- 1Ceyear Technologies Co., Ltd., Qingdao, China
- 2Science and Technology on Electronic Test and Measurement Laboratory, Qingdao, China
- 3Shandong Electronic Test and Measurement Technology Innovation Center, Qingdao, China
A wide mode-hop-free and narrow-linewidth tunable laser diode source employing a Littman-Metcalf configuration with a diffraction grating is developed. We conducted a series of experiments to evaluate the tuning characteristics and spectral linewidth of the proposed external-cavity diode laser. A continuous, mode-hop-free wavelength tuning range of approximately 180 nm within the S+C+L band is achieved, with a spectral linewidth below 32.95 kHz. Moreover, the device maintains an optical signal-to-noise ratio exceeding 62.60 dB and an output power above 10.11 dBm over the entire tuning range under long-term free-running conditions. This tunable laser, offering both mode-hop-free operation and narrow-linewidth output, holds significant promise for practical applications such as coherent detection.
1 Introduction
Due to the inherent limitations of laser gain media, which typically exhibit fixed emission spectra, the versatility of laser systems is fundamentally constrained. Consequently, broadening the accessible wavelength range and achieving precise wavelength tuning have emerged as critical areas in laser research [1–4]. Tunable-wavelength lasers, capable of flexible output adjustment, are critical for applications such as spectral detection, fiber optic sensing, remote sensing, medical science, and high-resolution spectroscopy [5–11]. Moreover, attaining fine wavelength tuning alongside narrow spectral linewidths is essential for optimizing performance in precision-demanding areas such as coherent optical communications, laser-based metrology, and detailed spectroscopic analysis [12–15]. Among various light sources, wavelength-tunable semiconductor lasers (WTSLs) have attracted significant attention due to their broad spectral coverage, precise wavelength tuning, and stable spectral output. These features make WTSLs ideal for applications such as gas detection, high-order quadrature amplitude modulation (QAM) coherent transmission, optical fiber sensing, and high-resolution spectroscopy [16–20]. Consequently, extensive research efforts have been dedicated to developing WTSLs with enhanced performance [21–24].
To date, demonstrations of WTSLs covering the full C-band, L-band, and even the combined C + L band have been achieved using techniques such as sampled-grating distributed Bragg reflectors (SG DBR), dispersion tuning, and external-cavity feedback [25–27]. Among these, the external-cavity feedback configuration is the most widely used [28, 29]. Moreover, various mode-selection filters—including blazed diffraction gratings [25], interference filters (IF) [30], and acousto-optic tunable filters (AOTF) [31]—have been integrated into external-cavity structures to further enhance performance. For instance, Zhang et al. demonstrated a compact MEMS-based WTSL operating at the C-band with a tuning range of approximately 40 nm (1528.77–1568.36 nm), achieving a wavelength accuracy of ±1 GHz and a spectral linewidth below 50 kHz [32]. Similarly, Wang et al. reported a WTSL covering 1525–1565 nm using a wavelength-scanning filter based on an IF and diffraction grating, which yielded a maximum side-mode suppression ratio (SMSR) of about 57 dB at 1555 nm and a Lorentz linewidth under 13.6 kHz [33]. The rapid growth of silicon photonic integrated circuits (PICs) has also spurred novel designs [34]. For example, Chen et al. presented a hybrid integrated Si3N4 WTSL with dual micro-ring external-cavity feedback, achieving a 55 nm tuning range and a linewidth below 8 kHz [35]. In addition, diffraction-grating configurations—such as the Littrow and Littman-Metcalf setups—have been effectively applied for wavelength selection and beam feedback. Guo et al. demonstrated an enhanced mirror-grating Littrow-type configuration at 805 nm that maintained constant lateral output beam displacement and provided a mode-hop-free tuning range of 4.34 nm [36]. Other notable works include an InGaAs-InP strained multiple quantum well (MQW) WTSL covering 1,494–1,667 nm with a linewidth of approximately 0.4 nm [37], a traditional Littman-Metcalf configuration spanning 797.38–807.26 nm [38], and a mode-hop-free design offering a 100 nm tuning range (1,480–1,580 nm) with a minimum SMSR of 65.54 dB [24]. Despite these advances, most studies on external-cavity optical feedback WTSLs have focused on the C-band, L-band, C+L-band, or S + C-band, while mode-hop-free operation over the combined S+C+L bands has received less attention. Addressing this gap is therefore of significant value.
Here, we propose an external-cavity optical feedback WTSL that achieves a wide, mode-hop-free tuning range from 1,450 nm to 1,630 nm—covering the telecommunication windows S (1,460–1,530 nm), C (1,530–1,565 nm), and L (1,565–1,625 nm), which is about 77.8% times enhancement compared with [26]. A commercially available bare chip serves as the gain medium and is self-packaged as an airtight module. A tuning mirror integrated with a blazed grating functions as both the mode filter and the source of optical feedback. Experimental results confirm that the proposed WTSL operates in a single-longitudinal mode with a spectral linewidth below 32.95 kHz. Additionally, the SMSR measured throughout the entire S + C + L band reaches a maximum of 71.03 dB at 1590 nm, and a peak output power of approximately 15.82 dBm is achieved.
2 Experimental setup
Figure 1 highlights the schematic diagram of our proposed WTSL. It can be divided into two major parts, namely, the internal-cavity part for optical gain and the external-cavity part for mode selection. The internal-cavity generally contains a single angled facet gain chip (GC) with a 3 dB bandwidth more than 100 nm, which is used to provide a large gain bandwidth with low ripple. One edge (or called normal facet, L1) is 10% reflection-coated (corresponding loss: 0.2 dB), and the other facet of the GC (R1) is 0.005% AR-coated. The external-cavity includes a commercially available molded aspherical lens (F1) with a focusing length of 4.51 mm, a diffractive grating (G1) with 900 lines per mm and about 75% average diffraction efficiency (1st-order), a gold-coated tuning mirror (M1), and a brushless direct current motor (DC1) with maximum rotating speed of 24,000 revolutions per minute (RPM). All the fiber components used in our scheme are panda-style polarization-maintaining (PM) fiber pigtails. A stable continuous wave (CW) output from the R1 edge of the GC (Thorlabs, SAF1150S2) is shaped by the F1 (Edmund, #87-155), the collimated laser beam is diffracted on the surface of the G1 (Newport Richardson, 33025FL01-155R), and then feedbacked into the GC along the original optical path to generate a high spectral purity laser beam. Finally, the emission wavelength-tunable is output from the normal facet of the GC, which is controlled by rotating the gold mirror driven by the DC1 located outside the external-cavity, and coupled into a PM-fiber collimator. The output fiber is connected to a PM-fiber isolator followed by a 1 × 2 (50:50) optical coupler (OC) feeding into an optical power meter (OPM) and a wavelength meter (Yokogawa, AQ6151B) with a wavelength accuracy of ±0.2 ppm. For subsequent wavelength-related characteristics measurements, the OPM (Thorlabs, PM100D) is replaced by an optical spectrum analyzer (OSA, 6362DA) with 10 p.m. resolution fabricated by Ceyear. For intrinsic linewidth measurements of the proposed WTSL, the output going to the 3 dB OC is instead connected to a commercially available linewidth measurement tool (SYCATUS, A0040A). The main cavity length is about 0.5 cm, the external air cavity length from the R1 to the G1 is about 0.3 cm, and from the G1 to the M1 is about 0.2 cm, so the total length of the WTSL is about 0.5 cm. Therefore, the corresponding longitudinal mode spacing is about 3 GHz@1550 nm. Meanwhile, all the cavity parts are packaged in a metal housing, where the temperature is controlled by a 7.56 W thermoelectric cooler (TEC). With this geometry, a stable single-longitudinal-mode wavelength-tunable lasing output can be obtained.
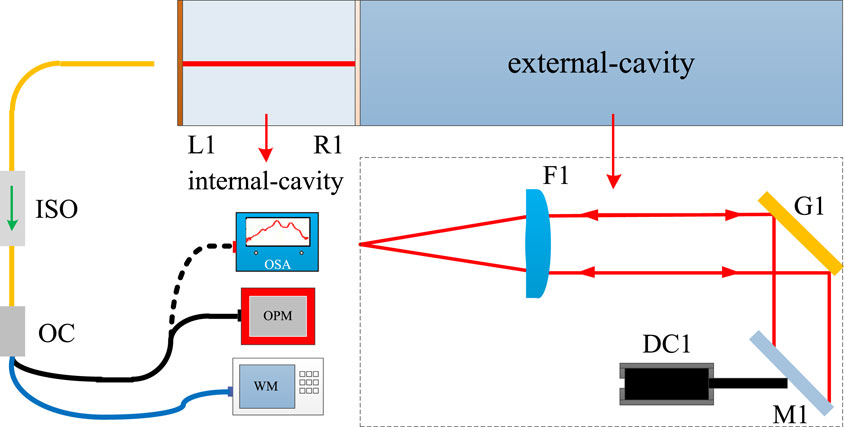
Figure 1. Schematic diagram of our proposed WTSL. F1, molded aspherical lens; OC, optical coupler; ISO, isolator; G1, diffractive grating; DC1, direct current motor; OPM, optical power meter; WM, wavelength meter; OSA, optical spectrum analyzer; M1, gold-coated tuning mirror.
3 Experimental results and discussion
After adding the optimized external-cavity part to the internal-cavity part, the output power of the proposed WTSL versus the drive current is firstly measured at different resonant wavelengths and shown in Figure 2A. Optimum alignment is accomplished when the waist position of the airtight module is adjusted to equal the length of the external-cavity [27]. It can be seen from Figure 2A that the threshold current at 1,450 nm, 1,540 nm, and 1,630 nm resonant wavelengths are 260 mA, 70 mA and 270 mA, respectively. As shown in Figure 2A, the measured results are halved due to the use of the 3 dB optical coupler. Therefore, the corresponding output power PStart, PCenter and Pend are 10.26 mW (10.11 dBm), 38.22 mW (15.82 dBm) and 10.44 mW (10.19 dBm) at the drive current of 400 mA, respectively. Obviously, the output power over the entire tuning range exceeds 10.11 dBm, and a peak output power of about 15.82 dBm is obtained at 1,540 nm. Meanwhile, it is worth noting that there is a mode-hopping point (or called mode-hopping region) from 430 mA to 440 mA. This phenomenon may result from current-induced adjacent mode jump. In addition, when the drive current exceeds about 450 mA, the output power of the tunable laser source tends to be saturated. Therefore, we set the operating current of the proposed WTSL to 400 mA to realize the optimal output characteristics. Figures 2B–D show the resonant wavelength of the WTSL as a function of the drive current, respectively, which indicates the threshold current are consistent with the results in Figure 2A, and the spectral coverage can be obtained from 1449.917 nm to 1630.0986 nm. Figures 2E–G plot the relationship between the output wavelength characteristics of the presented WTSL and the drive current when the injection current is in the range of 270 mA–500 mA with 10 mA per step, which indicates the slope efficiency are approximately 3.1 p.m./mA, 2.8 p.m./mA and 3.4 p.m./mA, respectively. From Figure 2E, it can be observed that the proposed laser source has a mode-hopping region, which corresponds exactly to the abrupt of the output power in Figure 2A.
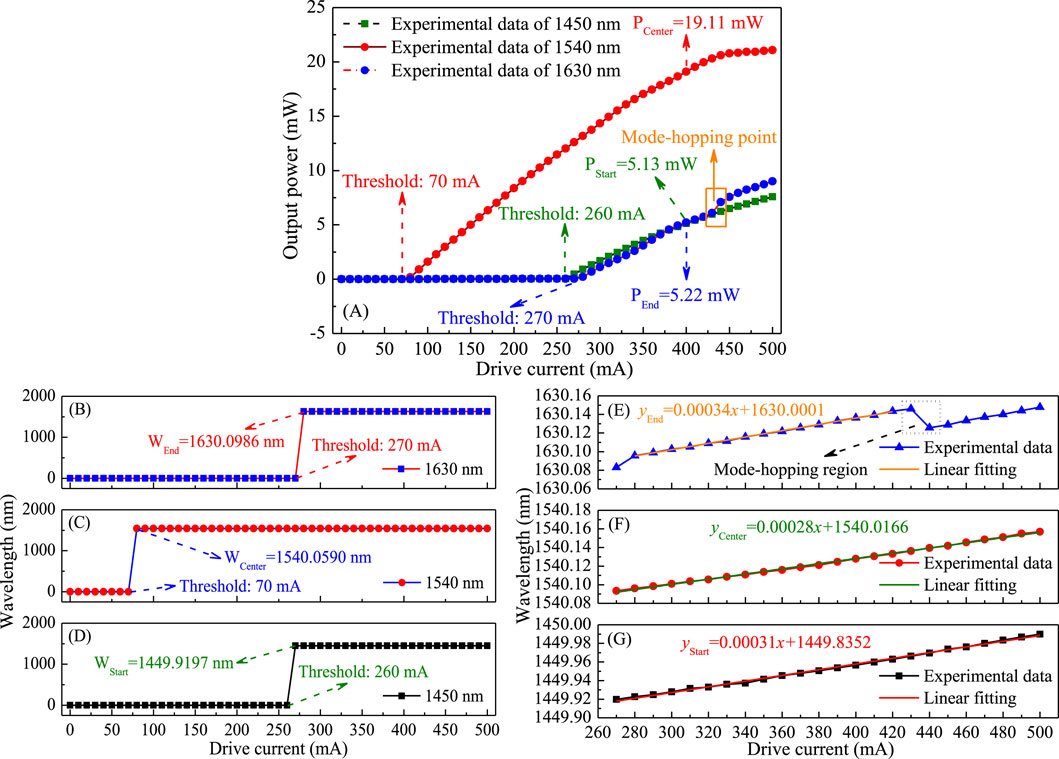
Figure 2. (A) P-I plot of the proposed WTSL operating at 1,480 nm, 1,540 nm, and 1,630 nm, respectively. (B–D). The resonant wavelength of the WTSL as a function of the drive current. (E–G) The relationship between the output wavelength characteristics of the proposed WTSL and the drive current.
Figure 3A is the superimposed output optical spectra of the proposed WTSL at an injection current of 400 mA. The single-longitudinal-mode laser beam for different resonance wavelengths are tuned by adjusting the physical lengths of the external-cavity rotated around the pivot point, and the peaks in the superimposed output optical spectra correspond to different DC1 (tuning mirror) positions. As highlighted in Figure 3A, the corresponding result shows a wide spectral coverage of about 180 nm, namely, it can be adjusted from 1,450 nm to 1,630 nm with about 10 nm per step. Additionally, as can be observed from Figure 3B, the presented tunable semiconductor laser source keeps excellent SMSR output performance during the entire scanning range when the drive current remains about 400 mA. The maximum and minimum SMSR (Smax and Smin) of the proposed WTSL are measured to be 71.03 dB and 62.60 dB, respectively. In the case of the drive current of about 400 mA, the SMSR over the whole tuning range of more than 62.60 dB can be achieved.
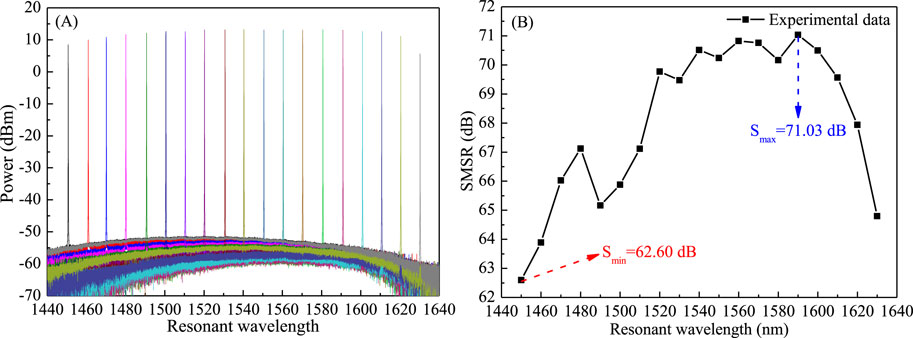
Figure 3. (A) The superimposed output optical spectra of the proposed WTSL at different resonance wavelengths. (B) The SMSR versus the resonant output wavelength at a drive current of about 400 mA.
In our designed external-cavity configuration, the minimum axial mode spacing is close to 20.3 p.m., as depicted in Figure 2E, which is due to the physical length of the WTSL cavity of about 67 mm at 1650 nm. In particular, the larger the axial mode spacing, the smaller the physical length of the external-cavity, and the corresponding resonant output wavelength will be in the short-wavelength region. It can be seen from Figure 4 that the maximum and minimum deviation of the adjacent output wavelength (Dmax and Dmin) are estimated to be 4.1 p.m. and −5.7 p.m., respectively. Because the maximum absolute value of the wavelength differential (Dmin) is much smaller than the minimum axial mode spacing, this indicates that our proposed laser structure can realize mode-hopping free output throughout the full band [39].
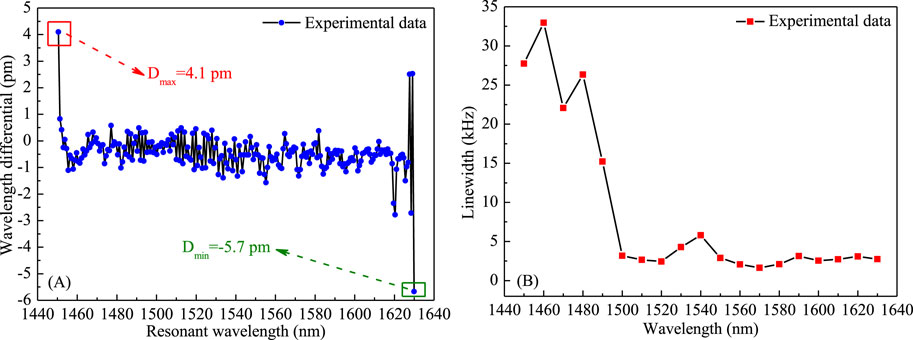
Figure 4. (A) The mode-hopping free performance of the proposed WTSL. (B) The relationship between the intrinsic linewidth of the proposed tunable laser source and the lasing wavelength.
Finally, the relationship between the intrinsic linewidth of the proposed tunable laser source and the lasing wavelength is measured using a commercial optical noise analyzer which is fabricated by the SYCATUS. The measured spectra will be Lorentz line-type. Owing to the assembly capacity of the external-cavity, the temperature control capability, and low-noise driving capability, as plotted in Figure 4B, the intrinsic linewidth of the output resonant wavelength is no more than 32.95 kHz in the entire mode-hopping-free tunable region, and when the lasing output wavelength is about 1,570 nm, an ultra-narrow intrinsic linewidth of about 1.63 kHz can be obtained. Significantly, the blazed grating plays a major role in the compression of the linewidth. Therefore, in the next step, we will carry out the study of blazed grating parameters on the output characteristics of WTSL.
4 Conclusion
In conclusion, we designed and experimentally demonstrated a mode-hop-free, wavelength-tunable semiconductor laser operating over the S+C+L band with external-cavity optical feedback. The external cavity, based on a Littman configuration and comprised of a ruled grating and a tuning mirror, serves as the mode selector. Our experimental results show that the output wavelength of the proposed laser can be continuously tuned from 1,450 nm to 1,630 nm, achieving a side-mode suppression ratio (SMSR) of 71.03 dB at 1590 nm and an intrinsic linewidth of 1.63 kHz at 1,570 nm. Notably, the laser maintains mode-hop-free operation across the entire tuning range. These outstanding performance metrics indicate that the proposed laser configuration holds significant potential for high-capacity coherent communication systems, including those operating at 1.6 Tbit/s or even 3.2 Tbit/s.
Data availability statement
The raw data supporting the conclusions of this article will be made available by the authors, without undue reservation.
Author contributions
LS: Conceptualization, Funding acquisition, Resources, Writing – original draft, Writing – review and editing. ZL: Formal Analysis, Funding acquisition, Investigation, Writing – review and editing. JW: Formal Analysis, Writing – review and editing. LL: Software, Writing – review and editing. BY: Validation, Writing – review and editing. ZZ: Software, Writing – review and editing. SZ: Validation, Writing – review and editing. LH: Conceptualization, Funding acquisition, Methodology, Software, Writing – original draft, Writing – review and editing.
Funding
The author(s) declare that financial support was received for the research and/or publication of this article. This research was funded by National Natural Science Foundation of China, grant number U23B2046; National Key R&D Program of China, grant numbers 2022YFF0705900 and 2022YFF0707102.
Acknowledgments
Thanks to Yu Wei and Ce Zheng for their support during the experiments.
Conflict of interest
Authors LS, ZL, JW, LL, BY, ZZ, SZ, and LH were employed by Ceyear Technologies Co., Ltd.
Generative AI statement
The author(s) declare that no Generative AI was used in the creation of this manuscript.
Publisher’s note
All claims expressed in this article are solely those of the authors and do not necessarily represent those of their affiliated organizations, or those of the publisher, the editors and the reviewers. Any product that may be evaluated in this article, or claim that may be made by its manufacturer, is not guaranteed or endorsed by the publisher.
References
1. Qi Y, Li J, Zhang Y, Yan D, Chang F, Yu X, et al. Research progress of all-solid-state intra-cavity vortex beam generation in visible laser (invited). Infrared Laser Eng (2023) 52(8):20230424. doi:10.3788/IRLA20230424
2. Chen H, Bai Z, Chen J, Li X, Zhu Z, Wang Y, et al. Diamond Raman vortex lasers. ACS Photon (2025) 12(2):864–9. doi:10.1021/acsphotonics.4c01852
3. Sheng L, Ba D, Lv Z. Low-noise and high-gain of stimulated Brillouin amplification via orbital angular momentum mode division filtering. Appl Opt (2019) 58(1):147–51. doi:10.1364/AO.58.000147
4. Chen H, Cui Y, Li X, Zhang B, Cai Y, Ding J, et al. High-power dual-wavelength intracavity diamond Raman laser. Funct Diamond (2023) 3(1):2282527. doi:10.1080/26941112.2023.2282527
5. Lv Z, Liu Z, Chen H, Jin D, Hao X, Fan W, et al. Review of multi-wavelength laser technology based on crystalline Raman conversion (invited). Infrared Laser Eng (2023) 52(8):20230420. doi:10.3788/IRLA20230420
6. Li K, Song C, Yue J, Jia M, Xu Z, Wu D, et al. 亚纳秒Zig-Zag板条激光器实现500 Hz焦耳量级输出. Infrared Laser Eng (2023) 52(8):20230423. doi:10.3788/IRLA20230423
7. Sun T, Guo Y, Wang T, Huo J, Zhang L. Widely tunable wavelength spacing dual-wavelength single longitudinal mode erbium doped fiber laser. Opt Fiber Technology (2014) 20(3):235–8. doi:10.1016/j.yofte.2014.02.006
8. Swain K, Das S, Samal S, Sahu S, Palai G. Realization of 20 monochromatic laser beams using a photonic crystal (PhC) structure via the principle of filtering. Lasers Eng (2023) 54:39–49. doi:10.21203/rs.3.rs-697176/v1
9. Amiri I, Sahoo S, Palai G, Tripathy S. Generation of ‘16’ type of biomedical laser using a single photonic structure: a new paradigm to operation in medical science. Optik (2019) 197:163227. doi:10.1016/j.ijleo.2019.163227
10. Nayak S, Panigrahi P, Palai G, Satpathy R. Optimization of optical communication using optical fiber at tri-optical windows. Comput Commun Intelligence (2025) 279–82. doi:10.1201/9781003581215-57
11. Misra S, Bala I, Swain K, Satpathy R, Palai G, Mishra C. Optimization of microstructred fiber’s mode distribution for high speed data transmission. J Opt (2024) 3(5):1–11. doi:10.1007/s12596-024-01881-3
12. Bai Z, Zhao Z, Tian M, Jin D, Pang Y, Li S, et al. A comprehensive review on the development and applications of narrow-linewidth lasers. Microwave Opt Tech Lett (2022) 64(12):2244–55. doi:10.1002/mop.33046
13. Villanueva G, Perez-Millan P, Palací J, Cruz J, Andrés M, Marti J. Dual-wavelength DFB erbium-doped fiber laser with tunable wavelength spacing. IEEE Photon Technology Lett (2010) 22(4):254–6. doi:10.1109/LPT.2009.2038594
14. Al-Taiy H, Wenzel N, Preußler S, Klinger J, Schneider T. Ultra-narrow linewidth, stable and tunable laser source for optical communication systems and spectroscopy. Opt Lett (2014) 39(20):5826–9. doi:10.1364/OL.39.005826
15. Chen H, Zhao Z, Fan W, Cai Y, Zhang B, Ding J, et al. High-power, ultra-low-noise cascaded diamond Raman lasers with spectrum compression. High Power Laser Sci Eng (2024) 12:e82. doi:10.1017/hpl.2024.68
16. Lou X, Feng Y, Chen C, Dong Y. Multi-point spectroscopic gas sensing based on coherent FMCW interferometry. Opt Express (2020) 28(6):9014–26. doi:10.1364/OE.389746
17. Ma Y, Yang Q, Tang Y, Chen S, Shieh W. 1-Tb/s single-channel coherent optical OFDM transmission over 600-km SSMF fiber with subwavelength bandwidth access. Opt Express (2009) 17(28):9421–7. doi:10.1364/OE.17.009421
18. Xu P, Yu X, Chen Z, Sheng L, Liu J, Zhou S, et al. Distributed refractive index sensing based on bending-induced multimodal interference and Rayleigh backscattering spectrum. Opt Express (2021) 29(14):21530–8. doi:10.1364/OE.430637
19. Sheng L, Huang L, Yan J, Qiao S, Zhang A, Jin H, et al. Distributed multi-parameter sensing based on the Brillouin scattering effect in orbital angular momentum guiding fiber. Opt Continuum (2022) 1(1):133–42. doi:10.1364/OPTCON.446952
20. Doringsho K, Ernsting I, Rinkle R, Schiller S, Wicht A. Low-noise, tunable diode laser for ultra-high-resolution spectroscopy. Opt Lett (2007) 32(413):2876–8. doi:10.1364/ol.32.002876
21. Zhang A, Qiao S, Sheng L, Huang L, Liu Z, Ju J, et al. Study on external cavity diode laser with a wide mode-hopping free tuning range. Fron Phys (2022) 10:1093179. doi:10.3389/fphy.2022.1093179
22. Harvey K, Myatt C. External-cavity diode laser using a grazing-incidence diffraction grating. Opt Lett (1991) 16(413):910–2. doi:10.1364/ol.16.000910
23. Hawthorn C, Weber K, Scholten R. Littrow configuration tunable external cavity diode laser with fixed direction output beam. Rev Sci Instrum (2001) 72(413):4477–9. doi:10.1063/1.1419217
24. Sheng L, Ge C, Cao Q, Huang L, Zhao Z, Li L, et al. Wide-range external-cavity tunable semiconductor laser with mode-hopping free. Infrared Laser Eng (2023) 52(8):20230374. doi:10.3788/IRLA20230374
25. Larson M, Bhardwaj A, Xiong W, Feng Y, Huang X, Petrov K, et al. Narrow linewidth sampled-grating distributed Bragg reflector laser with enhanced side-mode suppression. In: In proceedings of optical fiber communication conference (2015). p. M2D–1. doi:10.1364/OFC.2015.M2D.1
26. Kasai K, Nakazawa M, Tomomatsu Y, Endo T. 1.5μm, mode-hop-free full C-band wavelength tunable laser diode with a linewidth of 8 kHz and a RIN of -130 dB/Hz and its extension to the L-band. Opt Express (2017) 25(18):22113–24. doi:10.1364/OE.25.022113
27. Sun J, Qiu L, Liu L, Sheng L, Cui Y, Huang L, et al. Output characteristics of external-cavity mode-hop-free tunable laser source in C+L band. Photonics (2024) 11:677. doi:10.3390/photonics11070677
28. Gong H, Liu Z, Zhou Y, Zhang W. Extending the mode-hop-free tuning range of an external-cavity diode laser by synchronous tuning with mode matching. Appl Opt (2014) 53(33):7878–84. doi:10.1364/AO.53.007878
29. Sheng L, Wang J, Huang L, Zhang A, Zhang Z, Qiao S, et al. Advances in narrow linewidth and wide tuning range external-cavity wavelength-swept lasers. Fron Phys (2024) 12:1380905. doi:10.3389/fphy.2024.1380905
30. Zhang L, Liu T, Chen L, Xu G, Jiang C, Liu J, et al. Development of an interference filter-stabilized external-cavity diode laser for space applications. Photonics (2020) 7(12):7010012. doi:10.3390/photonics7010012
31. Magdich L, Chamorovskiy A, Shidlovsky V, Shramenko M, Yakubovich S. Tunable semiconductor laser with two acousto-optic tunable filters in its external cavity. Quan Electron (2020) 50(2):136–40. doi:10.1070/QEL17180
32. Zhang D, Zhao J, Yang Q, Liu W, Fu Y, Li C, et al. Compact MEMS external cavity tunable laser with ultra-narrow linewidth for coherent detection. Opt Express (2012) 20(18):19670–82. doi:10.1364/oe.20.019670
33. Wang Y, Ding K, Wu H, Zhao T, Wu Y, Cui Q, et al. Tunable narrow linewidth external cavity diode laser employing wide interference filter and diffraction grating. Appl Sci (2023) 13:10790. doi:10.3390/app131910790
34. Malik A, Guo J, Tran M, Kurczveil G, Liang D, Bowers J. Widely tunable, heterogeneously integrated quantum-dot O-band lasers on silicon. Photon Res (2020) 8(10):1551–7. doi:10.1364/PRJ.394726
35. Chen C, Wei F, Han X, Su Q, Pi H, Xin G, et al. Hybrid integrated Si3N4 external cavity laser with high power and narrow linewidth. Opt Express (2023) 31(16):26078–91. doi:10.1364/OE.487850
36. Guo H, Olamax G. Analysis of no mode-hop tuning of mirror-grating external-cavity diode laser. Opt Commun (2018) 421:90–3. doi:10.1016/j.optcom.2018.03.074
37. Fedorova K, Cataluna M, Kudryashov I, Khalfin V, Rafailov E. Broadly tunable InGaAsP-InP strained multi-quantum-well external cavity diode laser. IEEE Photonic Technol Lett (2010) 22(413):1205–7. doi:10.1109/LPT.2010.2051661
38. Jin J, Jiao Q, Li Y, Lv F, Zhang G. Study on external-cavity semiconductor laser. Chin Opt Lett (2003) 1(3):147–8.
Keywords: wavelength-tunable laser, semiconductor laser, mode-hopping free, external-cavity feedback, narrow linewidth
Citation: Sheng L, Liu Z, Wang J, Liu L, Yin B, Zhang Z, Zhou S and Huang L (2025) Mode-hop-free wavelength-tunable semiconductor lasers covering the S+C+L band via external-cavity optical feedback. Front. Phys. 13:1586316. doi: 10.3389/fphy.2025.1586316
Received: 02 March 2025; Accepted: 15 April 2025;
Published: 09 May 2025.
Edited by:
Tonglei Cheng, Northeastern University, ChinaReviewed by:
Xiaobo Hu, Zhejiang Sci-Tech University, ChinaG. Palai, Sri Sri University, India
Tianli Feng, Shandong University, China
Copyright © 2025 Sheng, Liu, Wang, Liu, Yin, Zhang, Zhou and Huang. This is an open-access article distributed under the terms of the Creative Commons Attribution License (CC BY). The use, distribution or reproduction in other forums is permitted, provided the original author(s) and the copyright owner(s) are credited and that the original publication in this journal is cited, in accordance with accepted academic practice. No use, distribution or reproduction is permitted which does not comply with these terms.
*Correspondence: Lin Huang, aHVhbmdsaW5AY2V5ZWFyLmNvbQ==; Zhiming Liu, bGl1emhpbWluZ0BjZXllYXIuY29t