- 1Laboratorio Neuroimmagini, Fondazione Santa Lucia IRCCS, Rome, Italy
- 2MARBILab - Museo storico della fisica e Centro studi e ricerche Enrico Fermi, Rome, Italy
- 3Laboratori Nazionali del Sud, Istituto Nazionale di Fisica Nucleare, Catania, Italy
Sodium magnetic resonance imaging is a non-invasive technique that provides information about sodium levels in tissues. It has significant applications in brain research due to the important role of sodium in both normal brain function and pathological processes. Total sodium concentration is the most widely used derived metric; it offers insights into sodium content across different brain regions. However, the functional role of sodium is closely linked to its distribution within intra- and extracellular spaces. Sodium osmotic homeostasis affects the intracellular volume fraction, a parameter that can be altered in various neurological disorders. Unfortunately, distinguishing intracellular from extracellular sodium nuclear magnetic resonance signals is challenging, even with the use of contrast agents. In recent years, several methodologies have been proposed to study sodium compartmentalization in humans, typically involving tailored acquisition techniques and modeling approaches. This mini-review provides a brief overview of the challenges, methodologies, and potential applications of compartmentalized sodium MR imaging in human neuroscience.
Introduction
Magnetic resonance imaging (MRI) is a non-invasive imaging technique widely used in clinical practice. Conventional MRI is based on hydrogen nuclei because of their large gyromagnetic ratio and abundance in the human body. Notwithstanding the ubiquitous presence of 1H-based MRI and its flexibility for multiple contrasts, other nuclei can offer insights into specific mechanisms. In recent years, the use of heteronuclear MRI, and in particular, of sodium MRI, has significantly expanded. The dynamics of sodium is crucial for several physiological processes, including neuronal excitability, synaptic transmission, and cellular energy metabolism; indeed, approximately 15% of the gray matter energy budget and 44% of the white matter ATP turnover is believed to be used only to maintain the resting potential, neglecting signaling. Overall, up to 50% of the cortical energy budget is assigned to Na+/K+ ATPase [1] and is thus needed to maintain sodium and potassium concentration gradients across the cellular membrane. For sodium, the intracellular and extracellular concentrations are approximately 10–15 mM and 140 mM, respectively [2, 3]. Dysfunctions in sodium homeostasis are associated with various neurological conditions, including multiple sclerosis (MS), Alzheimer’s disease (AD), stroke, epilepsy, and brain tumors [4]. Therefore, sodium represents a promising biomarker [5].
Sodium is the most abundant cation, providing the second strongest nuclear magnetic resonance (NMR) signal in the human body after hydrogen, but its lower gyromagnetic ratio (11.3 MHz/T), lower abundance in the human brain, and nuclear spin of 3/2 imply a sensitivity which is only 9.3% of that of the proton. These factors lead to a poor signal-to-noise ratio (SNR) [2], which is partially compensated by the use of ultra-high field strengths (7 T ad above) [6–9]. Sodium is characterized by a quadrupole magnetic moment, leading to two distinct T2 relaxation times: a slow component of 15–40 m and a fast component of 0.5–8 ms [10–13]. The fast component accounts for approximately 60% of the total sodium NMR signal, requiring MR sequences with very short echo time (TE).
After the first pioneering sodium studies on humans and intact animals, performed in the early 1980s [14, 15], the feasibility of 23Na-MRI in clinical settings has benefited from recent advances in MRI technology and acquisition strategies. In particular, non-Cartesian k-space sampling offers advantages in terms of spatial resolution and acquisition time [16], while ultrashort TE (UTE) or zero TE (ZTE) sequences allow the detection of the short T2 tissue components [17]. UTE sequences begin the acquisition with a minimal delay after the excitation pulse [18], while in ZTE sequences, the sampling gradients are turned on before the radiofrequency (RF) excitation pulse. In ZTE sequences, the center of the k-space is crossed at zero echo time, and due to the switching of hardware from the transmit to receive mode, the center of the k-space is not sampled. Different strategies have been developed to overcome this problem, such as single-point acquisitions (PETRA) [19]. UTE and ZTE acquisition techniques can be customized in terms of SNR, total acquisition time, and point spread function by adapting the density of the k-space points. Examples include density-adapted radial sequence [20], 3D cones [21], twisted projection imaging (TPI) [16, 22], and FLORET [23]. Non-Cartesian sequence reconstruction methods use regridding, which involves interpolating the sampled data points in a rectilinear grid [24], and performing fast Fourier transform (FFT) or non-uniform FFT [12] on the regridded data [25].
Sodium compartmentalization
Sodium MRI of the brain can follow different approaches according to the information of interest. The basic approach treats the brain as a single compartment and uses UTE or ZTE sequences to collect spin-density weighted (SDW) 23Na MR data. Then, a calibration is performed in order to determine the tissue sodium concentration (TSC). However, changes in TSC can be the result of independent changes within the intracellular and extracellular compartments (IC and EC, respectively). Moreover, pathologies are often associated with changes in the volume fraction [26]. Distinguishing IC from EC sodium requires a combination of acquisition techniques and mathematical models. Most experimental approaches exploit the different relaxation times between bound sodium and free sodium [27], assuming that the intracellular component is mainly composed of bound sodium. Being generally suppression techniques, that is, techniques that selectively suppress or filter a component of the signal, they tend to suffer from low SNR and incomplete suppression.
Sequences
Some sequences have been introduced to isolate IC sodium, but their effectiveness is still debated. These include triple quantum filtering (TQF), double single-quantum (SQ) acquisition, inversion recovery (IR), and bi-exponential weighting.
TQF
The sodium nucleus is a spin 3/2 system with four degenerate spin states (3/2, ½, −½, and −3/2), and is thus characterized by a quadrupolar moment. The transition between the levels can occur via single, double, or triple quantum coherence; SQ relaxation involving the ±3/2 states (outer levels) is faster (T2short) than the relaxation involving the inner levels (T2long). However, if sodium is free (that is, the motional correlation time is much smaller than the Larmor period), the two exponential decays degenerate to a single, longer decay time [13].
The TQF sequence consists of three excitation pulses with the same flip angle and three different phases. The delays between pulses create higher-order coherences, and the read-out signal consists of contributions from all coherence pathways, which can be selected by phase cycling or gradients. The goal is to retain the signal from bound sodium (intracellular) [28]. The intracellular sodium molar fraction (ISMF) and the TSC can be measured directly with MRI, while the intracellular sodium concentration (ISC) and the intracellular volume fraction can be obtained by the combination of the first two quantities (see the Mathematical models section). These parameters can give complementary information about tissue state: for example, an increase of TSC and ISC with no increase of ISMF can follow cellular death, while an increase of ISMF with no increase of TSC is associated with cell swelling [29]. TQF presents several problems: low signal from filtering, high SAR from the multiple RF pulses, and the dependence on T2 leads to low SNR, long acquisition times, and poor quantification. Fiege et al. proposed a method for simultaneous acquisition of single quantum and TQF to reduce total scan time, called SISTINA [30], which was later optimized and enhanced to include relaxometry [31, 32].
Dual SQ acquisition
Bound (intracellular) sodium is characterized by a bi-exponential decay with a T2short component amounting to approximately 60% of the signal [7, 33–41]; the contribution of the T2long component can be obtained by subtracting an SQ image acquired with a short echo time, where the T2short component is suppressed, from one obtained with an ultrashort echo time, including signals from nearly all sodium nuclei. This technique is characterized by lower SAR and higher SNR than TQF at the expense of incomplete suppression of the T2long signal [27].
IR
Inversion recovery acquisition is a widespread technique used to suppress the signal coming from a specific compartment, exploiting different T1 relaxations between tissues. The sequence is a standard spin echo preceded by a 180° RF preparation pulse, where the excitation pulse is applied at the specific inversion time (TI) corresponding to the minimum magnetization in the compartment to suppress. While the extracellular space is rich in molecules, it is less dense than cytoplasm. Thus, EC T1 is expected to be longer than IC T1 [42], allowing IR-based selective suppression; in IR-based suppression, SAR can be high, SNR low, and suppression incomplete. However, long (soft) inversion pulses reduce SAR. In sodium MRI, relaxation during long RF pulses cannot be ignored because of very low T2. It has been shown that by tailoring the duration of a soft inversion pulse, the different relaxation properties of IC and EC compartments allow for efficient extracellular signal attenuation while increasing intracellular magnetization at the EC-nulling TI (Soft Inversion Recovery FLuid Attenuation, SIRFLA) [42]. In vivo acquisitions at 4.7 T showed an SNR of 18 for brain tissues and an SNR of 3 for the cerebrospinal fluid (CSF) [43].
Bi-exponential weighting
Another approach is to differentiate between bound and free sodium by exploiting the differences in transverse relaxation [44]. The method is still based on multiple-quantum filtering techniques but with the benefit of improved SNR. Two images with different contrasts are acquired and subtracted to generate a bi-exponential weighted image contrast [44]. The first image is an SDW acquired after the first 90° RF pulse, containing the contribution of all sodium ions, and the second one is a single quantum filtering (SQF) acquired after a second 90° RF pulse, containing mostly signals from mono-exponentially (free) relaxing sodium. The difference image can be calculated as follows [44]:
where S indicates the signal, DIM is the difference image method, and v is a weighting factor that takes into account the signal losses between the two acquisitions due to T2* relaxation, namely:
where TE1 and TE2 are the two echo times of the acquisitions, and
Mathematical models
An estimate of tissue-specific sodium concentration can be obtained by applying mathematical models to data acquired with the appropriate weighting. The most used method considers two compartments, IC and EC, but the inclusion of more compartments has been proposed. Madelin et al. [2] developed a three-compartment model by dividing the brain into IC, EC, and a solid-like compartment constituted of cell membranes and nuclei, proteins, and other large molecules. The model was further developed by Gilles et al. [45] to include a CSF compartment.
2CM model
The two-compartment (2CM) approach [8, 46] partitions the tissue into homogeneous IC and EC compartments, characterized by volume
The intracellular volume fraction η and the intracellular sodium molar fraction ISMF (χ in the equations) can thus be written as
Combining these equations, it is possible to obtain
Total sodium concentration
3CM model
The three-compartment model (3CM)adds to IC and EC a solid compartment (SC, index i = 3) that includes cell membranes, nuclei, proteins, and other metabolites. The solid compartment has no sodium content (
where
where
4CM model
The four-compartment model (4CM) [45] is a further refinement of the 3CM. Here, the CSF is considered a distinct compartment (i = 4), and
where
Compartmentalized sodium MRI in brain diseases
23Na-MRI is sensitive to physiological and pathological processes that happen during the progression of several neurological diseases [50, 51]: in fact, many brain disorders show sodium concentration anomalies associated with sodium–potassium pump dysfunction or cellular membrane impairment, allowing a sodium MRI to target specific features of the pathology. Anomalies in overall sodium are often caused by an increase in the intracellular sodium concentration or the extracellular volume fraction. Therefore, differentiating the compartments can help and improve the diagnostic information of sodium imaging.
Anomalies in sodium levels play a crucial role in the pathophysiology of multiple sclerosis [52]. The most common form of MS is characterized by a first period of relapsing/remitting (RR) symptoms that later converts into a secondary progression phase (SPMS). RRMS is primarily characterized by demyelination, resulting in impairment of action potential conduction. The demyelinated axons can experience an overexpression of sodium channels along their membranes. Albeit this process re-establishes signal transduction and contributes to clinical remission in RRMS, it is responsible for higher sodium influx, resulting in an increased energy demand to maintain resting potential and sodium homeostasis. Moreover, intra-axonal sodium accumulation contributes to axonal degeneration by reversal of the sodium/calcium exchanger that increases intra-axonal calcium (Ca), ultimately causing irreversible damage, whose accumulation leads to SPMS [53–55].
Considering the fundamental role of sodium in MS, 23Na-MRI has been widely employed. TSC has been studied in normal-appearing white matter (NAWM), normal-appearing grey matter (NAGM), CSF, and MS lesions [55–58]. Various studies have shown an increase of TSC in lesions as well as in cortical gray and white matter across primary, secondary, and relapsing-remitting multiple sclerosis (RRMS) [55–57]. Additionally, sodium levels are linked to clinical measures of disability and impairment [57]. Studies on lesions have demonstrated that TSC is elevated in contrast-enhancing lesions, T1 hypointense lesions, and T1 isointense lesions, while non-enhancing lesions with a reduced apparent diffusion coefficient (ADC) exhibit TSC levels that are comparable to those in NAWM. This consistency in TSC suggests that the tissue structure may be preserved early on, prior to any breakdown of the blood–brain barrier (BBB). Thus, TSC may represent a sensitive biomarker of chronic tissue abnormalities, BBB disruption, and vasogenic edema in contrast-enhancing lesions. Normal levels of TSC in lesions with a decreased ADC may indicate that the lesions are in an early stage [10, 59, 60].
The well-demonstrated increase of TSC in MS can be driven by intracellular sodium accumulation, expansion of the extracellular space, or both [57]. Strategies based on single-quantum, inversion recovery, and triple-quantum filtering have been used to disentangle the intracellular contribution [8]. Findings suggest that intracellular sodium concentration can give complementary information about MS lesions and inflammatory processes. Petracca et al. [8] found an increase in ISC in both WM and GM of RRMS patients; a decrease of the intracellular volume fraction was observed in several clusters in WM and GM, while changes at the whole-brain scale were significant only in WM. Interestingly, lesion burden and disability were correlated to TSC and intracellular volume fraction but not to ISC, suggesting that the latter is a consequence of early neuro/axonal metabolic dysfunction, while the former reflects tissue loss [8]. Studies on lesions have demonstrated a different intracellular concentration between hyperacute, acute, and chronic ones [61, 62], suggesting the sensitivity of ISC to reveal inflammatory mechanisms during the first stage of the disease (see Table 1).
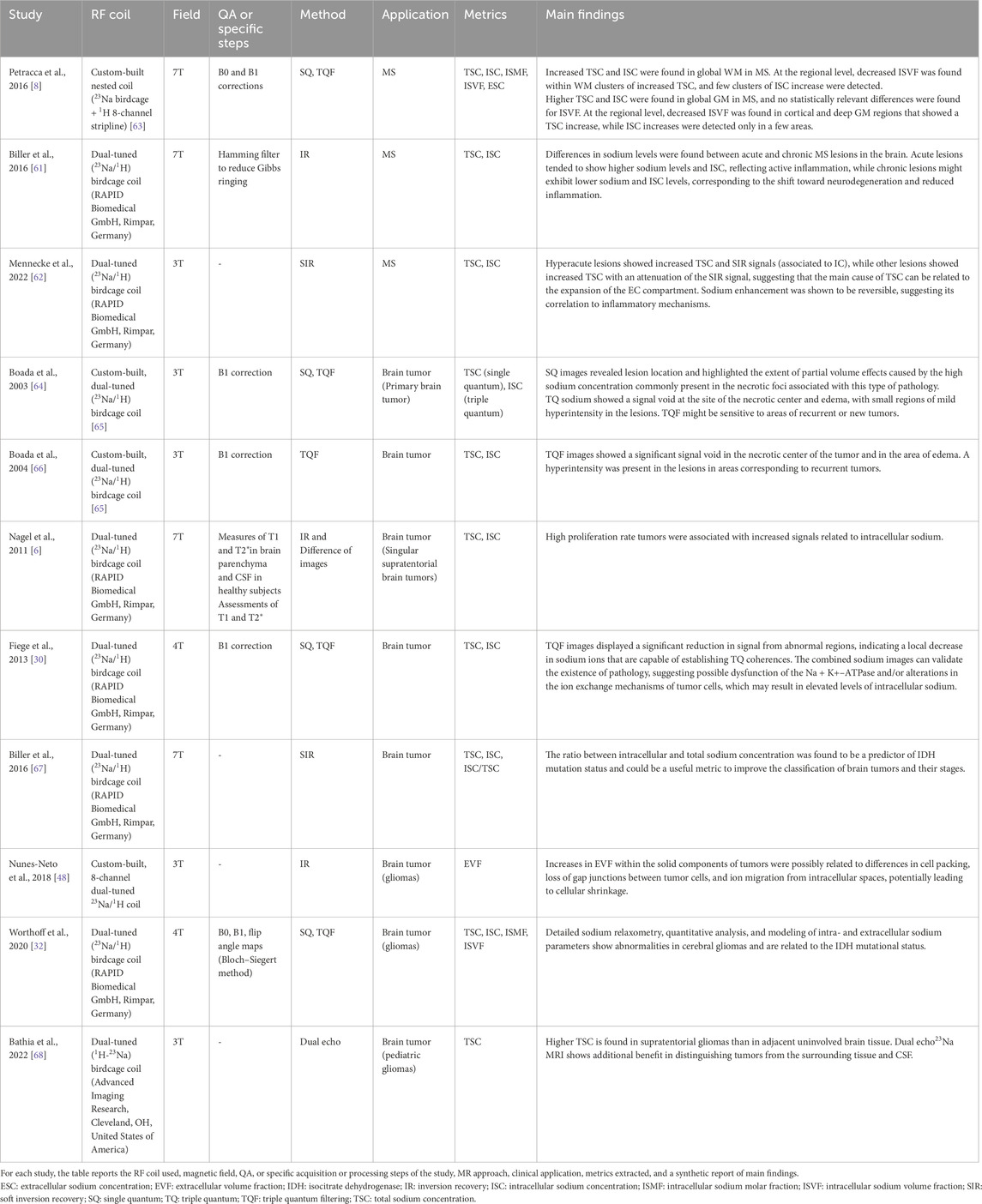
Table 1. Applications and main findings of sodium compartmentalization techniques in multiple sclerosis and brain tumors.
Alzheimer’s disease is characterized by extracellular deposits of β amyloid peptides (Aβ). Postmortem studies have shown a correlation between Aβ accumulation and ion imbalance [69–71]; disruption of Na+ homeostasis is induced by Aβ oligomers and is responsible for neuronal network destabilization in the early stage of the disease [71]. TSC was found to be high in AD patients, suggesting that sodium imbalance during the progression of the disease might have a key role in energy failure [72, 73]. However, recent ex vivo studies have shown that sodium increase in brain tissue does not correspond to an increase in the CSF, indicating that anomalies can be associated with the intracellular pool [69]. However, no direct evidence exists for this hypothesis so far.
Stroke is one of the most common causes of death and the leading cause of long-term disability. During a stroke, the blood flow in the brain is compromised, resulting in a breakdown of cellular energy production, followed by the piling of sodium and calcium in the intracellular compartment. A measure of intracellular sodium would have the benefit of being related to the direct pathological consequence of stroke. A study on non-human primates has shown that the duration of brain ischemia can be associated with a threshold on the TSC beyond which tissue reperfusion would be of little benefit [74]. Studies on humans have demonstrated how sodium can be considered a promising biomarker for tissue viability and cell integrity, and how its integration into clinical practice can have an impact on future diagnosis and treatments [75–77]. It is likely that knowledge about IC sodium would help personalized decisions on reperfusion.
Epilepsy is characterized by recurring seizures, causing a large inflow of sodium. Intracellular 23Na-MRI is thus an obvious biomarker to quantify the physiological effects of seizures. Moreover, cell shrinkage and cerebral atrophy have been reported in epileptic areas [78, 79]. Both phenomena are associated with an increase in the extracellular volume fraction, resulting in an increase of TSC in the epileptogenic zone [80]. An increase in TSC in epileptogenic zones suggests intracellular sodium accumulation, even in interictal periods [81], and demonstrates long-term changes in sodium levels [80, 82]. A decrease in TSC was observed in a patient who suffered non-convulsive seizures during the scan, suggesting transient neuronal swelling and reduction of extracellular volume fraction [82]. Some recent studies [80, 83] investigated the ratio between T2*short and T2*long, showing an increase of the short component mainly localized to epileptogenic zones, whereas TSC was increased in all regions. These results suggest that a differential weighting of intracellular/extracellular sodium can be sufficient to highlight specific pathophysiological phenomena.
Traumatic brain injury (TBI) is the result of acute events in which an external force damages the brain. TBI causes stretch-induced damage to the cell membranes along the axons, causing mechanical damage and mechanoporation [84], triggering ionic and proteolytic cascades, and ultimately resulting in disruption of ionic homeostasis. Sodium imbalances following mild TBI are associated with patient outcomes [85]. Surprisingly, a decrease of TSC in TBI was reported [84, 85], contrary to what could be expected. A recent longitudinal study [86] has investigated TSC levels 3 months after the injury, when no significant variations of TSC were found, suggesting normalization of the sodium ionic equilibrium.
Brain tumors represent another important application for 23Na-MRI. The most aggressive are characterized by rapid cell proliferation and angiogenesis: both of these factors are linked to a reduced Na+-K+-ATPase activity [87], resulting in an increase of the intracellular sodium concentration. Cellular proliferation can also be associated with an increase in extracellular volume fraction [88]. The level of Na+ in malignant tumors is higher than in healthy tissues [46, 89–95]. IC sodium is thus a potential biomarker for cancer staging. Moreover, EC volume fraction is expected to increase following treatment. Therefore, compartmentalized sodium measurements are a promising biomarker for evaluating response to treatment. Studies on compartments have demonstrated a decrease in the signal associated with intracellular sodium (see Table 1) [30, 48, 64, 66, 96]. However, Nagel et al. [6] reported an increased IR signal (associated with intracellular sodium) for tumors with high proliferation rates: a positive correlation between the MIB-1 proliferation rate and the IR signal was found, and no correlation considering TSC values was found. These results suggest the potential of compartmentalization to discriminate among different types of brain tumors [6, 67] and their progression [68].
Technical remarks
The disentanglement of IC and EC sodium needs specific acquisition sequences and mathematical models. The scanning sequences developed for selective acquisition from a single compartment suffer from some limitations: they are based on the idea that intracellular and extracellular pools are composed of bound sodium and free sodium, respectively, and have different relaxation properties, but this hypothesis is likely an oversimplification, leading to partial or poor compartment specificity [97]. Moreover, all scanning approaches for compartmentalized sodium quantification suffer from poor SNR and a very long scanning time.
Sodium imaging is, in general, impaired by hardware and technical limitations. The challenge with RF technology for sodium MRI lies in the ability to detect small signals with very short T2 relaxation times [98, 99], thus requiring high sensitivity and fast switching between transmit and receive modes. While most sodium coils used to be custom-built or handmade, commercial RF coils, including 23Na/1H birdcage coils and surface coils, are now available. Surface coils are more sensitive, but limited coverage and highly inhomogeneous B1 field impair quantitative studies [98, 100]. In recent years, phased array sodium RF receive coils have been introduced. The efficient design of phased array receive coils and the added benefit of potential parallel imaging are attractive, but these coils necessitate correction methods for accurate sodium quantification [26, 101–103]. Acquisition strategies may also be optimized; recent studies have proposed methods to reduce scan time using postprocessing with convolutional neural networks [77] or fingerprinting to simultaneously acquire sodium density and sodium relaxation parameters and improve sodium MRI resolution [104–107].
Part of the ongoing research still relies on simulations and phantom studies. Phantoms with varying sodium and agar concentrations can simulate the relaxation behavior of compartments and aid in optimizing sequence parameters. However, further development is required regarding the quality of agar phantoms and the development of more stable alternatives to enhance optimization and sodium quantification [108].
Finally, improvements are expected from the diffusion of deep-learning-based methods for denoising or model optimization and fitting, but the relatively limited size of 23Na datasets requires tailored solutions [109].
Conclusion
The first MRI studies on compartmentalization of 23Na were presented more than 20 years ago. Because sodium compartmentalization is directly linked to cellular homeostasis, it can provide unique, relevant, and specific clinical information about tissue integrity and physiology in several serious pathologies, including cancer and neurodegenerative or neuroinflammatory diseases.
However, technical difficulties of scanning and postprocessing, as well as scanning time, currently make compartmentalized sodium imaging a tool for clinical research that is not expected to expand to clinical practice in the near future.
Author contributions
IE: investigation, visualization, and writing – original draft. MG: visualization and writing – review and editing. FG: conceptualization, funding acquisition, supervision, visualization, and writing – review and editing.
Funding
The author(s) declare that financial support was received for the research and/or publication of this article. The work was funded by the European Union – Next Generation EU – NRRP M6C2 – Investment 2.1 Enhancement and strengthening of biomedical research in the NHS under the grant PNRR-MAD-2022-12376889.
Conflict of interest
The authors declare that the research was conducted in the absence of any commercial or financial relationships that could be construed as a potential conflict of interest.
The author(s) declared that they were an editorial board member of Frontiers, at the time of submission. This had no impact on the peer review process and the final decision.
Publisher’s note
All claims expressed in this article are solely those of the authors and do not necessarily represent those of their affiliated organizations, or those of the publisher, the editors and the reviewers. Any product that may be evaluated in this article, or claim that may be made by its manufacturer, is not guaranteed or endorsed by the publisher.
References
1. Engl E, Attwell D. Non-signalling energy use in the brain. J Physiol (2015) 593(16):3417–29. doi:10.1113/jphysiol.2014.282517
2. Madelin G, Kline R, Walvick R, Regatte RR. A method for estimating intracellular sodium concentration and extracellular volume fraction in brain in vivo using sodium magnetic resonance imaging. Scientific Rep (2014) 4(1):4763–7. doi:10.1038/srep04763
3. Murphy E, Eisner DA. Regulation of intracellular and mitochondrial sodium in health and disease. Circ Res (2009) 104(3):292–303. doi:10.1161/circresaha.108.189050
4. Ha Y, Jeong JA, Kim Y, Churchill DG. Sodium and potassium relating to Parkinson's disease and traumatic brain injury. Met Ions Life Sci (2016) 16:585–601. doi:10.1007/978-3-319-21756-7_16
5. Nielles-Vallespin S, Weber M, Bock M, Bongers A, Speier P, Combs SE, et al. 3D radial projection technique with ultrashort echo times for sodium MRI: clinical applications in human brain and skeletal muscle. Magn Reson Med Official J Int Soc Magn Reson Med (2007) 57(1):74–81. doi:10.1002/mrm.21104
6. Nagel AM, Bock M, Hartmann C, Gerigk L, Neumann JO, Weber MA, et al. The potential of relaxation-weighted sodium magnetic resonance imaging as demonstrated on brain tumors. Invest Radiol (2011) 46(9):539–47. doi:10.1097/rli.0b013e31821ae918
7. Ridley B, Nagel AM, Bydder M, Maarouf A, Stellmann JP, Gherib S, et al. Distribution of brain sodium long and short relaxation times and concentrations: a multi-echo ultra-high field 23Na MRI study. Scientific Rep (2018) 8(1):4357. doi:10.1038/s41598-018-22711-0
8. Petracca M, Vancea RO, Fleysher L, Jonkman LE, Oesingmann N, Inglese M. Brain intra- and extracellular sodium concentration in multiple sclerosis: a 7 T MRI study. Brain (2016) 139(Pt 3):795–806. doi:10.1093/brain/awv386
9. Fleysher L, Oesingmann N, Stoeckel B, Grossman RI, Inglese M. Sodium long-component T mapping in human brain at 7 Tesla. Magn Reson Med Official J Int Soc Magn Reson Med (2009) 62(5):1338–41. doi:10.1002/mrm.22133
10. Huhn K, Engelhorn T, Linker RA, Nagel AM. Potential of sodium MRI as a biomarker for neurodegeneration and neuroinflammation in multiple sclerosis. Front Neurol (2019) 10:84. doi:10.3389/fneur.2019.00084
11. Shah NJ, Worthoff WA, Langen KJ. Imaging of sodium in the brain: a brief review. NMR Biomed (2016) 29(2):162–74. doi:10.1002/nbm.3389
12. Madelin G, Lee JS, Regatte RR, Jerschow A. Sodium MRI: methods and applications. Prog Nucl Magn Reson Spectrosc (2014) 79:14–47. doi:10.1016/j.pnmrs.2014.02.001
13. Madelin G, Regatte RR. Biomedical applications of sodium MRI in vivo. J Magn Reson Imaging (2013) 38(3):511–29. doi:10.1002/jmri.24168
14. Hilal S, Maudsley AA, Simon HE, Perman WH, Bonn J, Mawad ME, et al. In vivo NMR imaging of tissue sodium in the intact cat before and after acute cerebral stroke. Am J Neuroradiology (1983) 4(3):245–9.
15. Hilal SK, Maudsley AA, Ra JB, Simon HE, Roschmann P, Wittekoek S, et al. In vivo NMR imaging of sodium-23 in the human head. J Comput Assist tomography (1985) 9(1):1–7. doi:10.1097/00004728-198501000-00001
16. Boada FE, Gillen JS, Noll DC, Shen GX, Thulborn KR. Data acquisition and postprocessing strategies for fast quantitative sodium imaging. Int J Imaging Syst Technol (1997) 8(6):544–50. doi:10.1002/(sici)1098-1098(1997)8:6<544::aid-ima6>3.0.co;2-a
17. Larson PE, Han M, Krug R, Jakary A, Nelson SJ, Vigneron DB, et al. Ultrashort echo time and zero echo time MRI at 7T. Magnetic Resonance Materials in Physics. Biol Med (2016) 29:359–70. doi:10.1007/s10334-015-0509-0
18. Romanzetti S, Mirkes CC, Fiege DP, Celik A, Felder J, Shah NJ. Mapping tissue sodium concentration in the human brain: a comparison of MR sequences at 9.4Tesla. Neuroimage (2014) 96:44–53. doi:10.1016/j.neuroimage.2014.03.079
19. Grodzki DM, Jakob PM, Heismann B. Ultrashort echo time imaging using pointwise encoding time reduction with radial acquisition (PETRA). Magn Reson Med (2012) 67(2):510–8. doi:10.1002/mrm.23017
20. Nagel AM, Laun FB, Weber M, Matthies C, Semmler W, Schad LR. Sodium MRI using a density-adapted 3D radial acquisition technique. Magn Reson Med Official J Int Soc Magn Reson Med (2009) 62(6):1565–73. doi:10.1002/mrm.22157
21. Gurney PT, Hargreaves BA, Nishimura DG. Design and analysis of a practical 3D cones trajectory. Magn Reson Med Official J Int Soc Magn Reson Med (2006) 55(3):575–82. doi:10.1002/mrm.20796
22. Lu A, Atkinson IC, Claiborne TC, Damen FC, Thulborn KR. Quantitative sodium imaging with a flexible twisted projection pulse sequence. Magn Reson Med (2010) 63(6):1583–93. doi:10.1002/mrm.22381
23. Pipe JG, Zwart NR, Aboussouan EA, Robison RK, Devaraj A, Johnson KO. A new design and rationale for 3D orthogonally oversampled k-space trajectories. Magn Reson Med (2011) 66(5):1303–11. doi:10.1002/mrm.22918
24. Bydder M, Samsonov AA, Du J. Evaluation of optimal density weighting for regridding. Magn Reson Imaging (2007) 25(5):695–702. doi:10.1016/j.mri.2006.09.021
25. Tan H, Zheng Y. Point spread function optimization for MRI reconstruction. In: Proceedings.(ICASSP'05). IEEE international conference on acoustics, speech, and signal processing, 2005. IEEE (2005).
26. Gast LV, Platt T, Nagel AM, Gerhalter T. Recent technical developments and clinical research applications of sodium (23Na) MRI. Prog Nucl Magn Reson Spectrosc (2023) 138:1–51. doi:10.1016/j.pnmrs.2023.04.002
27. Qian Y, Panigrahy A, Laymon CM, Lee VK, Drappatz J, Lieberman FS, et al. Short-T2 imaging for quantifying concentration of sodium (23Na) of bi-exponential T2 relaxation. Magn Reson Med (2015) 74(1):162–74. doi:10.1002/mrm.25393
28. Fleysher L, Oesingmann N, Inglese M. B0 inhomogeneity-insensitive triple-quantum-filtered sodium imaging using a 12-step phase-cycling scheme. NMR Biomed (2010) 23(10):1191–8. doi:10.1002/nbm.1548
29. Fleysher L, Oesingmann N, Brown R, Sodickson DK, Wiggins GC, Inglese M. Noninvasive quantification of intracellular sodium in human brain using ultrahigh–field MRI. NMR Biomed (2013) 26(1):9–19. doi:10.1002/nbm.2813
30. Fiege DP, Romanzetti S, Mirkes CC, Brenner D, Shah NJ. Simultaneous single-quantum and triple-quantum-filtered MRI of 23Na (SISTINA). Magn Reson Med (2013) 69(6):1691–6. doi:10.1002/mrm.24417
31. Worthoff WA, Shymanskaya A, Shah NJ. Relaxometry and quantification in simultaneously acquired single and triple quantum filtered sodium MRI. Magn Reson Med (2019) 81(1):303–15. doi:10.1002/mrm.27387
32. Worthoff WA, Shymanskaya A, Lindemeyer J, Langen K, Shah NJ. Relaxometry and quantification in sodium MRI of cerebral gliomas: a FET-PET and MRI small-scale study. NMR Biomed (2020) 33(10):e4361. doi:10.1002/nbm.4361
33. Hubbard PS. Nonexponential nuclear magnetic relaxation by quadrupole interactions. J Chem Phys (1970) 53(3):985–7. doi:10.1063/1.1674167
34. Shporer M, Civan MM. Nuclear magnetic resonance of sodium-23 linoleate-water: basis for an alternative interpretation of sodium-23 spectra within cells. Biophysical J (1972) 12(1):114–22. doi:10.1016/S0006-3495(72)86074-0
35. Berendsen HJ, Edzes HT. The observation and general interpretation of sodium magnetic resonance in biological material. Ann New York Acad Sci (1973) 204(1):459–85. doi:10.1111/j.1749-6632.1973.tb30799.x
36. Andrasko J. Nonexponential relaxation of 23Na+ in agarose gels. J Magn Reson (1969) 16(3):502–4. doi:10.1016/0022-2364(74)90233-9
37. Pekar J, Leigh JS. Detection of biexponential relaxation in sodium-23 facilitated by double-quantum filtering. J Magn Reson (1969) 69(3):582–4. doi:10.1016/0022-2364(86)90180-0
38. Riemer F, Solanky BS, Wheeler-Kingshott CA, Golay X. Bi-exponential 23Na T2* component analysis in the human brain. NMR Biomed (2018) 31(5):e3899. doi:10.1002/nbm.3899
39. Benkhedah N, Bachert P, Nagel AM. Two-pulse biexponential-weighted 23Na imaging. J Magn Reson (2014) 240:67–76. doi:10.1016/j.jmr.2014.01.007
40. Ra JB, Hilal S, Oh C. An algorithm for MR imaging of the short T2 fraction of sodium using the FID signal. J Comput Assist tomography (1989) 13(2):302–9. doi:10.1097/00004728-198903000-00022
41. Lommen JM, Probing the microscopic environment of physiological sodium ions through observation of the T2* relaxation (2007) .
42. Stobbe R, Beaulieu C. In vivo sodium magnetic resonance imaging of the human brain using soft inversion recovery fluid attenuation. Magn Reson Med Official J Int Soc Magn Reson Med (2005) 54(5):1305–10. doi:10.1002/mrm.20696
43. Stobbe R, Boyd A, Smyth P, Emery D, Valdés Cabrera D, Beaulieu C. Sodium intensity changes differ between relaxation-and density-weighted MRI in multiple sclerosis. Front Neurol (2021) 12:693447. doi:10.3389/fneur.2021.693447
44. Benkhedah N, Bachert P, Semmler W, Nagel AM. Three-dimensional biexponential weighted (23)Na imaging of the human brain with higher SNR and shorter acquisition time. Magn Reson Med (2013) 70(3):754–65. doi:10.1002/mrm.24516
45. Gilles A, Nagel AM, Madelin G. Multipulse sodium magnetic resonance imaging for multicompartment quantification: proof-of-concept. Sci Rep (2017) 7(1):17435–19. doi:10.1038/s41598-017-17582-w
46. Ouwerkerk R, Bleich KB, Gillen JS, Pomper MG, Bottomley PA. Tissue sodium concentration in human brain tumors as measured with 23Na MR imaging. Radiology (2003) 227(2):529–37. doi:10.1148/radiol.2272020483
47. Madelin G, Babb J, Xia D, Regatte RR. Repeatability of quantitative sodium magnetic resonance imaging for estimating pseudo-intracellular sodium concentration and pseudo-extracellular volume fraction in brain at 3 T. PloS one (2015) 10(3):e0118692. doi:10.1371/journal.pone.0118692
48. Nunes Neto LP, Madelin G, Sood TP, Wu CC, Kondziolka D, Placantonakis D, et al. Quantitative sodium imaging and gliomas: a feasibility study. Neuroradiology (2018) 60:795–802. doi:10.1007/s00234-018-2041-1
49. Go KG. The normal and pathological physiology of brain water. Adv Tech Stand Neurosurg (1997) 23:47–142. doi:10.1007/978-3-7091-6549-2_2
50. Hagiwara A, Bydder M, Oughourlian T, Yao J, Salamon N, Jahan R, et al. Sodium MR neuroimaging. Am J Neuroradiology (2021) 42(11):1920–6. doi:10.3174/ajnr.a7261
51. Zaric O, Juras V, Szomolanyi P, Schreiner M, Raudner M, Giraudo C, et al. Frontiers of sodium MRI revisited: from cartilage to brain imaging. J Magn Reson Imaging (2021) 54(1):58–75. doi:10.1002/jmri.27326
52. Bruschi N, Boffa G, Inglese M. Ultra-high-field 7-T MRI in multiple sclerosis and other demyelinating diseases: from pathology to clinical practice. Eur Radiol Exp (2020) 4:59–13. doi:10.1186/s41747-020-00186-x
53. Smith KJ. Sodium channels and multiple sclerosis: roles in symptom production, damage and therapy. Brain Pathol (2007) 17(2):230–42. doi:10.1111/j.1750-3639.2007.00066.x
54. Waxman SG. Axonal conduction and injury in multiple sclerosis: the role of sodium channels. Nat Rev Neurosci (2006) 7(12):932–41. doi:10.1038/nrn2023
55. Inglese M, Madelin G, Oesingmann N, Babb JS, Wu W, Stoeckel B, et al. Brain tissue sodium concentration in multiple sclerosis: a sodium imaging study at 3 tesla. Brain (2010) 133(3):847–57. doi:10.1093/brain/awp334
56. Zaaraoui W, Konstandin S, Audoin B, Nagel AM, Rico A, Malikova I, et al. Distribution of brain sodium accumulation correlates with disability in multiple sclerosis: a cross-sectional 23Na MR imaging study. Radiology (2012) 264(3):859–67. doi:10.1148/radiol.12112680
57. Paling D, Solanky BS, Riemer F, Tozer DJ, Wheeler-Kingshott CAM, Kapoor R, et al. Sodium accumulation is associated with disability and a progressive course in multiple sclerosis. Brain (2013) 136(7):2305–17. doi:10.1093/brain/awt149
58. Maarouf A, Audoin B, Konstandin S, Rico A, Soulier E, Reuter F, et al. Topography of brain sodium accumulation in progressive multiple sclerosis. Biol Med (2014) 27:53–62. doi:10.1007/s10334-013-0396-1
59. Eisele P, Konstandin S, Griebe M, Szabo K, Wolf ME, Alonso A, et al. Heterogeneity of acute multiple sclerosis lesions on sodium (23Na) MRI. Mult Scler J (2016) 22(8):1040–7. doi:10.1177/1352458515609430
60. Eisele P, Konstandin S, Szabo K, Ebert A, Roßmanith C, Paschke N, et al. Temporal evolution of acute multiple sclerosis lesions on serial sodium (23Na) MRI. Mult Scler Relat Disord (2019) 29:48–54. doi:10.1016/j.msard.2019.01.027
61. Biller A, Pflugmann I, Badde S, Diem R, Wildemann B, Nagel AM, et al. Sodium MRI in multiple sclerosis is compatible with intracellular sodium accumulation and inflammation-induced hyper-cellularity of acute brain lesions. Scientific Rep (2016) 6(1):31269. doi:10.1038/srep31269
62. Mennecke AB, Nagel AM, Huhn K, Linker RA, Schmidt M, Rothhammer V, et al. Longitudinal sodium MRI of multiple sclerosis lesions: is there added value of sodium inversion recovery MRI. J Magn Reson Imaging (2022) 55(1):140–51. doi:10.1002/jmri.27832
63. Wiggins GC, Brown R, Fleysher L, Zhang B, Stoeckel B, Inglese M, et al. A nested dual frequency birdcage/stripline coil for sodium/proton brain imaging at 7T. In: Proc Intl Soc Mag Reson Med 18th Scientific Meeting (2010), Stockholm, Sweden, May 1–7, 2010:1500.
64. Boada FE, Davis D, Walter K, Torres-Trejo A, Kondziolka D, Bartynski W, et al. Single and triple quantum sodium MRI of primary human brain tumors. In: Proc Intl Soc Mag Reson Med 11th Scientific Meeting (2003), Toronto, Canada, July 10–16, 2010:117.
65. Shen GX, Boada FE, Thulborn KR. Dual-frequency, dual-quadrature, birdcage RF coil design with identical B1 pattern for sodium and proton imaging of the human brain at 1.5 T. Magn Reson Med (1997) 38(5):717–25. doi:10.1002/mrm.1910380507
66. Boada FE, Davis D, Walter K, Torres-Trejo A, Kondziolka D, Bartynski W, et al. Triple quantum filtered sodium MRI of primary brain tumors. In: 2004 2nd IEEE international symposium on biomedical imaging: nano to macro (IEEE cat No. 04EX821). IEEE (2004).
67. Biller A, Badde S, Nagel A, Neumann JO, Wick W, Hertenstein A, et al. Improved brain tumor classification by sodium MR imaging: prediction of IDH mutation status and tumor progression. Am J Neuroradiology (2016) 37(1):66–73. doi:10.3174/ajnr.a4493
68. Bhatia A, Lee VK, Qian Y, Paldino MJ, Ceschin R, Hect J, et al. Quantitative sodium (23Na) MRI in pediatric gliomas: initial experience. Diagnostics (2022) 12(5):1223. doi:10.3390/diagnostics12051223
69. Graham SF, Nasarauddin MB, Carey M, McGuinness B, Holscher C, Kehoe PG, et al. Quantitative measurement of [Na+] and [K+] in postmortem human brain tissue indicates disturbances in subjects with Alzheimer's disease and dementia with Lewy bodies. J Alzheimers Dis (2015) 44(3):851–7. doi:10.3233/jad-141869
70. Vitvitsky VM, Garg SK, Keep RF, Albin RL, Banerjee R. Na+ and K+ ion imbalances in Alzheimer's disease. Biochim Biophys Acta (2012) 1822(11):1671–81. doi:10.1016/j.bbadis.2012.07.004
71. Pannaccione A, Piccialli I, Secondo A, Ciccone R, Molinaro P, Boscia F, et al. The Na(+)/Ca(2+)exchanger in Alzheimer's disease. Cell Calcium (2020) 87:102190. doi:10.1016/j.ceca.2020.102190
72. Haeger A, Bottlaender M, Lagarde J, Porciuncula Baptista R, Rabrait-Lerman C, Luecken V, et al. What can 7T sodium MRI tell us about cellular energy depletion and neurotransmission in Alzheimer's disease? Alzheimer's and Demen (2021) 17(11):1843–54. doi:10.1002/alz.12501
73. Mellon EA, Pilkinton D, Clark C, Elliott M, Witschey W, Borthakur A, et al. Sodium MR imaging detection of mild Alzheimer disease: preliminary study. AJNR Am J Neuroradiol (2009) 30(5):978–84. doi:10.3174/ajnr.a1495
74. Boada FE, Qian Y, Nemoto E, Jovin T, Jungreis C, Jones SC, et al. Sodium MRI and the assessment of irreversible tissue damage during hyper-acute stroke. Translational stroke Res (2012) 3:236–45. doi:10.1007/s12975-012-0168-7
75. Thulborn KR, Davis D, Snyder J, Yonas H, Kassam A. Sodium MR imaging of acute and subacute stroke for assessment of tissue viability. Neuroimaging Clin North America (2005) 15(3):639–53, xi. doi:10.1016/j.nic.2005.08.003
76. Adlung A, Licht C, Reichert S, Özdemir S, Mohamed SA, Samartzi M, et al. Quantification of tissue sodium concentration in the ischemic stroke: a comparison between external and internal references for 23Na MRI. J Neurosci Methods (2022) 382:109721. doi:10.1016/j.jneumeth.2022.109721
77. Adlung A, Paschke NK, Golla A, Bauer D, Mohamed SA, Samartzi M, et al. 23Na MRI in ischemic stroke: acquisition time reduction using postprocessing with convolutional neural networks. NMR Biomed (2021) 34(4):e4474. doi:10.1002/nbm.4474
78. Bernhardt BC, Rozen DA, Worsley KJ, Evans AC, Bernasconi N, Bernasconi A. Thalamo-cortical network pathology in idiopathic generalized epilepsy: insights from MRI-based morphometric correlation analysis. Neuroimage (2009) 46(2):373–81. doi:10.1016/j.neuroimage.2009.01.055
79. Liu RS, Lemieux L, Bell GS, Sisodiya SM, Bartlett PA, Shorvon SD, et al. Cerebral damage in epilepsy: a population-based longitudinal quantitative MRI study. Epilepsia (2005) 46(9):1482–94. doi:10.1111/j.1528-1167.2005.51603.x
80. Azilinon M, Makhalova J, Zaaraoui W, Medina Villalon S, Viout P, Roussel T, et al. Combining sodium MRI, proton MR spectroscopic imaging, and intracerebral EEG in epilepsy. Hum Brain Mapp (2023) 44(2):825–40. doi:10.1002/hbm.26102
81. Fisher RS, Scharfman HE, deCurtis M. How can we identify ictal and interictal abnormal activity? Adv Exp Med Biol (2014) 813:3–23. doi:10.1007/978-94-017-8914-1_1
82. Ridley B, Marchi A, Wirsich J, Soulier E, Confort-Gouny S, Schad L, et al. Brain sodium MRI in human epilepsy: disturbances of ionic homeostasis reflect the organization of pathological regions. Neuroimage (2017) 157:173–83. doi:10.1016/j.neuroimage.2017.06.011
83. Azilinon M, Wang HE, Makhalova J, Zaaraoui W, Ranjeva JP, Bartolomei F, et al. Brain sodium MRI-derived priors support the estimation of epileptogenic zones using personalized model-based methods in Epilepsy. Netw Neurosci (2024) 8:673–96. doi:10.1162/netn_a_00371
84. Grover H, Qian Y, Boada F, Lakshmanan K, Flanagan S, Lui Y. MRI evidence of altered callosal sodium in mild traumatic brain injury. Am J Neuroradiology (2018) 39(12):2200–4. doi:10.3174/ajnr.a5903
85. Gerhalter T, Chen AM, Dehkharghani S, Peralta R, Adlparvar F, Babb JS, et al. Global decrease in brain sodium concentration after mild traumatic brain injury. Brain Commun (2021) 3(2):fcab051. doi:10.1093/braincomms/fcab051
86. Gerhalter T, Chen AM, Dehkharghani S, Peralta R, Gajdosik M, Zarate A, et al. Longitudinal changes in sodium concentration and in clinical outcome in mild traumatic brain injury. Brain Commun (2024) 6(4):fcae229. doi:10.1093/braincomms/fcae229
87. Leslie TK, James AD, Zaccagna F, Grist JT, Deen S, Kennerley A, et al. Sodium homeostasis in the tumour microenvironment. Biochim Biophys Acta Rev Cancer (2019) 1872(2):188304. doi:10.1016/j.bbcan.2019.07.001
88. Vargová L, Homola A, Zámečník J, Tichý M, Beneš V, Syková E. Diffusion parameters of the extracellular space in human gliomas. Glia (2003) 42(1):77–88. doi:10.1002/glia.10204
89. Kline RP, Wu EX, Petrylak DP, Szabolcs M, Alderson PO, Weisfeldt ML, et al. Rapid in vivo monitoring of chemotherapeutic response using weighted sodium magnetic resonance imaging. Clin Cancer Res (2000) 6(6):2146–56.
90. S AM, Adlung A, Ruder AM, Hoesl MAU, Schad L, Groden C, et al. MRI detection of changes in tissue sodium concentration in brain metastases after stereotactic radiosurgery: a feasibility study. J Neuroimaging (2021) 31(2):297–305. doi:10.1111/jon.12823
91. Thulborn KR, Davis D, Adams H, Gindin T, Zhou J. Quantitative tissue sodium concentration mapping of the growth of focal cerebral tumors with sodium magnetic resonance imaging. Magn Reson Med Official J Int Soc Magn Reson Med (1999) 41(2):351–9. doi:10.1002/(sici)1522-2594(199902)41:2<351::aid-mrm20>3.3.co;2-8
92. Boada FE, Qian Y, Davis D, Lieberman F, Hamilton R, Mint A, Schwartz E, et al. Sodium MRI and 1H MRS in the diagnosis and monitoring of primary brain tumors. In: Proc Intl Soc Mag Reson Med 17th Scientific Meeting (2009), Honolulu, HI, United States, April 18–24, 2009:149.
93. Kim S, Merugumala S, Lin AP. A uniformity correction method to reduce scan time for 7T sodium imaging of brain tumors. J Neuroimaging (2022) 32(6):1062–9. doi:10.1111/jon.13041
94. Huang L, Zhang Z, Qu B, Cui Z, Wang Y, Li J, et al. Imaging of sodium MRI for therapy evaluation of brain metastase with Cyberknife at 7T: a case report. Cureus (2018) 10(4):e2502. doi:10.7759/cureus.2502
95. Poku LO, Phil M, Cheng Y, Wang K, Sun X. 23Na-MRI as a noninvasive biomarker for cancer diagnosis and prognosis. J Magn Reson Imaging (2021) 53(4):995–1014. doi:10.1002/jmri.27147
96. Fiege DP, Development and application of multiple-quantum coherence techniques for in vivo sodium MRI at high and ultra-high field strengths (2014) .
97. Burstein D, Springer CS. Sodium MRI revisited. Magn Reson Med (2019) 82(2):521–4. doi:10.1002/mrm.27738
98. Wiggins GC, Brown R, Lakshmanan K. High-performance radiofrequency coils for 23Na MRI: brain and musculoskeletal applications. NMR Biomed (2016) 29(2):96–106. doi:10.1002/nbm.3379
99. Bangerter NK, Kaggie JD, Taylor MD, Hadley JR. Sodium MRI radiofrequency coils for body imaging. NMR Biomed (2016) 29(2):107–18. doi:10.1002/nbm.3392
100. Giovannetti G, Flori A, Martini N, Francischello R, Aquaro GD, Pingitore A, et al. Sodium radiofrequency coils for magnetic resonance: from design to applications. Electronics (2021) 10(15):1788. doi:10.3390/electronics10151788
101. Wilferth T, Mennecke A, Gast LV, Lachner S, Müller M, Rothhammer V, et al. Quantitative 7T sodium magnetic resonance imaging of the human brain using a 32-channel phased-array head coil: application to patients with secondary progressive multiple sclerosis. NMR Biomed (2022) 35(12):e4806. doi:10.1002/nbm.4806
102. Benkhedah N, Hoffmann SH, Biller A, Nagel AM. Evaluation of adaptive combination of 30-channel head receive coil array data in 23 N a MR imaging. Magn Reson Med (2016) 75(2):527–36. doi:10.1002/mrm.25572
103. Shajan G, Mirkes C, Buckenmaier K, Hoffmann J, Pohmann R, Scheffler K. Three-layered radio frequency coil arrangement for sodium MRI of the human brain at 9.4 Tesla. Magn Reson Med (2016) 75(2):906–16. doi:10.1002/mrm.25666
104. Kratzer FJ, Flassbeck S, Nagel AM, Behl NG, Knowles BR, Bachert P, et al. Sodium relaxometry using 23Na MR fingerprinting: a proof of concept. Magn Reson Med (2020) 84(5):2577–91. doi:10.1002/mrm.28316
105. Yu Z, Madelin G, Sodickson DK, Cloos MA. Simultaneous proton magnetic resonance fingerprinting and sodium MRI. Magn Reson Med (2020) 83(6):2232–42. doi:10.1002/mrm.28073
106. Kratzer FJ, Flassbeck S, Schmitter S, Wilferth T, Magill AW, Knowles BR, et al. 3D sodium (23Na) magnetic resonance fingerprinting for time-efficient relaxometric mapping. Magn Reson Med (2021) 86(5):2412–25. doi:10.1002/mrm.28873
107. Rodriguez GG, Yu Z, Shaykevich S, O'Donnell LF, Aguilera L, Cloos MA, et al. Super-resolution of sodium images from simultaneous 1H MRF/23Na MRI acquisition. NMR Biomed (2023) 36(10):e4959. doi:10.1002/nbm.4959
108. Hellerbach A, Schuster V, Jansen A, Sommer J. MRI phantoms–are there alternatives to agar? PloS one (2013) 8(8):e70343. doi:10.1371/journal.pone.0070343
Keywords: sodium MRI, 23Na MRI, brain, compartmentalization, neurological diseases, biomarkers, modeling
Citation: Egidi I, Guidi M and Giove F (2025) Compartmentalization of sodium in the human brain: a mini-review of 23Na-MRI methods. Front. Phys. 13:1487822. doi: 10.3389/fphy.2025.1487822
Received: 28 August 2024; Accepted: 24 March 2025;
Published: 23 April 2025.
Edited by:
Silvia Capuani, National Research Council (CNR), ItalyReviewed by:
Imran Iqbal, New York University, United StatesGisela E. Hagberg, University Hospital and Faculty of Medicine, University of Tübingen, Germany
Copyright © 2025 Egidi, Guidi and Giove. This is an open-access article distributed under the terms of the Creative Commons Attribution License (CC BY). The use, distribution or reproduction in other forums is permitted, provided the original author(s) and the copyright owner(s) are credited and that the original publication in this journal is cited, in accordance with accepted academic practice. No use, distribution or reproduction is permitted which does not comply with these terms.
*Correspondence: Federico Giove, ZmVkZXJpY28uZ2lvdmVAdW5pcm9tYTEuaXQ=