase reaction method and electrochemical method. Among them, the minimum cooling rate required by the melt quenching method is called the crystallization critical cooling rate. At cooling above this rate, glassy alloys can be obtained (Figure 5B). The commonly used quenching rate is greater than 105°C/s (Axinte, 2012). In 1960, Professor Pol Duwez of the California Institute of Technology first reported the quenching method as a method for studying amorphous materials in the journal Nature (). Although the mechanism of glass transition is not yet fully understood, the method of preparing amorphous materials by quenching melts has already achieved industrial applications (Gao et al., 2016; Li et al., 2016). The liquid-phase reaction method and electrochemical method usually control the random arrangement of growth particles in an aqueous or organic solution system at room temperature or at a temperature not higher than 200°C to prepare amorphous materials with a certain morphology. The next section of this chapter provides a systematic description of these two methods.
(3) Solid phase method. Solid phase methods include laser radiation, thermal decomposition, ion implantation and other means. When irradiated by laser or electron beams with high energy density (up to 106 W/cm2 or higher), the surface of the solid material will be partially melted and an amorphous layer will be formed (Figure 5C) (Qiao et al., 2020; Ozden and Morley, 2021). The thermal decomposition method refers to the decomposition of crystalline materials to produce disordered structures. For example, the preparation of glassy carbon uses solid-state thermal decomposition. Ion implantation refers to the implantation of high-energy amorphous ions into the surface of crystalline materials. The implanted ion components and implantation depth mainly depend on the properties and surface states of crystalline materials.
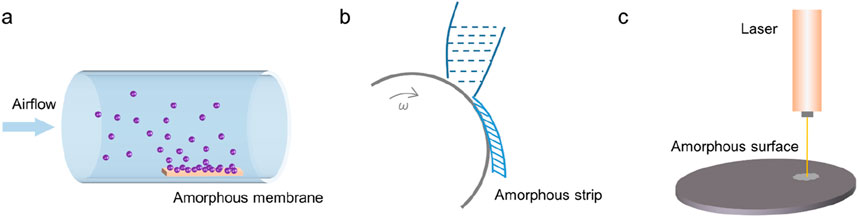
Figure 5. Three kinds of amorphous methods. (a) Physical or chemical vapor deposition. (b) Melt quenching. (c) Laser radiation.
The methods introduced above have the characteristics of high equipment requirements, complex systems, and energy accumulation, and are generally used in the preparation of amorphous materials in high-tech fields such as national defense and electronic devices. The hydrothermal method, sol-gel method, and electrolysis method commonly used in the field of chemical preparation have unique advantages in the preparation of nanomaterials, which have mild conditions and low equipment requirements. Therefore, developing chemical preparation methods for amorphous nanomaterials has great prospects.
3 Research progress of AMNMs
Micro-nano-scale amorphous materials are an important part of the study of amorphous solids. As mentioned above, when the size of the material is reduced to the nanometer level, under the action of nano-effects such as surface effect, small-size effect, dielectric effect, macroscopic quantum tunneling effect, nano-materials show peculiar properties, which present important application value in the semiconductor materials, functional coating materials, permanent magnet materials and other high-tech fields (Kang et al., 2023). In addition to nano-effects, compared with crystalline nanomaterials, amorphous nanomaterials also exhibit a series of unique properties, which have attracted extensive attention from scholars at home and abroad. In recent years, the controllable synthesis and preparation of amorphous micro-nanomaterials has made great progress (Huang et al., 2023; Guo et al., 2024; Cao et al., 2024). This section reviews the current construction and synthesis processes of various amorphous micro-nano materials on the basis of summarizing the structural characteristics of amorphous micro-nano materials, and puts forward the current challenges and perspectives.
3.1 Structural features
Similar to the structural units of crystalline nanomaterials, the structural units of amorphous nanomaterials can also be classified according to the dimension of nanometer size, which can be specifically divided into zero-dimensional, one-dimensional and two-dimensional structural units (Guo et al., 2024; Moseson and Taylor, 2023; Lan et al., 2024; Wang et al., 2024). Usually, in practical applications, a single structural unit is either self-grown on the substrate material, or loaded on the substrate material after synthesis, or the same/different structural nano-units are self-assembled to form a multi-level macroscopic composite material. Common substrate materials include conductive glass, carbon material, metal sheet, metal foam, etc., which generally have the characteristics of high conductivity and good mechanical properties. In general, amorphous micro-nano materials present the following characteristics in structure.
(1) Amorphous morphology is more likely to appear. Due to the lack of specification of lattice orientation inside amorphous materials, the growth process after nucleation often evolves irregular morphologies, such as nanoparticles or irregular nanosheets. This feature increases the difficulty of synthesizing amorphous nanostructures with specific morphology.
(2) Different components are evenly distributed. Due to the chaotic arrangement of particles inside the amorphous material, there is no segregation, aggregation or agglomeration among different components, showing good uniform dispersion. This enables amorphous micro-nano materials to take full advantage of the unique properties of different components; different components that are often uniformly dispersed can also produce coupling or synergistic effects to further enhance their physical or chemical properties.
(3) Morphological evolution is more likely to occur. When the environment of the amorphous material changes, such as temperature rise, electric field or magnetic field changes, the internal metastable disordered structure may evolve and produce a structural phase change, showing that the geometric structure of the amorphous material evolves. Morphological evolution that occurs during service may lead to structural or performance failures, which have adverse effects on the long-term stability of amorphous micro-nano materials.
3.2 Single-scale structure
The single-scale structure of amorphous nanomaterials refers to the structure composed of one nanostructure unit. The single-scale structure has a single composition, which is more intuitive when studying the structure-activity relationship of materials, and obtaining a single-scale structure is the basis for subsequent construction of multi-scale micro-nano structures, so it has been widely studied.
(1) Zero-dimensional amorphous. Zero-dimensional amorphous nanomaterials include atomic clusters, nanoparticles, nanoflowers, nano-polyhedral, nanocages, and various core-shell structures. Generally, single-atom and high-entropy alloys can also be regarded as amorphous nanomaterials, which are also classified as zero-dimensional amorphous nanomaterials here. Figure 6 shows several common zero-dimensional amorphous nanostructures.
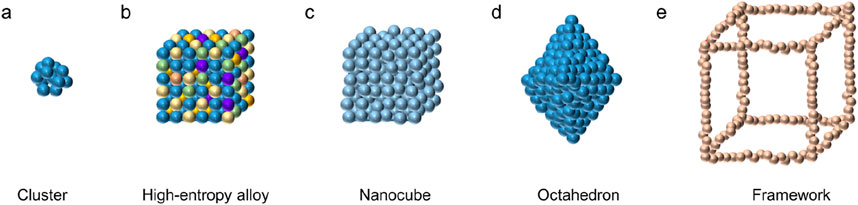
Figure 6. Zero-dimensional amorphous examples inlcuding cluster (a), high-entropy alloy (b), nanocube (c), nano-octahedron (d), and framework (e).
Clusters are generally aggregates with a particle size of less than 1 nm and composed of a dozen to more than a hundred atoms, representing the state of matter in the initial stage of nucleation. Generally, nucleation particles show a strong tendency of close packing and crystallographic anisotropy when forming clusters (Belyakova and Slovokhotov, 2003). According to the amorphous growth theory, when the atoms in the nucleation stage are too late to rearrange and the growth process of the nuclei is hindered, amorphous clusters can be formed (Gebauer and Cölfen, 2011). For example, pre-nucleated clusters in biomineralization can aggregate with each other to form amorphous nuclei or induce the formation of crystals and form crystal-amorphous interfaces at appropriate solute concentrations (Mann et al., 1993). Through experimental means, the number of atoms constituting the cluster is further reduced, and diatoms or even single atoms will be formed. In single-atom to atomic-cluster structures, the huge specific surface area enables ultra-high reactivity.
Nanoparticles generally refer to microparticles with nanometer scale in three dimensions of space, whose particle size is larger than atomic clusters, and usually show irregular shapes. The preparation of amorphous nanoparticles can be controlled by controlling the growth process in the initial growth stage after nucleation. For example, high-entropy alloy nanoparticles with up to five or more constituent elements can be obtained by rapid cooling, and the particles exhibit strong amorphous-forming ability. Moreover, under the action of high-entropy effect and “cocktail” effect (Yin and Xu, 2018), high-entropy alloy nanoparticles often exhibit unexpected functional properties, including near-zero thermal expansion coefficient and simultaneous realization of ultra-high strength and super-toughness.
Amorphous nano-polyhedral in nanoparticles exhibit crystal-like features such as cubes, octahedrons, and tetra-hexahedrons in appearance, but the arrangement of atoms inside them is chaotic. Because amorphous generally exhibits an amorphous state, it is difficult to prepare amorphous nanomaterials with regular morphology. Corresponding amorphous nano-polyhedral frameworks can sometimes be obtained by etching methods.
The core-shell structure usually shows shell and core shapes such as sphere, cube or octahedron. Generally, one-dimensional multilayer nanostructures are called nanocables, which are similar in structure and will not be described here. Nanoparticles with a core-shell structure can have an amorphous shell to enhance their mechanical properties while protecting the performance of the core from being affected. The core-shell structure has many applications in biomedical fields such as drug delivery and cell labeling.
(2) One-dimensional amorphous. According to the aspect ratio and internal structure, one-dimensional nanomaterials can usually be divided into nanowires, nanorods, nanotubes, etc. Generally, one-dimensional materials with a length greater than 1 μm are called nanowires, while those with a length of less than 1 μm are called nanorods (Figure 7a). The discovery of carbon nanotubes by Iijima et al. in 1991 led to the research of one-dimensional nanomaterials. One-dimensional amorphous nanomaterials require the growth of an amorphous structure in one dimension, while the growth of the other two dimensions is restricted, so the synthesis is more difficult. Due to their good electrical conductivity and flexibility, one-dimensional amorphous nanomaterials have important application prospects in the fields of nanodevices, integrated circuits, and electrochemical energy storage.
(3) Two-dimensional amorphous. Among various amorphous nanomaterials, the research on 2D amorphous materials is the most extensive and in-depth, mainly because the amorphous growth mode of amorphous materials makes it easier to obtain 2D sheet-like morphology (Figure 7b). Two-dimensional amorphous materials that have been reported so far include transition metal hydroxides, noble metal oxides, layered double hydroxides (LDHs), transition metal carbides, and transition metal chalcogenides. Usually, the crystal structure of the nanosheets obtained by exfoliating the crystals by the exfoliation method may be destroyed and become amorphized. Amorphous two-dimensional materials, especially ultra-thin two-dimensional amorphous materials with a large number of defects and active sites on the surface can be used as highly active catalysts, and can also provide supporting substrates for zero-dimensional or one-dimensional nanomaterials, so they have important application value.
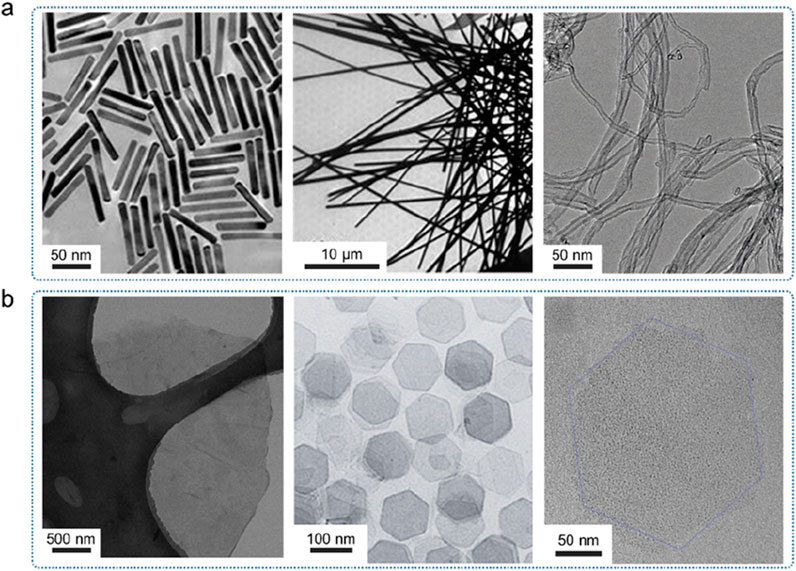
Figure 7. Electron microscopy images of one-dimensional and two-dimensional materials. (a) One-dimensional materials, from left to right are nanorods, nanowires and nanotubes. Adapted with permission from ref. Feng et al. (2016). Copyright 2018. (b) Two-dimensional materials, from left to right are graphene, nickel hydroxide and their enlarged images. Adapted with permission from ref. Qiao et al. (2020). Copyright 2016, WILEY-VCH Verlag GmbH and Co. KGaA, Weinheim.
The controllable synthesis of amorphous nanomaterials that tend to form amorphous forms into micro-nanomaterials with zero-dimensional, one-dimensional or two-dimensional morphology not only broadens the application range of amorphous micro-nanomaterials, but also paves the way for the construction of subsequent multi-level composite structures and the macrostructure.
3.3 Multiscale composite structure
In a broad sense, multiscale composite structures include not only arrays, honeycombs, networks, or frameworks composed of single nano-units through directional or disordered assembly, but also two or more nano-units (including crystalline and amorphous, organic and inorganic, metal and non-metal, etc.) to participate in the composite structure formed by the joint assembly (Figures 8a–c).
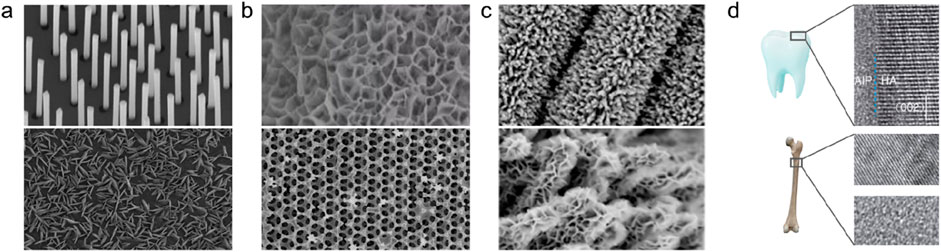
Figure 8. Composite structures formed by the nano-units. (a) Nanowire and nanosheet arrays on a flat substrate. Adapted with permission from ref. Spurgeon et al. (2021). Copyright 2021, Springer Nature. (b) Self-standing nano-frameworks. Adapted with permission from ref. Cockayne (2007). Copyright 2012, Elsevier Inc. (c) Nanosheet arrays on one-dimensional substrates. Adapted with permission from ref. Ye et al. (2015). Copyright 2020, Elsevier Inc. (d) Nano-interfaces of natural materials. Adapted with permission from ref. Li et al. (2011). Copyright 2021, Multidisciplinary Digital Publishing Institute.
Multiscale composite materials not only have the characteristics of their nano-units, but also may produce synergistic effects or coupling responses between nano-units. Practice has proved that multi-level composite nanomaterials can achieve the integration of various target properties, such as high intrinsic activity and high number of active sites in catalysts, high strength and light weight in mechanical materials, etc. In addition, the multi-level structure in high-performance biomaterials such as carapace, bone, and tooth enamel after natural selection also proves the excellent comprehensive performance of the composite structure (Figure 8d).
(1) Amorphous nanoarray. Amorphous nanoarrays include nanowire/tube arrays and nanosheet arrays. During the preparation process, in order to obtain a larger specific surface area, verticality and better integration with the substrate, it is often necessary to precisely control the size and arrangement of the nano-units.
The types of nanowire arrays that have been reported so far include zinc oxide, aluminum oxide, carbon nanotubes, indium arsenide, gallium nitride and other nanowires or nanotubes grown vertically on the substrates. The substrate materials used are generally screened according to the use scenarios considering factors such as specific surface area, electrical conductivity, mechanical properties, etc., usually using single crystal silicon, ITO glass, metal sheets, etc. For example, researchers used nickel foam as a substrate to control and prepare iron oxyhydroxide nanotube arrays by electrochemical deposition (Figure 9) (Feng et al., 2016). The layer of cobalt ions in the nanotube hierarchical structure provides high electrical conductivity. In addition, by pre-patterning the substrate material by means of photolithography, a nanowire array with a specific pattern can be obtained, which is particularly important in the development and application of micro-nano devices.
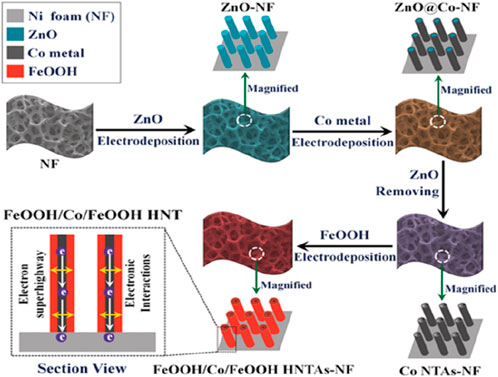
Figure 9. The preparation of a nanowire arrays. Adapted with permission from ref. Feng et al. (2016). Copyright 2016, WILEY-VCH Verlag GmbH and Co. KGaA, Weinheim.
The research of nanosheet arrays mainly focuses on obtaining high specific surface area and high active-mass loading through arraying, because the free nanosheets will stack and reduce the specific surface area in actual use. In addition to the high specific surface area, the nanosheet array also has high stability, which can ensure that the structure does not collapse or fall off under service conditions. Nanowires can also be mixed in the nanosheet array to form a composite array, or conversely, nanosheets can be composited in the nanowire array to form a multilevel composite structure. For example, researchers obtained a multi-level vertical nanosheet array grown on a carbon fiber substrate through two CVD, electrodeposition, and phosphating treatments in sequence (Figure 10) (Ji et al., 2021).
(2) Self-assembly of amorphous nanostructures. In the process of manufacturing new materials with multi-level structures, self-assembly often plays a major role. Based on the primary structural unit, self-assembly is guided by some molecular or organic templates, and the hierarchical management from nano-scale structure specification to macro-scale management occurs, thereby regulating the performance and application of materials. Common forces driving nanoscale self-assembly include van der Waals forces, electrostatic interactions, hydrogen bonds, entropy effects, surface tension, and solvent interactions (Bigioni et al., 2006; Boles et al., 2016). Self-assembled nano-units include zero-dimensional nanoparticles and one-dimensional nanowires or nanotubes (Wei et al., 2002). Composite materials obtained by self-assembly can range from simple single-layer films to materials with complex patterns or structures (Figure 11). More classic cases come from biological macromolecules such as spider silk, silk, etc., as well as biological shells and bones with complex shapes in biological self-assembly.
(3) Crystal-amorphous composite structure. Crystalline nanostructures have good mechanical properties, but lack of plasticity. Amorphous nanostructures have good plasticity and reactivity. Combining the two in a composite structure can make the material have both crystalline and amorphous properties. For example, the nanocrystalline particles can be uniformly dispersed on the amorphous substrate through the amorphous crystallization method, thereby enhancing the strength and ductility of the material. The amorphous nanoalloy with an amorphous surface exhibits greater plastic rheology and strain hardening, which can provide high strength and high plasticity, thereby avoiding “catastrophic failure” (Wu et al., 2017). For another example, Guo et al. introduced the rigid MnO2 crystal skeleton into the flexible amorphous MnO2 ultra-thin nanosheets to form a “bionic leaf” composite structure, which improved the mechanical stability of the structure, and the amorphous structure promoted the electron transport, and it also has excellent cycle stability as a lithium-ion battery anode material (Jia et al., 2020). It is worth mention that amorphous polyamorphs were reported through an in situ X-ray diffraction observation. Unlike crystalline polymorphism, which arises from rearrangements of atoms in a periodic lattice, polyamorphic transitions in glasses involve abrupt changes in short-to medium-range atomic configurations, which has implications for understanding the structure of amorphous materials (Sheng et al., 2007).

Figure 10. The preparation of a multi-level vertical nanosheet arrays. Adapted with permission from ref. Ji et al. (2021). Copyright 2021, Springer Nature.
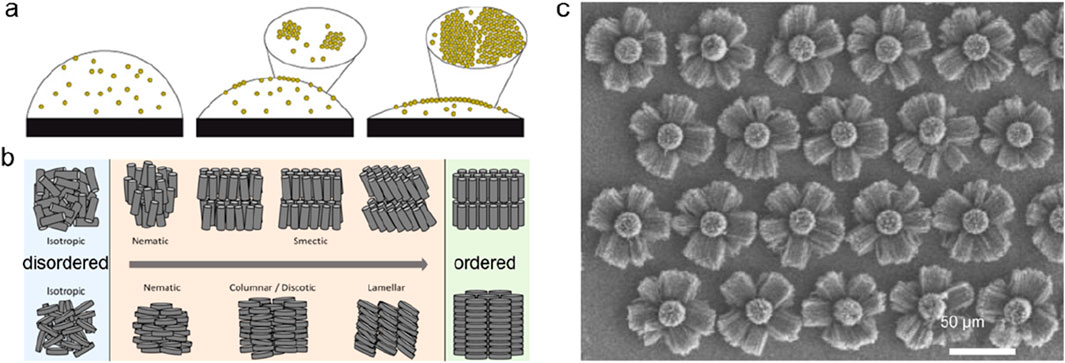
Figure 11. Self-assembled structures. (a) Simple single-layer films assembled by nanoparticles. Adapted with permission from ref. Ozden and Morley (2021). Copyright 2006, Springer Nature. (b) Structures with complex assembled patterns. Adapted with permission from ref. Kang et al. (2023). Copyright 2016, American Chemical Society. (c) Nano-flowers assembled by carbon nanotubes. Adapted with permission from ref. Wei et al. (2002). Copyright 2002, Springer Nature.
3.4 Construction process
The artificial design and construction of substances with structural units of specific shapes and the assembly of macroscopic materials have a profound impact on the practical application of nanotechnology. At present, the synthesis method of amorphous micro-nano materials is relatively simple, and there is a lack of universal synthesis process. For a specific structure, there are fewer optional synthesis methods and control means. In general, the guiding ideology of the synthesis method of amorphous micro-nano materials can be divided into two categories: “top-down” method and “bottom-up” method (Figure 12) (Yu et al., 2013; Abid et al., 2022). The top-down method usually uses laser lithography, mechanical grinding, etching, stripping, electrospinning and other means to process bulk materials or precursor materials to obtain amorphous micro-nano materials with target morphology; while the bottom-up method is usually to assemble and grow microscopic particle units such as atoms, molecules or nanoparticles into an amorphous structure under the action of chemical bonds or external driving forces. Commonly used methods include chemical vapor deposition, hydrothermal/solvothermal method, electrochemical, etc. And the comparison of approaches for amorphous construction is listed in Table 1.
(1) Chemical vapor deposition. CVD technology is mainly used in the preparation of amorphous thin films and is widely used in the semiconductor industry. For example, the amorphous silicon thin film in the amorphous silicon thin film solar cell can be prepared by ion-enhanced CVD (PECVD) technology. In addition, borosilicate glass, silicon oxide, silicon nitride, titanium dioxide and other amorphous thin film materials can also be prepared by CVD technology (Carenco et al., 2013). For example, the deposition of large-area transition metal dichalcogenides (TMDs, such as MoS2) on amorphous SiO2 substrates has been extensively studied (Figures 13A,B) (Ji et al., 2015; van der Zande et al., 2013; Najmaei et al., 2013; Sarkar et al., 2015). Technologies with similar principles to CVD also include magnetron sputtering, atomic layer deposition, physical vapor deposition, epitaxial growth, etc. Most of the amorphous nanomaterials prepared by these methods have the same characteristics.
(2) Solution synthesis methods. Among the synthesis methods of nanomaterials, the synthesis technology using solution system is the most widely studied, which is due to its characteristics of simple operation, low cost, easy control of the process, and easy scale-up. Taking the hydrothermal/solvothermal method as an example, researchers have developed a variety of synthetic routes, such as adding templates, redox agents, complexing agents, and directing agents to the system (Fei et al., 2021). Through the hydrothermal method, nano-amorphous materials with different morphologies can be prepared, and nano-array composite materials can also be grown on the substrate (Figures 13c–f). In the field of hydrothermal synthesis of inorganic nanomaterials, Li et al. has done a lot of work and achieved many creative results (Ji et al., 2020; Tong et al., 2019; Jiang et al., 2020).
(3) Electrochemical methods. The conversion of nano-precursors into amorphous nanostructures using electrochemical methods is a relatively novel approach. Electrochemical methods usually include electrochemical deposition, electrochemical replacement, and in situ electrochemical conversion (Figure 14) (Li et al., 2021; Shen et al., 2022). For example, aqueous manganese phosphate can be deposited on a graphite substrate by electrochemical deposition, which is subsequently transformed into amorphous manganese phosphate with a highly porous structure (Yang et al., 2021). The research group of Jin successfully prepared amorphous PdM alloy nanowires (a-PdM NWs, M = Fe, Co, Ni, Cu) at low temperature through a general electro-displacement assisted strategy (Wang et al., 2021). Specifically, this method uses ligand adsorption to generate amorphous nuclei of non-noble metal materials, and introduces palladium atoms through replacement reactions at low temperature, thereby realizing the preparation of PdM amorphous alloy nanowires.
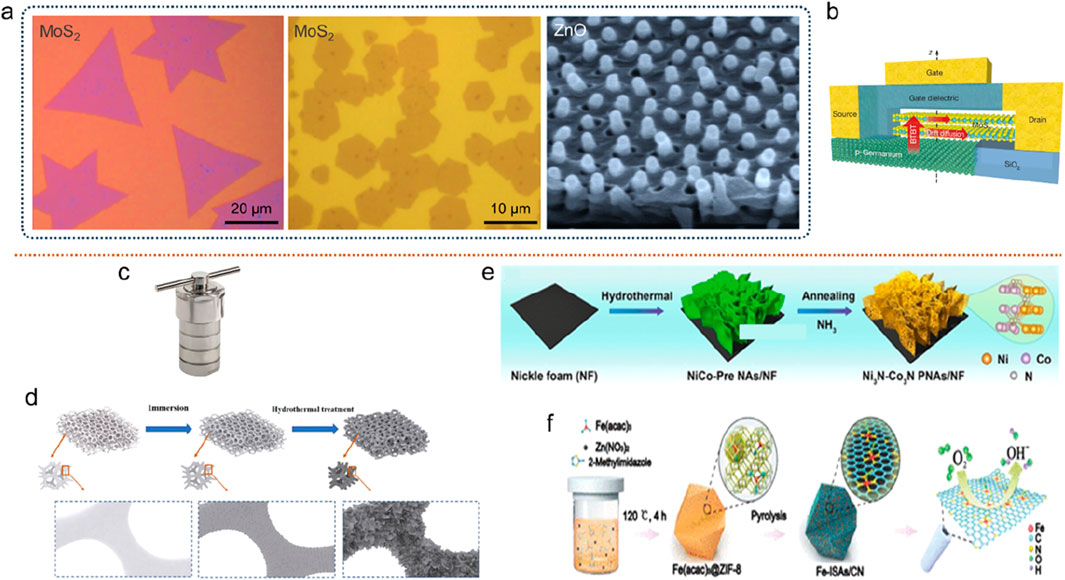
Figure 13. Preparation methods of AMNMs. (a,b) Chemical vapor deposition. Adapted with permission from ref. Gebauer and Cölfen (2011), Mann et al. (1993), Yin and Xu (2018). Copyright 2013, 2015, Springer Nature. (c–f) Solution synthesis. Adapted with permission from ref. Ji et al. (2021). Copyright 2013, 2020, American Chemical Society.
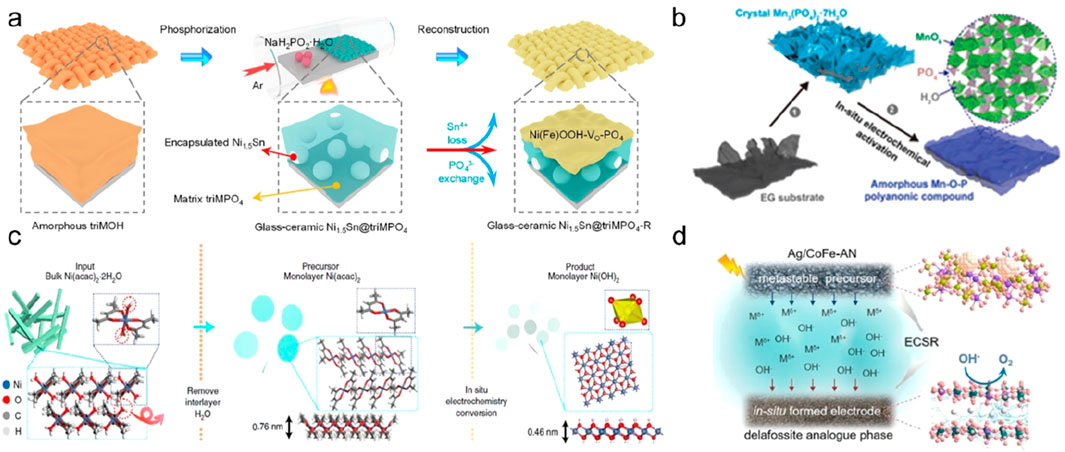
Figure 14. Electrochemical methods for AMNMs. (a) Electrochemical deposition. Adapted with permission from ref. Wei et al. (2002). Copyright 2021, WILEY-VCH Verlag GmbH and Co. KGaA, Weinheim. (b–d) In situelectrochemical activation. Adapted with permission from ref. Sheng et al. (2007), Yu et al. (2013), Abid et al. (2022). Copyright 2021, American Chemical Society, Springer Nature, and Elsevier Inc.
The research group of Guo reported a method for in situ conversion of single-layer nickel acetylacetonate (Ni(acac)2) into amorphous Ni(OH)2 single-layer nanosheets directly on the electrode (Kang et al., 2021), and passed Co doping can further tune the electronic structure of the amorphous single-layer nanosheets, resulting in ultrahigh oxygen evolution reactivity. The research group has carried out a series of related work and accumulated rich experience. It has rationally manipulated and utilized the preparation of amorphous nanomaterials using electrochemical in situ reconstitution, and successfully applied the synthesized amorphous nanomaterials to electrocatalysis (Liu and Guo, 2021; Kang et al., 2022; Liu et al., 2020).
(4) Electrospinning method. Electrospinning is an efficient method for preparing one-dimensional amorphous nanomaterials (with a diameter of tens to hundreds of nanometers) and their composite films (Xue et al., 2019). The preparation process is usually under the action of an electric field, with the precursor solution or melt becoming a “Taylor cone” at the needle and expanding into a fiber filament (Figure 15a). The viscosity, conductivity, surface tension and other factors of the solution have a great influence on the quality of spinning. Electrospinning can also be used to make coaxial fiber “cables” by using coaxial needles. For example, Yu et al. reported the design of a noble metal-free Bi4V2O11/CeO2 nanostructure through a spinneret electrospinning process and subsequent calcination. The structure has a nanocrystalline inner core of CeO2 and an amorphous Bi4V2O11 surface (Lv et al., 2018).
(5) Template method. Template methods can be divided into soft template methods and hard template methods. Common soft templates include molecules such as surfactants and polymers, while hard templates include porous alumina, molecular sieves, carbon nanotubes, graphene, etc. (Figure 15b) (Wang et al., 2005; Doustkhah et al., 2021; Kaneti et al., 2021). Nanostructures such as nanocages and polyhedral frameworks can be obtained by etching based on the morphology of the hard template. Guo et al. constructed a universal sacrificial template method (Figure 15c) (Nai et al., 2013): with the help of soft and hard acid-base theory, the sacrificial template was coordinated-etched, and the hollow ammonium oxide of transition metal hydroxide was successfully synthesized. The team then developed a photolithography method to prepare amorphous NiFe-based oxide and hydroxide nanocages (Cai et al., 2019). After the photolithography reaction, the high-valence Ni acts as an active component and plays an important role in the water oxidation reaction of the amorphous nanocages. Graphene oxide is often used as a template for the growth of two-dimensional ultrathin amorphous nanomaterials because of its large π bonds on the surface that can electrostatically adsorb cations (Zhao et al., 2016a; Zhao et al., 2019).
(6) Pyrolysis method. Pyrolysis has good results in the preparation of amorphous nanoparticles and nanosheets. For example, in the preparation of high-entropy alloy nanoparticles, the pulsed carbon thermal shock method used by Hu et al. can thermally decompose the precursor into amorphous nanoparticles (Figure 16a), the types of metal elements can be as high as eight (this research group has reported high-entropy alloy nanoparticles with up to 15 elements) (Yao et al., 2022; Yao et al., 2021). For another example, the research group of Li reported a general method for preparing ultra-thin nanosheets of amorphous noble metals by annealing precursors, and synthesized a variety of amorphous nanosheets of noble metal oxides (Figure 16b) (Wu et al., 2019).
(7) Stripping method. The exfoliation method refers to the use of external effects such as mechanical force, intercalation solvent or ions to detach the layered constituent units on the layered bulk material layer by layer and disperse them into the solution, so as to obtain ultra-thin to single-layer two-dimensional nanomaterials (Figure 16c) (Cai et al., 2018; Anasori et al., 2017). The most classic case of this type of method is the preparation of graphene. In 2004, Geim et al. first used tape to peel off highly oriented pyrolytic graphite to obtain single-layer graphene, and thus won the 2010 Nobel Prize in Physics. Two-dimensional materials obtained by the exfoliation method also include titanium carbide, LDHs, MoS2, etc. Commonly used intercalants in electrochemical exfoliation or solvent exfoliation include alkali metal ions (Li+, Na+, K+), organic cations, methylpyrrolidone (NMP), dimethylformamide (DMF), etc. (Ambrosi et al., 2015). During the peeling process, ultrasound can also be used to strengthen the assistance basic concepts.
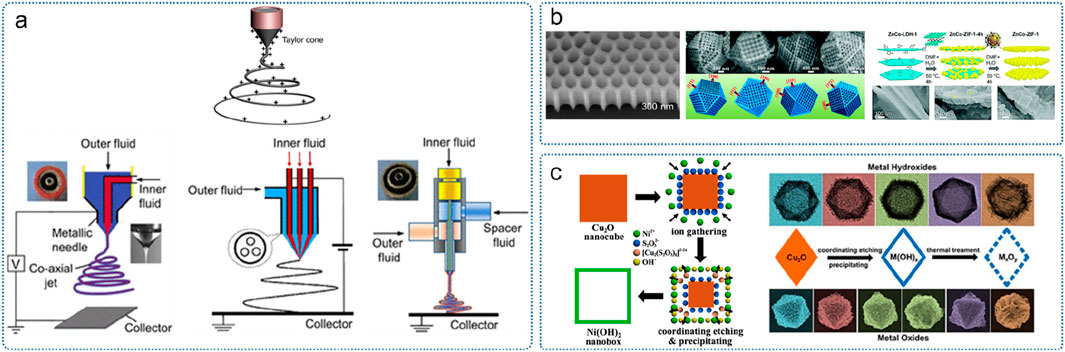
Figure 15. Electrospinning and template methods. (a) Traditional operation modes of electrospinning. Adapted with permission from ref. van der Zande et al. (2013). Copyright 2019, American Chemical Society. (b) Hard templates. Adapted with permission from ref. Sarkar et al. (2015), Toh et al. (2020), Zhang et al. (2015). Copyright 2005, Springer Nature; Copyright 2021, The Royal Society of Chemistry; Copyright 2021, Elsevier Inc. (c) Sacrificial templates. Adapted with permission from ref. Ahmed et al. (2017). Copyright 2013, American Chemical Society.
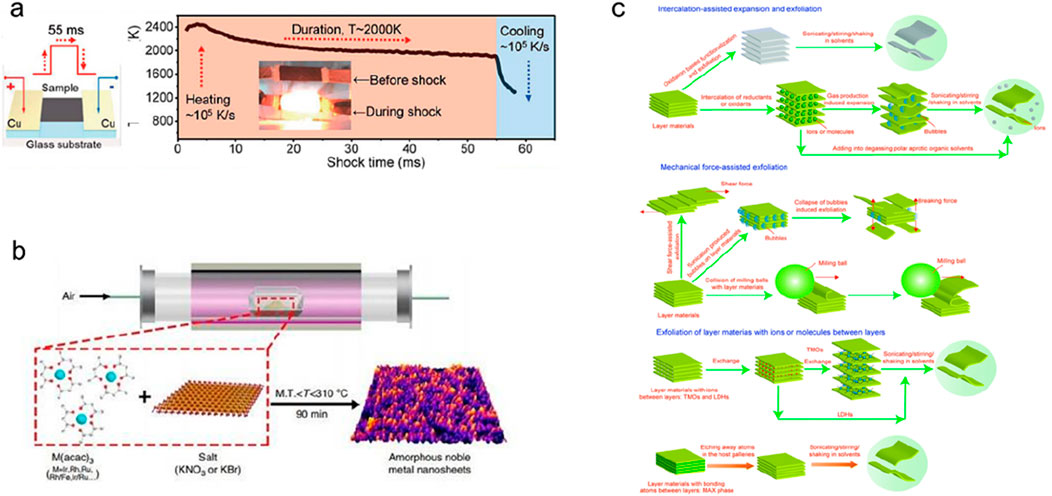
Figure 16. Pyrolysis and stripping methods. (a) Pulsed carbon thermal shock method. Adapted with permission from ref. Gao et al. (2017). Copyright 2022, American Association for the Advancement of Science. (b) Annealing and decomposition. Adapted with permission from ref. Jiang et al. (2018). Copyright 2019, Springer Nature. (c) Traditional exfoliation processes. Adapted with permission from ref. Fei et al. (2021). Copyright 2017, Springer Nature.
4 Applications of AMNMs
Nanotechnology has penetrated into the national economy and high-tech fields for decades, and has already fully demonstrated its very broad application prospects. As a unique branch of the nanomaterial family, amorphous micro-nanomaterials have shown great application potential in many fields such as energy, electronics, chemical industry, medicine, and national defense. Under the guidance of national strategies, scientists from all over the world are vigorously promoting the construction research and industrial application of amorphous micro-nano materials. In the following, the research status of amorphous micro-nano materials in the fields of electrocatalysis, energy storage and conversion, biomedicine, aerospace and other fields is summarized through a brief review of the literature.
4.1 Lightweight and high-strength materials (LVHS)
Lightweight and high-strength materials (LVHS) have the characteristics of light weight and high strength, and have important applications in various fields of modern industry. At present, the design of lightweight and high-strength micro-nano materials are mainly based on application requirements, and through the inspiration of natural organisms, multi-scale structural assembly is carried out to construct structural materials with advanced properties. Amorphous materials, as biomineralization precursors, are important links in the development of various biological tissues such as bone, pearl, and tooth enamel. Therefore, amorphous micro-nano materials are of great significance as bionic lightweight high-strength materials.
For example, through a simple liquid-phase reaction, Guo et al. innovatively realized the in situ growth of ultra-thin amorphous alumina nanosheets (AA) on the surface of graphene oxide (GO) (Zhao et al., 2016b); with this GO@ AA composite nanosheets are the basic structural unit, and a self-supporting amorphous alumina-reinforced GO-based ternary layered composite film is prepared by vacuum-assisted assembly technology; due to the addition of amorphous alumina nanosheets that perfectly fit the layer-by-layer stack structure, the composite film achieved a simultaneous increase in strength (305 MPa) and toughness (8.2 MJ/m3). In terms of tooth restoration, the team has developed a crystal-amorphous interface construction technology and created a hard tissue in situ growth repair strategy, uniformly growing an amorphous zirconia ceramic repair layer on the damaged enamel surface, successfully solving the traditional method problems such as low efficiency and weak interface connections (Wei et al., 2020).
Subsequently, in 2022, the research team reported that using natural enamel as a construction model, the enamel-like composite material with the structure most similar to enamel was prepared for the first time through the macro-synthesis and controllable assembly strategy of nanostructure units. This composite simultaneously realizes various contradictory mechanical properties such as high hardness, high elasticity, high strength, and high toughness (Zhao H. et al., 2022). Specifically, this strategy first designed and synthesized hydroxyapatite nanowires with a microscopic size close to that of tooth enamel pillars by hydrothermal method; by rationally regulating the growth and nucleation of materials, the amorphous zirconia ceramic layer was realized. The uniform growth on the surface of the wire mimics the inorganic amorphous interstitial layer of natural tooth enamel (Figure 17). It is worth noting that the amorphous-coated HA nanowires exhibit higher strength and toughness, which can be attributed to the tightly connected amorphous/crystalline interface and the excellent mechanical dissipation ability of amorphous materials.
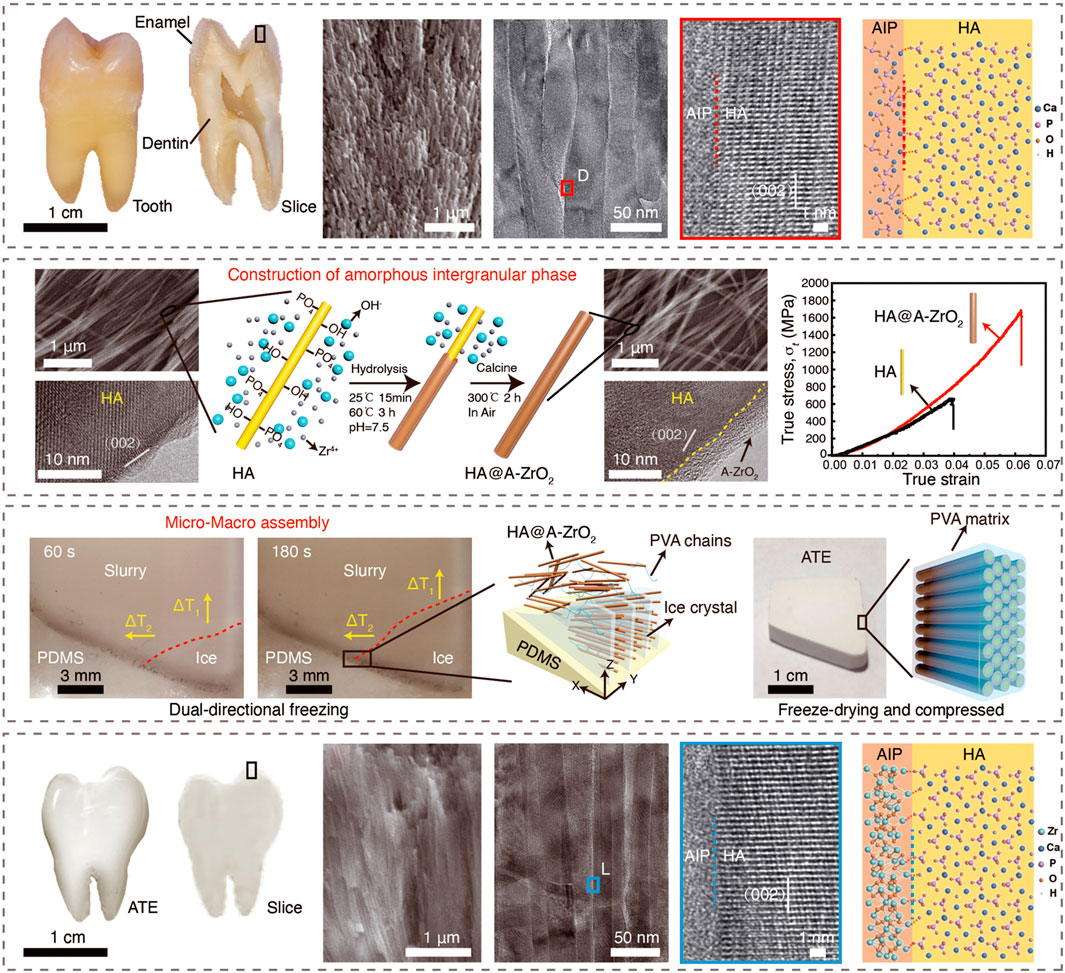
Figure 17. The structure comparation of natural tooth enamel and synthetic artificial tooth enamel. Adapted with permission from ref. Zhao H. et al. (2022). Copyright 2022, American Association for the Advancement of Science.
4.2 Electronics and energy storage
Amorphous micro-nano materials also have broad application prospects in the field of energy storage and conversion. For example, based on the structural characteristics of two-dimensional amorphous materials, including sufficient free space to release structural stress and volume change, fully exposed active sites can improve atomic utilization. For example, ultrathin amorphous nickel hydroxide nanosheets have been applied to supercapacitors (Kang et al., 2022). The results show that the prepared single-layer nickel hydroxide can realize two electron transfers, so that most of the Ni ions are converted into Ni4+ during the charging process, showing a redox capacity of 576 mAh/g, which is close to the theoretical capacity of the single-electron process. Twice (Figure 18a). Yu et al. reported the preparation of amorphous phosphorus-based anode materials by high-energy ball milling for sodium-ion batteries (Chen et al., 2021). In this structure, the volume expansion of amorphous P is isotropic, and the structural stability is improved. For another example, Guo et al. reported the amorphous-crystalline composite two-dimensional structure (bionic vein-mesophyll structure) MnO2 and used it in the research of lithium-ion battery anode materials and capacitor energy storage. The material showed excellent performance.
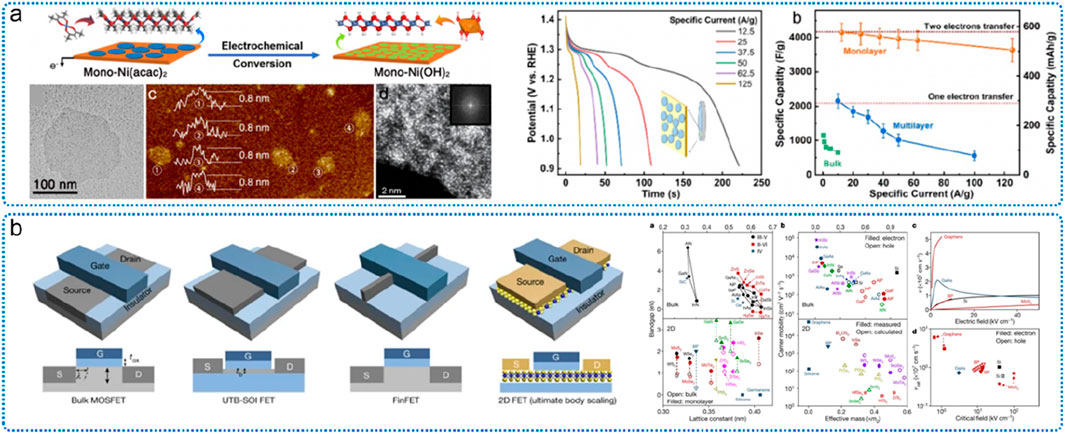
Figure 18. Two-dimensional ultra-thin amorphous nickel hydroxide and its energy storage application (a) and the application of two-dimensional materials in transistor (b). Adapted with permission from ref. Gao et al. (2017). Copyright 2022, American Association for the Advancement of Science.
In addition, in the field of electronics, ultra-thin two-dimensional rare earth amorphous nanomaterials can integrate the unique optical and magnetic behaviors of rare earth elements into the layer, and then have unique properties in terms of luminescence, magnetic moment, magnetic susceptibility, electronic and magnetic relaxation time, etc., Nature. For example, the magnetic moments of R2Ti2O7 and R2Zr2O7 (R = Gd, Dy, Tb) materials are affected by the crystal field around the amorphous rare earth ions. Compared with the strong interaction between rare earth ions in crystalline materials, materials containing amorphous components have a more stable structural state (Kuznetsov et al., 2020). For another example, in the new generation of electronic computers, field effect transistors (FETs) constructed by atomically thin single-layer or several-layer two-dimensional semiconductors such as MoS2, MoSe2, GaAs, etc., are considered to be a new channel to break through the limit of “Moore’s Law” material (Figure 18b) (Liu et al., 2021). The research on the electronic structure and physical and chemical properties of ultra-thin amorphous materials will help to promote the understanding of electron transport and other properties in the working process of FETs.
4.3 Medicine
Amorphous micro-nano materials also have broad application space in the field of biomedicine. For example, due to problems such as poor pharmacokinetics, poor biodistribution, and serious side effects of existing chemotherapy drugs, their therapeutic effects still cannot meet clinical needs. Precision medicine based on nanomaterials has shown some potential applications in tumor treatment, such as targeted drug delivery, photothermal therapy, and photodynamic therapy. Notably, these therapeutic strategies are mainly derived from the physical properties of nanomaterials, including shape, size, optics, and magnetism. Furthermore, they inevitably require external assistance during application, such as lasers, electric or magnetic fields.
First, amorphous nanomaterials can serve as a class of chemotherapy drugs that work independently. Amorphous materials with high activity can induce abundant intracellular reactive oxygen species (ROS), including hydrogen peroxide (H2O2), superoxide anion (O2−), and hydroxyl radicals (OH). Destruction of subcellular organelles and functional biomacromolecules, leading to self-destruction of cancer cells. The reported studies include gold nanoparticles, Ag2S amorphous nanoparticles, etc. (Wang et al., 2019).
Secondly, amorphous micro-nanomaterials also have the potential to be widely used in precise targeted drug delivery. For example, amorphous magnetic zinc-zirconium phosphate hydrated nanosheets are used as protein loading efficiency due to their large number of protein interaction sites (Guo et al., 2021). In addition to two-dimensional nanosheet materials, the hollow core-shell structure is considered to be the most potential carrier for targeted drug delivery. For example, Wang et al. has carried out systematic work to prepare up to 8-layer multi-core-multi-shell structures through reasonable nanostructure design. Through the regulation of the composition and structure of the core and shell, a variety of drug releases in a temporal and spatial order can be achieved (Figure 19) (Zhao D. et al., 2022; Zhao et al., 2020; Wang et al., 2022; Zhao et al., 2021).
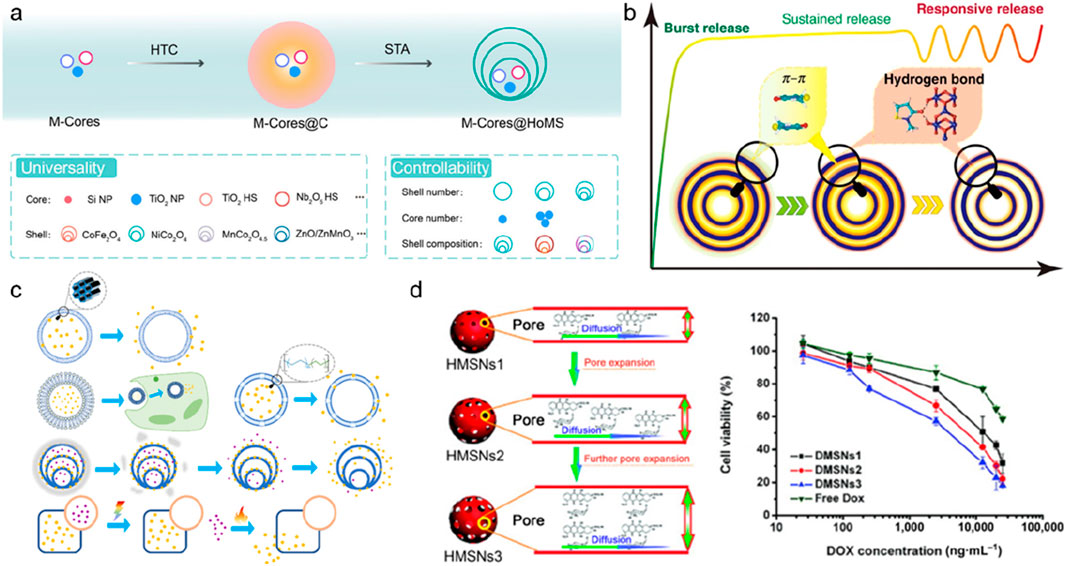
Figure 19. A schematic diagram of the multilayer core-shell structure of micro-nano-materials and their application in targeted drug delivery. (a) The structure and types of nuclear-shell materials. (b) A schematic diagram of the changes in the process of drug release over time. (c) a schematic diagram of hollow mesoporous materials, hollow liposomes and polymer capsules, hollow multi-shell structures, and Janus particles in the release characteristics of different carriers and drug molecules. (d) A representative diagram of controlling the drug release rate by adjusting the pore size to 3.2, 6.4, and 12.6 nm, and the in vitro cytotoxicity of nuclear-shell materials with different pore sizes to test cells.
In addition, amorphous micro-nanomaterials can also be used in medical implants. Most metal medical implants such as prosthetic limbs, artificial joints, vascular stents, etc., are made of stainless steel, cobalt chromium alloy or metal titanium. Although these materials can play a supporting role after being implanted in the human body, they cannot dispel the force generated by the growth of the patient’s body tissue, and prolonged use of implants with poor elasticity may also lead to fatigue fractures. Due to the extraordinary mechanical properties of amorphous alloys, they can adapt to the human body and are very biocompatible. In addition, amorphous materials prepared by 3D printing devices are expected to have significantly improved performance over existing steel or titanium devices (Imai et al., 2020). For example, the use of amorphous micro-nano metal materials to make vascular stents can reduce the size of the support rods to less than 5 μm while ensuring mechanical strength and prolonging the service life. At present, amorphous alloys that are widely studied include titanium alloys, magnesium alloys, cobalt-nickel-based alloys, and zirconium alloys. Researchers have studied the mechanical properties, corrosion resistance, biocompatibility, surface modification, and degradability of materials Numerous studies (Hasiak et al., 2022; Sun et al., 2022).
4.4 Aerospace
Metal materials are closely related to the development of human civilization. Amorphous alloys and high-entropy alloys have shown important strategic value in aerospace and other defense fields. For example, iron-based, cobalt-based and titanium-based amorphous alloys have high strength (several GPa), high toughness (hundreds of MPa/m1/2), high elastic deformation (>2%), and radiation and corrosion resistance. It can serve stably in the space environment. Amorphous coatings with corrosion resistance and wave-absorbing properties can be applied to carrier-based aircraft and stealth aircraft. Amorphous alloys with high specific strength, high elastic limit, and low bulk expansion coefficient can be used in key components of aerospace vehicles such as satellites, space stations, and space probes (Figure 20) (Wang et al., 2006; Amorphous China; George et al., 2019). However, the biggest challenge in the development of amorphous alloys is still the limitation of the synthesis scale, especially many new amorphous alloy systems developed have not been applied on a large scale (Wang, 2022).
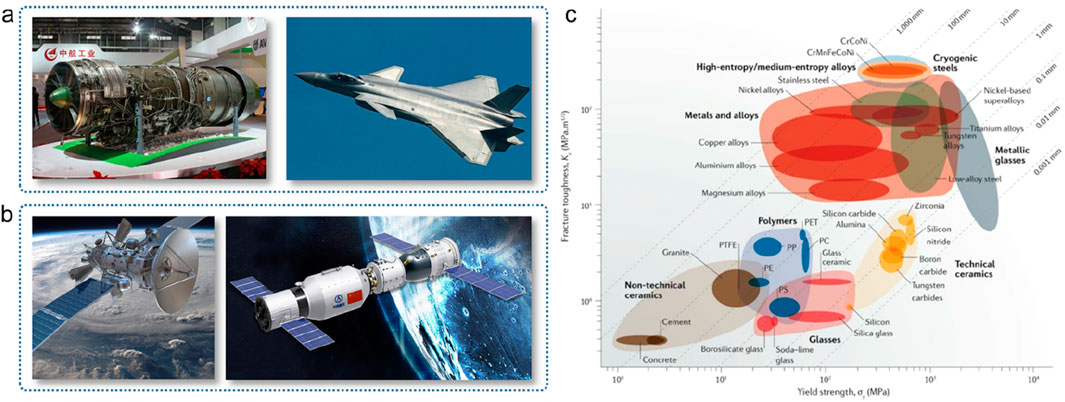
Figure 20. The application prospects of amorphous materials in the fields of aviation (a) and aerospace (b), as well as the comparison of the mechanical properties of high entropy alloys and various mechanical materials in the scale dimension (c).
5 Opportunities and challenges
In recent years, countless researchers have devoted themselves to the study of amorphous micro-nano materials, constructing a wealth of synthesis and preparation methods, and making numerous attempts to develop the application fields of amorphous micro-nano materials. As an emerging material, amorphous micro-nano materials show broad application prospects. The diversity of components in the micro-nano structure and the synergy between different elements contribute to their excellent service performance. For example, in the field of electrocatalysis, by accurately identifying the active sites in amorphous micro-nano materials, the synergistic effect or electronic state between metal ions in the microenvironment can be revealed, thereby improving the electrocatalytic performance of the material. However, it must be acknowledged that the research on amorphous micro-nano materials is still in its infancy and faces several urgent challenges.
Firstly, the controllable synthesis of amorphous micro-nano materials is still in the exploratory stage, and the successful development of many nanostructures has gone through extensive experimental exploration. Considering the structural and combinatorial diversity of micro/nanomaterials, the paradigm for developing novel amorphous micro/nanomaterials should shift from the traditional trial-and-error approach to descriptor-based systematic design. Therefore, it is necessary to combine theoretical prediction with experimental verification to identify the structural characteristics of highly active and stable amorphous micro-nano materials and to find new synthesis processes and development routes for micro-nano materials based on this. Secondly, assembling the synthesized amorphous micro-nano materials into macroscopic materials while maintaining the characteristics and stability of micro-nano materials, especially under harsh working conditions of elevated temperature and high electrolyte concentration, is also a significant challenge. Additionally, the synthesis and macro-integration of amorphous micro-nano materials should be adjusted according to the assembly and operation procedures of the device to meet economic and scalable requirements. Overcoming the mechanism of amorphous formation in science and the practical application of amorphous materials in technology are problems that must be addressed by researchers in this field.
Author contributions
GL: Data curation, Investigation, Writing – original draft. HH: Conceptualization, Supervision, Writing – review and editing.
Funding
The author(s) declare that financial support was received for the research and/or publication of this article. The work was supported by the National Natural Science Foundation of China (52422210, 52373221, 52250119), the National Key R&D Program of China (2020YFA0710403).
Conflict of interest
Author HH was employed by Aerospace Internet of Things Technology Co., Ltd.
Generative AI statement
The author(s) declare that no Generative AI was used in the creation of this manuscript.
Publisher’s note
All claims expressed in this article are solely those of the authors and do not necessarily represent those of their affiliated organizations, or those of the publisher, the editors and the reviewers. Any product that may be evaluated in this article, or claim that may be made by its manufacturer, is not guaranteed or endorsed by the publisher.
References
Abid, N., Khan, A. M., Shujait, S., Chaudhary, K., Ikram, M., Imran, M., et al. (2022). Synthesis of nanomaterials using various top-down and bottom-up approaches, influencing factors, advantages, and disadvantages: a review. Adv. Colloid Interf. Sci. 300, 102597. doi:10.1016/j.cis.2021.102597
Ahmed, S., Ding, X., Bao, N., Bian, P., Zheng, R., Wang, Y., et al. (2017). Inducing high coercivity in MoS2 nanosheets by transition element doping. Chem. Mater. 29, 9066–9074. doi:10.1021/acs.chemmater.7b02593
Ambrosi, A., Sofer, Z., and Pumera, M. (2015). Lithium intercalation compound dramatically influences the electrochemical properties of exfoliated MoS 2. Small 11, 605–612. doi:10.1002/smll.201400401
Amorphous China. Does China’s aviation “heart” need amorphous alloys? Available online at: http://www.bmgchina.com/shownew.aspx?id=134 (Accessed August 29, 2016).
Anasori, B., Lukatskaya, M. R., and Gogotsi, Y. (2017). 2D metal carbides and nitrides (MXenes) for energy storage. Nat. Rev. Mater. 2, 16098–16117. doi:10.1038/natrevmats.2016.98
Axinte, E. (2012). Metallic glasses from “alchemy” to pure science: present and future of design, processing and applications of glassy metals. Mater. Des. 35, 518–556. doi:10.1016/j.matdes.2011.09.028
Baghestani, G., King, H. J., and Hocking, R. K. (2024). The functional role of disordered metal oxides from active catalysis to biological metabolism. Adv. Energy Mater. 14, 2400281. doi:10.1002/aenm.202400281
Belyakova, O. A., and Slovokhotov, Yu. L. (2003). Structures of large transition metal clusters. Russ. Chem. Bull. 52, 2299–2327. doi:10.1023/b:rucb.0000012351.07223.d4
Bigioni, T. P., Lin, X. M., Nguyen, T. T., Corwin, E. I., Witten, T. A., and Jaeger, H. M. (2006). Kinetically driven self assembly of highly ordered nanoparticle monolayers. Nat. Mater. 5, 265–270. doi:10.1038/nmat1611
Boles, M. A., Engel, M., and Talapin, D. V. (2016). Self-assembly of colloidal nanocrystals: from intricate structures to functional materials. Chem. Rev. 116, 11220–11289. doi:10.1021/acs.chemrev.6b00196
Cai, X., Luo, Y., Liu, B., and Cheng, H.-M. (2018). Preparation of 2D material dispersions and their applications. Chem. Soc. Rev. 47, 6224–6266. doi:10.1039/c8cs00254a
Cai, Z., Li, L., Zhang, Y., Yang, Z., Yang, J., Guo, Y., et al. (2019). Amorphous nanocages of Cu-Ni-Fe hydr(oxy)oxide prepared by photocorrosion for highly efficient oxygen evolution. Angew. Chem. Int. Ed. 58, 4189–4194. doi:10.1002/anie.201812601
Cao, M., Li, W., Li, T., Zhu, F., and Wang, X. (2024). Polymetallic amorphous materials: research progress in synthetic strategies and electrocatalytic applications. J. Mater. Chem. A 12, 15541–15557. doi:10.1039/d4ta01418a
Carenco, S., Portehault, D., Boissière, C., Mézailles, N., and Sanchez, C. (2013). Nanoscaled metal borides and phosphides: recent developments and perspectives. Chem. Rev. 113, 7981–8065. doi:10.1021/cr400020d
Chen, B., Yang, Y., Chen, A., Zhang, X., Saddique, J., Tang, M., et al. (2021). Sodium-ion battery anode construction with SnPx crystal domain in amorphous phosphorus matrix. Energy Mater. Adv. 2021, 1–11. doi:10.34133/2021/9795825
Choudhary, K., DeCost, B., Chen, C., Jain, A., Tavazza, F., Cohn, R., et al. (2022). Recent advances and applications of deep learning methods in materials science. NPJ Comput. Mater. 8, 59–26. doi:10.1038/s41524-022-00734-6
Cockayne, D. J. H. (2007). The study of nanovolumes of amorphous materials using electron scattering. Annu. Rev. Mater. Res. 37, 159–187. doi:10.1146/annurev.matsci.35.082803.103337
Doustkhah, E., Hassandoost, R., Khataee, A., Luque, R., and Assadi, M. H. N. (2021). Hard-templated metal–organic frameworks for advanced applications. Chem. Soc. Rev. 50, 2927–2953. doi:10.1039/c9cs00813f
Fei, M., Zhang, R., Li, L., Li, J., Ma, Z., Zhang, K., et al. (2021). Epitaxial growth of MnFe2O4 nanosheets arrays for supercapacitor. Electrochimica Acta 368, 137586. doi:10.1016/j.electacta.2020.137586
Feng, J.-X., Xu, H., Dong, Y., Ye, S., Tong, Y., and Li, G. (2016). FeOOH/Co/FeOOH hybrid nanotube arrays as high-performance electrocatalysts for the oxygen evolution reaction. Angew. Chem. 128, 3758–3762. doi:10.1002/ange.201511447
Feynman, R. P. (1956). There’s plenty of room at the bottom. California: The Annual Meeting of the American Physical Society.
Fu, W., Yang, S., Yang, H., Guo, B., and Huang, Z. (2019). 2D amorphous MoS3 nanosheets with porous network structures for scavenging toxic metal ions from synthetic acid mine drainage. J. Mater. Chem. A 7, 18799–18806. doi:10.1039/c9ta05861c
Gao, K., Guo, X. Q., and Zhang, R. (2016). Structure and formation mechanism of Co-Cu-Pb alloy particles prepared by single-roller rapid solidification method. Mater. Sci. Eng. Powder Metall. 21, 862–869.
Gao, R., Zhang, Q., Soyekwo, F., Lin, C., Lv, R., Qu, Y., et al. (2017). Novel amorphous nickel sulfide@CoS double-shelled polyhedral nanocages for supercapacitor electrode materials with superior electrochemical properties. Electrochim. Acta 237, 94–101. doi:10.1016/j.electacta.2017.03.214
Gebauer, D., and Cölfen, H. (2011). Prenucleation clusters and non-classical nucleation. Nano Today 6, 564–584. doi:10.1016/j.nantod.2011.10.005
George, E. P., Raabe, D., and Ritchie, R. O. (2019). High-entropy alloys. Nat. Rev. Mater. 4, 515–534.
Guo, L. (2021). Amorphous nanomaterials: preparation, characterization and applications. New Jersey: John Wiley & Sons, Inc. doi:10.1002/9783527826360
Guo, Z., Liu, Z., and Tang, R. (2024). Applications of amorphous inorganics as novel functional materials. Mater. Chem. Front. 8, 1703–1730. doi:10.1039/d3qm01263h
Guo, Z.-Y., Zhang, C., Jiao, R. W., Yao, Q. h., and Chen, X. (2021). Construction of metal hydrate-based amorphous magnetic nanosheets for enhanced protein enrichment and immobilization. ACS Appl. Mater. Interfaces 13, 37915–37923. doi:10.1021/acsami.1c10086
Hasiak, M., Sobieszczańska, B., Łaszcz, A., Biały, M., Chęcmanowski, J., Zatoński, T., et al. (2022). Production, mechanical properties and biomedical characterization of ZrTi-based bulk metallic glasses in comparison with 316L stainless steel and Ti6Al4V alloy. Materials 15, 252. doi:10.3390/ma15010252
Huang, L., Cheng, L., Zhang, J., Wu, H., Su, J., et al. (2023). Direct synthesis of ammonia from nitrate on amorphous graphene with near 100% efficiency. Adv. Mater 35, 2211856. doi:10.1002/adma.202211856
Imai, K., Zhou, X., and Liu, X. (2020). Application of Zr and Ti-based bulk metallic glasses for orthopaedic and dental device materials. Metals 10, 203. doi:10.3390/met10020203
Ji, Q., Zhang, Y., Zhang, Y., and Liu, Z. (2015). Chemical vapour deposition of group-VIB metal dichalcogenide monolayers: engineered substrates from amorphous to single crystalline. Chem. Soc. Rev. 44, 2587–2602. doi:10.1039/c4cs00258j
Ji, S., Chen, Y., Wang, X., Zhang, Z., Wang, D., and Li, Y. (2020). Chemical synthesis of single atomic site catalysts. Chem. Rev. 120, 11900–11955. doi:10.1021/acs.chemrev.9b00818
Ji, X., Lin, Y., Zeng, J., Ren, Z., Lin, Z., Mu, Y., et al. (2021). Graphene/MoS2/FeCoNi(OH)x and graphene/MoS2/FeCoNiPx multilayer-stacked vertical nanosheets on carbon fibers for highly efficient overall water splitting. Nat. Commun. 12, 1380. doi:10.1038/s41467-021-21742-y
Jia, B., Chen, W., Luo, J., Yang, Z., Li, L., and Guo, L. (2020). Construction of MnO 2 artificial leaf with atomic thickness as highly stable battery anodes. Adv. Mater. 32, 1906582. doi:10.1002/adma.201906582
Jia, B., Liu, G., Zhang, B., Zheng, J., Yin, K., Lin, J., et al. (2024). General modification strategy on amorphous materials to boost catalytic performance. Adv. Funct. Mater. 34, 2405867. doi:10.1002/adfm.202405867
Jiang, J., Jiang, P., Wang, D., and Li, Y. (2020). The synthetic strategies for single atomic site catalysts based on metal–organic frameworks. Nanoscale 12, 20580–20589. doi:10.1039/d0nr05907b
Jiang, Y., Song, Y., Pan, Z., Meng, Y., Jiang, L., Wu, Z., et al. (2018). Rapid amorphization in metastable CoSeO3·H2O nanosheets for ultrafast lithiation kinetics. ACS Nano 12, 5011–5020. doi:10.1021/acsnano.8b02352
Kaneti, Y. V., Guo, Y., Septiani, N. L. W., Iqbal, M., Jiang, X., Takei, T., et al. (2021). Self-templated fabrication of hierarchical hollow manganese-cobalt phosphide yolk-shell spheres for enhanced oxygen evolution reaction. Chem. Eng. J. 405, 126580. doi:10.1016/j.cej.2020.126580
Kang, J., Qiu, X., Hu, Q., Zhong, J., Gao, X., Huang, R., et al. (2021). Valence oscillation and dynamic active sites in monolayer NiCo hydroxides for water oxidation. Nat. Catal. 4, 1050–1058. doi:10.1038/s41929-021-00715-w
Kang, J., Xue, Y., Yang, J., Hu, Q., Zhang, Q., Gu, L., et al. (2022). Realizing two-electron transfer in Ni(OH)2 nanosheets for energy storage. J. Am. Chem. Soc. 144, 8969–8976. doi:10.1021/jacs.1c13523
Kang, J., Yang, X., Hu, Q., Cai, Z., Liu, L. M., and Guo, L. (2023). Recent progress of amorphous nanomaterials. Chem. Rev. 123, 8859–8941. doi:10.1021/acs.chemrev.3c00229
Klement, W., Willens, R. H., and Duwez, P. (1960). Non-crystalline structure in solidified gold–silicon alloys. Nature 187, 869–870. doi:10.1038/187869b0
Kuznetsov, A. V., Churkin, O. A., Popov, V. V., Shchetinin, I. V., Ivanov, A. A., Yastrebtsev, A. A., et al. (2020). Magnetization of crystalline and amorphous phases of R2Ti2O7 and R2Zr2O7 (R = Gd, Dy, Tb). J. Supercond. Nov. Magn. 33, 2395–2404. doi:10.1007/s10948-019-05388-y
Lan, H., Wang, J., Cheng, L., Yu, D., Wang, H., and Guo, L. (2024). The synthesis and application of crystalline–amorphous hybrid materials. Chem. Soc. Rev. 53, 684–713. doi:10.1039/d3cs00860f
Lee, J. H., Loh, N. D., Yeo, Z. Y., Ong, Y. K., Balakrishnan, D., Limpo, C. M. A., et al. (2024). Engineering a hierarchy of disorder: a new route to synthesize high-performance 3D nanoporous all-carbon materials. Adv. Mater. 36, 2402628. doi:10.1002/adma.202402628
Li, N., Peng, J., Zhang, P., and Yue, Y. (2023). Amorphous MXene opens new perspectives. Adv. Mater. 35, 2300067. doi:10.1002/adma.202300067
Li, S., Li, Z., Ma, R., Gao, C., Liu, L., Hu, L., et al. (2021). A glass-ceramic with accelerated surface reconstruction toward the efficient oxygen evolution reaction. Angew. Chem. Int. Ed. 60, 3773–3780. doi:10.1002/anie.202014210
Li, X., et al. (2016). Properties, formation mechanism and application of amorphous alloys. Nonferrous Metals Mater. Eng. 37, 233–237.
Lichtenthaler, R. N., Abrams, D. S., and Prausnitz, J. M. (1973). Combinatorial entropy of mixing for molecules differing in size and shape. Can. J. Chem. 51, 3071–3080. doi:10.1139/v73-458
Liu, J., and Guo, L. (2021). In situ self-reconstruction inducing amorphous species: a key to electrocatalysis. Matter 4, 2850–2873. doi:10.1016/j.matt.2021.05.025
Liu, J., Hu, Q., Wang, Y., Yang, Z., Fan, X., Liu, L. M., et al. (2020). Achieving delafossite analog by in situ electrochemical self-reconstruction as an oxygen-evolving catalyst. Proc. Natl. Acad. Sci. U. S. A. 117, 21906–21913. doi:10.1073/pnas.2009180117
Liu, Y., Duan, X., Shin, H. J., Park, S., Huang, Y., and Duan, X. (2021). Promises and prospects of two-dimensional transistors. Nature 591, 43–53. doi:10.1038/s41586-021-03339-z
Lv, C., Yan, C., Chen, G., Ding, Y., Sun, J., Zhou, Y., et al. (2018). An amorphous noble-metal-free electrocatalyst that enables nitrogen fixation under ambient conditions. Angew. Chem. Int. Ed. 57, 6073–6076. doi:10.1002/anie.201801538
Mann, S., Archibald, D. D., Didymus, J. M., Douglas, T., Heywood, B. R., Meldrum, F. C., et al. (1993). Crystallization at inorganic-organic interfaces: biominerals and biomimetic synthesis. Science 261, 1286–1292. doi:10.1126/science.261.5126.1286
Meng, H., Chen, Z., Zhu, J., You, B., Wei, W., et al. (2024). In situ amorphization of electrocatalysts. Adv. Funct. Mater. 34, 2405270. doi:10.1002/adfm.202405270
Moseson, D. E., and Taylor, L. S. (2023). Crystallinity: a complex critical quality attribute of amorphous solid dispersions. Mol. Pharm. 20, 4802–4825. doi:10.1021/acs.molpharmaceut.3c00526
Nai, J., Tian, Y., Guan, X., and Guo, L. (2013). Pearson’s principle inspired generalized strategy for the fabrication of metal hydroxide and oxide nanocages. J. Am. Chem. Soc. 135, 16082–16091. doi:10.1021/ja402751r
Najmaei, S., Liu, Z., Zhou, W., Zou, X., Shi, G., Lei, S., et al. (2013). Vapour phase growth and grain boundary structure of molybdenum disulphide atomic layers. Nat. Mater. 12, 754–759. doi:10.1038/nmat3673
Ozden, M. G., and Morley, N. A. (2021). Laser additive manufacturing of Fe-based magnetic amorphous alloys. Magnetochemistry 7, 20. doi:10.3390/magnetochemistry7020020
Palmer, R. G., Stein, D. L., Abrahams, E., and Anderson, P. W. (1984). Models of hierarchically constrained dynamics for glassy relaxation. Phys. Rev. Lett. 53, 958–961. doi:10.1103/physrevlett.53.958
Pinal, R. (2008). Entropy of mixing and the glass transition of amorphous mixtures. Entropy 10, 207–223. doi:10.3390/entropy-e10030207
Qiao, J., Yu, P., Wu, Y., Chen, T., Du, Y., and Yang, J. (2020). A compact review of laser welding technologies for amorphous alloys. Metals 10, 1690. doi:10.3390/met10121690
Sarkar, D., Xie, X., Liu, W., Cao, W., Kang, J., Gong, Y., et al. (2015). A subthermionic tunnel field-effect transistor with an atomically thin channel. Nature 526, 91–95. doi:10.1038/nature15387
Shechtman, D., Blech, I., Gratias, D., and Cahn, J. W. (1984). Metallic phase with long-range orientational order and No translational symmetry. Phys. Rev. Lett. 53, 1951–1953. doi:10.1103/physrevlett.53.1951
Shen, S., Wang, Z., Lin, Z., Song, K., Zhang, Q., Meng, F., et al. (2022). Crystalline-amorphous interfaces coupling of CoSe2/CoP with optimized d-band center and boosted electrocatalytic hydrogen evolution. Adv. Mater. 34, 2110631. doi:10.1002/adma.202110631
Sheng, H. W., Liu, H. Z., Cheng, Y. Q., Wen, J., Lee, P. L., Luo, W. K., et al. (2007). Polyamorphism in a metallic glass. Nat. Mater. 6, 192–197. doi:10.1038/nmat1839
Spurgeon, S. R., Ophus, C., Jones, L., Petford-Long, A., Kalinin, S. V., Olszta, M. J., et al. (2021). Towards data-driven next-generation transmission electron microscopy. Nat. Mater. 20, 274–279. doi:10.1038/s41563-020-00833-z
Stachurski, Z. H. (2011). On structure and properties of amorphous materials. Materials 4, 1564–1598. doi:10.3390/ma4091564
Suarez, S. A. (2024). The master key: structural science in unlocking functional materials advancements. J. Appl. Crystallogr. 57, 606–622. doi:10.1107/s1600576724003674
Sun, B., Xu, M., Li, X., Zhang, B., Hao, R., Fan, X., et al. (2024). Unlocking single-atom catalysts via amorphous substrates. Nano Res. 17, 3533–3546. doi:10.1007/s12274-023-6312-8
Sun, K., Fu, R., Liu, X., Xu, L., Wang, G., Chen, S., et al. (2022). Osteogenesis and angiogenesis of a bulk metallic glass for biomedical implants. Bioact. Mater. 8, 253–266. doi:10.1016/j.bioactmat.2021.06.018
Tian, H., Yao, Z., Li, Z., Guo, J., and Liu, L. (2023). Unlocking more potentials in two-dimensional space: disorder engineering in two-dimensional amorphous carbon. ACS Nano 17, 24468–24478. doi:10.1021/acsnano.3c09593
Toh, C.-T., Zhang, H., Lin, J., Mayorov, A. S., Wang, Y. P., Orofeo, C. M., et al. (2020). Synthesis and properties of free-standing monolayer amorphous carbon. Nature 577, 199–203. doi:10.1038/s41586-019-1871-2
Tong, X., Cao, X., Han, T., Cheong, W. C., Lin, R., Chen, Z., et al. (2019). Convenient fabrication of BiOBr ultrathin nanosheets with rich oxygen vacancies for photocatalytic selective oxidation of secondary amines. Nano Res. 12, 1625–1630. doi:10.1007/s12274-018-2404-x
Urbain, F., Smirnov, V., Becker, J. P., Lambertz, A., Yang, F., Ziegler, J., et al. (2016). Multijunction Si photocathodes with tunable photovoltages from 2.0 V to 2.8 V for light induced water splitting. Energy Environ. Sci. 9, 145–154. doi:10.1039/c5ee02393a
van der Zande, A. M., Huang, P. Y., Chenet, D. A., Berkelbach, T. C., You, Y., Lee, G. H., et al. (2013). Grains and grain boundaries in highly crystalline monolayer molybdenum disulphide. Nat. Mater. 12, 554–561. doi:10.1038/nmat3633
Wang, G., Liu, J., Zhu, L., Ma, X., Wang, X., Yang, X., et al. (2019). Self-destruction of cancer induced by Ag2S amorphous nanodots. Small 15, 1902945. doi:10.1002/smll.201902945
Wang, J., Yang, M., and Wang, D. (2022). Progress and perspectives of hollow multishelled structures. Chin. J. Chem. 40, 1190–1203. doi:10.1002/cjoc.202100932
Wang, J.-Q., Song, L. J., Huo, J. T., Gao, M., and Zhang, Y. (2024). Designing advanced amorphous/nanocrystalline alloys by controlling the energy state. Adv. Mater. 36, 2311406. doi:10.1002/adma.202311406
Wang, W., Yang, X., Liu, Y., Wang, C., Li, J., et al. (2021). General synthesis of amorphous PdM (M = Cu, Fe, Co, Ni) alloy nanowires for boosting HCOOH dehydrogenation. Nano Lett. 21, 3458–3464. doi:10.1021/acs.nanolett.1c00074
Wang, W. H. (2022). Development trends and insights of amorphous alloy materials. Bull. Chin. Acad. Sci. 37, 352–359.
Wang, X., et al. (2005). Template - synthesis of nanostructured materials. Chem. Bull. 723-730. doi:10.14159/j.cnki.0441-3776.2005.10.001
Wang, X. J., et al. (2006). The current situation of amorphous alloy application. Mater. Rev. 20 (10) 75–79.
Wang, Z., Ren, X., Shi, X., Asiri, A., Wang, L., Li, X., et al. (2018). A platinum oxide decorated amorphous cobalt oxide hydroxide nanosheet array towards alkaline hydrogen evolution. J. Mater. Chem. A 6, 3864–3868. doi:10.1039/c8ta00241j
Wang, Z., Wang, Z., Liu, W., Xiao, W., and Lou, X. W. (2012). Amorphous CoSnO3@C nanoboxes with superior lithium storage capability. Energy Environ. Sci. 6, 87–91. doi:10.1039/c2ee23330d
Wei, B. Q., Vajtai, R., Jung, Y., Ward, J., Zhang, R., Ramanath, G., et al. (2002). Organized assembly of carbon nanotubes. Nature 416, 495–496. doi:10.1038/416495a
Wei, Y., Liu, S., Xiao, Z., Zhao, H., Luo, J., Deng, X., et al. (2020). Enamel repair with amorphous ceramics. Adv. Mater. 32, 1907067. doi:10.1002/adma.201907067
Wilding, M. C., and Benmore, C. J. (2006). Structure of glasses and melts. Neutron Scatt. Earth Sci. 63, 275–311. doi:10.2138/rmg.2006.63.12
Wu, G., Chan, K.-C., Zhu, L., Sun, L., and Lu, J. (2017). Dual-phase nanostructuring as a route to high-strength magnesium alloys. Nature 545, 80–83. doi:10.1038/nature21691
Wu, G., Zheng, X., Cui, P., Jiang, H., Wang, X., Qu, Y., et al. (2019). A general synthesis approach for amorphous noble metal nanosheets. Nat. Commun. 10, 4855. doi:10.1038/s41467-019-12859-2
Wu, Y., Zhang, Y., and Zhang, T. (2021). Application of 3D balanced growth theory to the formation of bulk amorphous alloys. Front. Mater. 8. doi:10.3389/fmats.2021.694920
Xue, J., Wu, T., Dai, Y., and Xia, Y. (2019). Electrospinning and electrospun nanofibers: methods, materials, and applications. Chem. Rev. 119, 5298–5415. doi:10.1021/acs.chemrev.8b00593
Yang, D., Song, Y., Zhang, M., Qin, Z., Dong, R., Li, C., et al. (2021). A manganese phosphate cathode for long-life aqueous energy storage. Adv. Funct. Mater. 31, 2100477. doi:10.1002/adfm.202100477
Yao, Y., Dong, Q., Brozena, A., Luo, J., Miao, J., Chi, M., et al. (2022). High-entropy nanoparticles: synthesis-structure-property relationships and data-driven discovery. Science 376, eabn3103. doi:10.1126/science.abn3103
Yao, Y., Huang, Z., Hughes, L. A., Gao, J., Li, T., Morris, D., et al. (2021). Extreme mixing in nanoscale transition metal alloys. Matter 4, 2340–2353. doi:10.1016/j.matt.2021.04.014
Ye, Y. F., Wang, Q., Lu, J., Liu, C. T., and Yang, Y. (2015). Design of high entropy alloys: a single-parameter thermodynamic rule. Scr. Mater. 104, 53–55. doi:10.1016/j.scriptamat.2015.03.023
Yin, X., and Xu, S. (2018). Properties and preparation of high entropy alloys. MATEC Web Conf. 142, 03003. doi:10.1051/matecconf/201814203003
Yoon, S., Yun, J.-Y., Lim, J.-H., and Yoo, B. (2017). Enhanced electrocatalytic properties of electrodeposited amorphous cobalt-nickel hydroxide nanosheets on nickel foam by the formation of nickel nanocones for the oxygen evolution reaction. J. Alloys Compd. 693, 964–969. doi:10.1016/j.jallcom.2016.09.247
Yu, H.-D., Regulacio, M. D., Ye, E., and Han, M.-Y. (2013). Chemical routes to top-down nanofabrication. Chem. Soc. Rev. 42, 6006–6018. doi:10.1039/c3cs60113g
Zhang, J., Li, Y., Chen, Z., Liu, Q., Chen, Q., and Chen, M. (2023). Amorphous electrode: from synthesis to electrochemical energy storage. ENERGY Environ. Mater. 6, e12573. doi:10.1002/eem2.12573
Zhang, X., Zhang, Y., Yu, B. B., Yin, X. L., Jiang, W. J., Jiang, Y., et al. (2015). Physical vapor deposition of amorphous MoS2 nanosheet arrays on carbon cloth for highly reproducible large-area electrocatalysts for the hydrogen evolution reaction. J. Mater. Chem. A 3, 19277–19281. doi:10.1039/c5ta05793k
Zhao, D., Yang, N., Wei, Y., Jin, Q., Wang, Y., He, H., et al. (2020). Sequential drug release via chemical diffusion and physical barriers enabled by hollow multishelled structures. Nat. Commun. 11, 4450. doi:10.1038/s41467-020-18177-2
Zhao, D., Yang, N., Xu, L., Du, J., Yang, Y., and Wang, D. (2022b). Hollow structures as drug carriers: recognition, response, and release. Nano Res. 15, 739–757. doi:10.1007/s12274-021-3595-5
Zhao, H., Chen, X., Wang, G., Qiu, Y., and Guo, L. (2019). Two-dimensional amorphous nanomaterials: synthesis and applications. 2D Mater. 6, 032002. doi:10.1088/2053-1583/ab1169
Zhao, H., Liu, S., Wei, Y., Yue, Y., Gao, M., Li, Y., et al. (2022a). Multiscale engineered artificial tooth enamel. Science 375, 551–556. doi:10.1126/science.abj3343
Zhao, H., Yue, Y., Zhang, Y., Li, L., and Guo, L. (2016a). Ternary artificial nacre reinforced by ultrathin amorphous alumina with exceptional mechanical properties. Adv. Mater. 28, 2037–2042. doi:10.1002/adma.201505511
Zhao, H., Yue, Y., Zhang, Y., Li, L., and Guo, L. (2016b). Ternary artificial nacre reinforced by ultrathin amorphous alumina with exceptional mechanical properties. Adv. Mater. 28, 2037–2042. doi:10.1002/adma.201505511
Keywords: amorphous, micro-nano materials, construction, applications, nanomaterials
Citation: Liu G and Huang H (2025) Research progress of amorphous micro-nano structured materials. Front. Mater. 12:1589830. doi: 10.3389/fmats.2025.1589830
Received: 08 March 2025; Accepted: 16 April 2025;
Published: 06 May 2025.
Edited by:
Erkan Oterkus, University of Strathclyde, United KingdomReviewed by:
Yuyao Kuang, University of California, Irvine, United StatesSebastian A. Suarez, Adam Mickiewicz University, Poland
Copyright © 2025 Liu and Huang. This is an open-access article distributed under the terms of the Creative Commons Attribution License (CC BY). The use, distribution or reproduction in other forums is permitted, provided the original author(s) and the copyright owner(s) are credited and that the original publication in this journal is cited, in accordance with accepted academic practice. No use, distribution or reproduction is permitted which does not comply with these terms.
*Correspondence: He Huang, aHVhbmdoZV8xOTcyQDE2My5jb20=